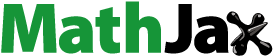
Abstract
Nanogels exhibit potential application values in drug delivery systems because of their tunable properties and biocompatibility. In this study, multifunctional hyaluronic-based nanogels (HNPs), all-trans retinoid acid (ATRA)/aggregation-induced emission luminogen (AIEgen) fluorophores (TPENH2)-grafted hyaluronic acid (HA) with disulfide bonds as linkers of (HA-ss-ATRA/TPENH2), were successfully developed for doxorubicin hydrochloride (DOX) delivery. Besides, the controls of HA-ATRA/TPENH2 were also developed for comparison. The HNPs with nanoscale particle sizes possessed an excellent DOX-loading capacity. As expected, the DOX-loaded HNPs exhibited the higher stability in a normal physiological environment (10 µM GSH), but rapidly disintegrated in the cancer microenvironment (20 mM GSH) according to an in vitro drug release study. The intracellular observation of HNPs by the fluorescence microscopy indicated that the self-fluorescent DOX-loaded HNPs with unique AIEgen characteristic transported DOX into the cancer cells and visibly accumulated within the cytoplasm. Importantly, the accumulation of DOX-loaded HNPs was largely increased due to the targeted reorganization of CD44 or LYCE-1 receptors by HA moieties on the surface of HNPs. Based on in vitro cytotoxicity analyses, the DOX-loaded HNPs displayed dramatically enhanced virulence to cancer cells compared to the controls and free DOX. This is the first study to achieve combined functionalities of targeted delivery, controllable release and real-time intracellular imaging on HA-base nanogels with enhanced antitumor efficiency. Therefore, the multifunctional HA-ss-ATRA/TPENH2 HNPs have good potential to develop a novel drug delivery platform for the targeted delivery and controlled release of DOX to achieve enhanced antitumor efficiency as well as real-time intracellular imaging.
Introduction
Cancer is a highly fatal disease which involves abnormal cell growth with the opportunity to move to and invade other parts of the body [Citation1–3]. To date, chemotherapy has still remained the most commonly used therapeutic option for cancer treatment [Citation4–6]. As a widely used chemotherapeutical drug, doxorubicin hydrochloride (DOX) can effectively inhibit the proliferation of cancer cells through DNA damage inside the nucleus [Citation7,Citation8]. Nevertheless, several drawbacks such as drug resistance, poor tumour accumulation and cardiotoxicity to humans often occur during DOX utilization [Citation9–11]. To combat some of these issues, multifunctional nanomaterials which can effectively deliver therapeutic agents to the site of cancer have been explored for the mediation of DOX delivery [Citation12–14]. In the past decade, various nanomaterials ranging from liposomes to emulsions have been used for the delivery of DOX [Citation15,Citation16]. Among these, nanoparticles based on “green” synthetic methodologies without the use of toxic solvents were highly desirable in drug delivery systems [Citation17]. Nanogels, as a promising kind of nanomaterials, have emerged rapidly due to their easy fabrication in an all-aqueous environment [Citation18]. Moreover, nanogels with hydrophilic polymeric networks possess many other preferential properties including high water content and low surface tension, which contribute to their biocompatibility and high drug-loaded efficiency in drug delivery [Citation19,Citation20].
However, conventional nanogels used to deliver drugs face a large problem of off-target effects, which cause serious toxic adverse effects to normal cells [Citation21,Citation22]. Hyaluronic acid (HA), a biocompatible polysaccharide with excellent ability to bind to the CD44 or LYCE-1 receptors overexpressed on the surface of a variety of tumor cells, has been used as a targeting moiety of the drug conjugates or nanomaterials to effectively enhance the cellular uptake and internalization of nanomaterials in tumor therapy [Citation23–25]. Moreover, HA is a suitable alternative to polyethylene glycol (PEG), with its ability not only to provide high-affinity binding to tumour sites but also to promote drug circulation [Citation26]. HA-modification endows the nanogels excellent biocompatibility, targeted therapy and long-circulation during utilization. Hence, it is important to develop HA-based nanogels as a new platform to achieve increased intracellular DOX concentrations for tumour-targeted delivery.
The other major issue is that many conventional nanogels have low drug concentrations released at target sites, causing decreased anti-cancer efficacy [Citation27–29]. To tackle this problem, naturally occurring bio-signals have been exploited for the development of stimuli-sensitive systems to tumour cells [Citation30]. It is known that cancer cells maintain more than 3-times higher levels of glutathione (GSH) in the cytoplasm and nucleus, causing a greater reduction than in those of normal cells [Citation31,Citation32]. The prominent redox variation between the intracellular environment of the tumour and normal cells endow redox-responsive drug-loaded nanomaterials with enhanced anti-cancer effects and subdued side effects by releasing substances only in the cancer cells instead of in normal cells [Citation33,Citation34]. Among the redox-responsive drug delivery systems, disulfide bonds are the most generally used functional groups to fabricate nanogels to achieve the controlled release of drugs in cancer cells because the disruption of polymeric networks of nanogels caused by thiol-disulfide breakage easily occurs in high intracellular GSH environments [Citation35]. Great effects have been achieved by the modification of nanogels by characteristic disulfide linkages to promote drug release functions.
One of the most urgent tasks in cancer treatment is exploring a simple and fast method to directly visualize the accurate drug localization to study the theranostic pharmacokinetics of them within complex and dynamic environments [Citation36,Citation37]. According to some recent studies, researchers functionalize nanomaterials with detectable groups, which can provide information including the cellular internalization pathway and intracellular biodistribution of drugs by testing them with corresponding imaging techniques, such as magnetic resonance imaging [Citation38], positron emission tomography [Citation39], computed tomography [Citation40] and fluorescence-based optical imaging. Among these techniques, fluorescence-based optical imaging is the universally used method focused on microlevel evaluations and may provide more general information about the transportation and distribution of nanoparticles in the entire organism [Citation41]. However, conventional organic dyes for optical imaging suffer from interference caused by photobleaching of the background and the aggregation-caused quenching (ACQ) effect [Citation42]. Especially for labelled nanoparticles which could aggregate by themselves, this photobleaching and the ACQ effect are particularly serious. Aggregation-induced emission luminogen (AIEgen), a novel kind of luminophores, works perfectly at high concentrations as well as in the aggregate state, with strong emission and a high photobleaching threshold [Citation43,Citation44]. The unique advantage endows us with great enthusiasm to develop versatile AIEgen-based nanogel theranostic systems, which will open up new avenues for ACQ-free and self-luminance drug delivery systems to achieve optimizing real-time optical imaging in cancer treatment.
In this study, we particularly aimed to develop multifunctional amphiphilic HNPs for the targeted delivery and controlled release of DOX, achieving enhanced antitumor efficiency and real-time intracellular imaging. HA served not only as a targeting agent but also as a hydrophilic agent of HNPs. We introduced All-trans retinoic acid (ATRA) as a hydrophobic nucleus of HNPs. Disulfide bonds were used as redox-sensitive linkages between HA and ATRA to develop HA-ss-ATRA amphiphilic conjugates. Thereafter, the AIE fluorophores (TPENH2), which have terminal amine groups, were modified with HA-ss-ATRA conjugates to develop HA-ss-ATRA/TPENH2 conjugates. Finally, doxorubicin (DOX) hydrochloride, as the main therapeutic agent, was incorporated into the HNPs network to develop DOX-loaded HNPs. The multifunctional DOX-loaded HNPs maintained stabilization circulating in the bloodstream but released drugs in a reducing environment when selectively taken up by cancer cells via HA-receptor-mediated action (as shown in Scheme 1), and generated enhanced cytotoxicity compared with the free DOX and HA-ATRA/TPENH2 HNPs. Furthermore, HNPs with unique AIE characteristics successfully achieved real-time intracellular imaging within dynamic environments. We expect that these multifunctional HNPs will inspire the development of new targeted and redox-responsive nanocarriers with real-time imaging and efficient therapeutic goals for cancer therapy.
Scheme 1. Synthesis of HA-ss-ATRA/TPENH2 conjugates (A). Illustration of the self-assembly, accumulation at cancer cells, intracellular trafficking pathway of redox-sensitive AIE HNPs, and real-time intracellular imaging of AIE HNPs (B). Circulation of AIE HNPs within vessel (a); Accumulation of DOX-loaded HNPs within the tumor site through passive and active targeting effects (b); CD44 receptor-mediated cellular internalization (c, d); Endo/Lysosomal escape, and GSH-controllable release of DOX in cytoplasm (e, f); Released DOX into nucleus for apoptosis and cytotoxicity (g).
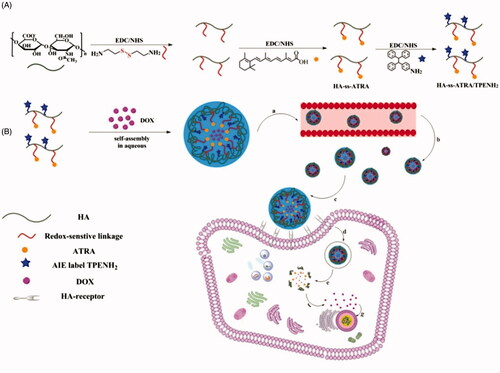
Experimental
Materials and reagents
Sodium hyaluronic acid (molecular weight 7 kDa) was purchased from Freda Biochem Co., Ltd. (Shandong, China). 1-Ethyl-3 (3-dimethylaminopropyl) carbodiimide (EDC), N-hydroxysuccinimide (NHS), tretinoin and glutathione were purchased from Energy Chemical Co., Ltd. (Shanghai, China). Doxorubicin (DOX) hydrochloride was purchased from Sinopharm Chemical Reagent Co., Ltd. (Shanghai, China). Cystamine dihydrochloride and ethylenediamine were purchased from Aladdin Reagent Database Inc. (Shanghai, China) and Macklin Biochemical Co., Ltd. (Shanghai China), respectively. Benzophenone and 4-aminobenzophenone were purchased from TCI Development Co., Ltd. (Shanghai, China). All other chemicals were of analytical grade and were used without further purification.
Synthesis of cystamine-modified HA and ethylenediamine modified HA
Cystamine-modified HA was prepared as previously reported [Citation45] with modification. Briefly, HA was dissolved in PBS solution with EDC and NHS and then stirred for 15 min to activate the carboxyl groups. The ratio of HA, NHS and EDC was 1:1.2:1.2. Then cystamine dihydrochloride was added. The mixture was stirred for 4 h at room temperature. The resulting solution was dialyzed (MWCO 3500) against 0.1 M NaCl and distilled water. Finally, the polymer solution was lyophilized and stored at 4 °C until further use.
Ethylenediamine-modified HA was synthesised by a similar method. Firstly, HA was dissolved in water with a certain ratio of EDC and NHS to activate the carboxyl groups of HA. Then, ethylenediamine was added with 4 h stirring at pH 4.75 and room temperature. Finally, the reaction was quenched by adding 0.1 M NaOH to adjust the pH of the reaction mixture to 7.0. The resulting solution was dialyzed (MWCO 3500) against distilled water for 48 h. The polymer solution was lyophilized and stored at 4 °C until further use.
Synthesis of HA-ss-ATRA conjugates and HA-ATRA conjugates
HA-ss-ATRA conjugates were prepared by reacting the carboxyl group of ATRA with the amino group of cystamine-modified HA. HA-ATRA conjugates were prepared by the same method. In brief, ATRA was dissolved in DMF with equimolar amounts of EDC and NHS at 0 °C for 6 h and at 20 °C overnight to activate the carboxyl group of ATRA. Cystamine-modified HA or ethylenediamine-modified HA was dissolved in formamide, then activated ATRA was added to the solution under gentle stirring for 24 h at room temperature, at a molar ratio of activated ATRA to HA of 0.5:1. The resulting solution was dialyzed against distilled water for two days. Finally, the solution was lyophilized, and the product (a yellow suspension) stored at 4 °C for further use.
Synthesis of AIE molecule TPENH2
AIE molecule TPENH2 was prepared according to previous reports [Citation46]. In brief, a certain amount of zinc powder was added to a 100 ml round-bottom flask containing 40 ml THF. The mixture was agitated in an ice bath until the temperature dropped to 0 °C. Then, titanium tetrachloride was slowly added to the reactant mixture, maintaining the temperature below 1 °C. The resulting solutions warmed to room temperature and started reflux condensation for 25 h. The mixture was cooled to 0 °C, pyridine added and stirred for 10 min. Benzophenone and 4-aminobenzophenone dissolved in 15 ml THF was slowly added to the above solution for 36 h reflux condensation. Finally, the reaction was quenched by adding 10% K2CO3. The crude extracts were obtained by extraction and TLC, and the final products were obtained as white powders by liquid chromatography (HPLC, Waters 1525) with a C18 column (5 μm particle size, 250 mm ×4.6 mm). The mobile phase consisted of methanol and water (71:29, v/v) with a flow rate of 1.0 ml/min. The detection wavelength was 254 nm and sample injected volume was 15 μl.
Preparation and characterization of HA-ss-ATRA/TPENH2 conjugates and HA-ATRA/TPENH2 conjugates
Amphiphilic HA-ss-ATRA/TPENH2 conjugates and HA-ATRA/TPENH2 conjugates were prepared by chemically grafting TPENH2 to HA-ss-ATRA and HA-ATRA conjugates, respectively. Briefly, HA-ss-ATRA was dissolved in formamide with EDC and NHS, and the mixture stirred for 10 min. The ratio of HA-ss-ATRA, NHS and EDC was 1:0.5:0.5. Then, TPENH2 dissolved in DMF was added to the above solution for 24 h with stirring at room temperature. The reactant solution was dialyzed against distilled water for 48 h. Finally, the resulting solution was filtered to remove unreacted TPENH2, the filtrate lyophilized, then stored at 4 °C until further use. HA-ATRA/TPENH2 conjugates were synthesised by the same procedure. Final products were analyzed by UV spectrophotometry (UV-1750, Shimadzu, Japan) and fluorescence spectrophotometry (RF-5301Pc, Shimadzu, Japan).
Preparation and characterization of AIE nanoparticles
The HA-ss-ATRA/TPENH2 nanoparticles were prepared by an ultrasonic method. Briefly, HA-ss-ATRA/TPENH2 conjugates were completely dissolved in distilled water and the solution was sonicated (KQ3200DE ultrasonicator, Jiangsu, China) for 30 min at 100 W in an ice bath. HA-ATRA/TPENH2 nanoparticles were manufactured in the same way. The morphology and size distribution were observed by scanning electron microscope (SEM, S-4800, Hitachi, Japan) and transmission electron microscope (TEM, HT 7700, Hitachi, Japan). The particle size was measured by dynamic light scattering (DLS, JY 92–2 D, Ningbo, China). The measurement conditions of the samples were 25 °C and 90° scattering angle.
Preparation of DOX-loaded AIE nanoparticles
To prepare DOX-loaded HA-ss-ATRA/TPENH2 nanoparticles, in brief, the HA-ss-ATRA/TPENH2 conjugates were dissolved in water under stirring for 10 min, and DOX dissolved in distilled water was added to the solution. The mixture was sonicated for 30 min at 100 W in an ice bath. Then, the solution was dialyzed (MWCO 3500) against distilled water for 48 h, and the final solution lyophilized. UV spectrophotometry was used to measure the concentrations of DOX. The drug-loading (DL) was calculated by the following equation:
In vitro release of drug from AIE nanoparticles triggered by glutathione
A dialysis method was used to obtain the dissolution curve by measuring the concentrations of DOX released from HA-ss-ATRA/TPENH2 and HA-ATRA/TPENH2 nanoparticles. DOX-loaded HA-ss-ATRA/TPENH2 and HA-ATRA/TPENH2 nanoparticles were dissolved in PBS (pH 7.4, 0.15 M) and placed in a dialysis bag (MWCO 3500) which existed two concentrations of GSH (10 μM and 20 mM), and the system stirred at 100 rpm at 37 °C in a water bath. At a series of planned time intervals, 3 ml dialysis fluid was taken out to detect the concentrations of DOX, measured by ultraviolet spectrophotometry.
In vitro cytotoxicity studies
The in vitro cytotoxicity of DOX-loaded nanoparticles and free DOX were assessed by means of MTT assay. The HepG-2 and 293 T cells were incubated in 96-well plates with cell density 7 × 103 cells/well in cell culture media (1640 + 10% FBS) in a humid environment with 5% CO2 at 37 °C for 24 h. The DOX-loaded nanoparticles with equivalent DOX concentrations, free DOX and blank nanoparticles solutions were treated with cells for 24 h, 48 h and 72 h. After incubating, to each well was added 10 μl MTT solutions (5 mg/ml in PBS) and the cells incubated for 4 h. Finally, the crystals were dissolved by DMSO after removing the media. The OD was measured with a microplate reader. Cell viability (%) was calculated as [(OD of test group – OD of blank group)/(OD of control group – OD of blank group)] × 100.
In vitro imaging of AIE nanoparticles
Fluorescence microscopy was used to observe the intracellular uptake and distribution of HA-ss-ATRA/TPENH2 nanoparticles. The HepG-2 cells were incubated in 6-well plates with a cell density of 3 × 105 cells/well with 5% CO2 at 37 °C for 24 h. After incubation, the cells were treated with HA-ss-ATRA/TPENH2 for 4 h, 6 h, 8 h and 10 h. Finally, cells were washed with PBS (pH 7.4) five times and observed by fluorescence microscopy.
Results and discussion
Characterization of HA-ss-ATRA conjugates and HA-ATRA conjugates
For the purpose of preparing the HA-ss-ATRA conjugates, cystamine dihydrochloride was used to concatenate HA polymers with retinoid acid through the terminal amine groups, as shown in Scheme 1. Following this method, some of the carboxyl groups of HA were modified with cystamine after activating with EDC and NHS. Then the exposed free amine reacted with retinoid acid by amine-reactive coupling. HA-ATRA conjugates were synthesised by uniform reaction mechanism but used ethylenediamine as the linker between HA and retinoid acid.
The chemical compositions of synthesised conjugates were verified by 1H NMR (as shown in . The methyl peak of the acetamide group of HA appeared at 2.0 ppm (–NCOCH3); other characteristic peaks of HA appeared at 3.37–4.60 ppm, and two new amide linkages between HA and ATRA appeared at 8.01 ppm and 8.29 ppm, respectively. The methylene in cystamine appeared at 2.83 ppm (–CH2CH2NH2–). The characteristic peaks of ATRA appeared at 1.0–1.5 ppm. The results indicated that ATRA was successfully grafted on to the HA chain.
Characterization of AIE molecule TPENH2
Scheme 2 illustrates the synthesis process of the TPENH2 compound. Briefly, products were obtained from a cross McMurry reaction between benzophenone and 4-aminobenzophenone, separating and then purifying the product by silica column chromatography and high-performance liquid chromatography (HPLC). The peak area of TPENH2 reached 94.18% from HPLC analysis (see the Supporting Information for details). The chemical structure has been verified by 1H NMR (see the Supporting Information for details). 1H NMR (500 MHz, DMSO) δ 7.51–7.42 (m, J = 14.7, 7.6 Hz, 4H), 7.37 (d, J = 7.4 Hz, 2H), 7.16–6.91 (m, 3H), 6.33 (m, J = 31.9, 8.2 Hz, 2H), 5.63 (d, J = 19.6 Hz, 1H), 5.03 (s, 1H).
Characterization of HA-ss-ATRA/TPENH2 and HA-ATRA/TPENH2 conjugates
The AIE molecules were modified with HA-ss-ATRA or HA-ATRA conjugates by amine-reactive coupling between TPENH2 and those HA carboxyl groups that were not reacted in the previous step. The UV absorbance of HA-ss-ATRA/TPENH2 conjugates was tested by ultraviolet spectrophotometry, as shown in . From 250 to 700 nm wavelengths, the HA-ss-ATRA/TPENH2 conjugates appeared as absorption peaks at 290 and 370 nm. The HA-ss-ATRA conjugates and TPENH2 appeared as absorption peaks at 290 and 350 nm, respectively. It can be seen that the characteristic peaks of HA-ss-ATRA/TPENH2 conjugates coincided with HA-ss-ATRA conjugates and TPENH2 respectively. The result confirmed that the HA-ss-ATRA conjugates were obviously embellished with AIE molecules. The fluorescence spectra of TPENH2, HA-ss-ATRA conjugates, and HA-ss-ATRA/TPENH2 conjugates were also determined. HA-ss-ATRA/TPENH2 conjugates displayed distinct fluorescent signals at 397 nm (see the Supporting Information for details).
Preparation and characterisation of AIE HNPs
The redox-sensitive HA-ss-ATRA/TPENH2 HNPs and non-redox-sensitive HA-ATRA/TPENH2 HNPs were prepared by a simple sonication method in aqueous conditions. The synthesis of HNPs was performed in an all-aqueous environment, which meets the requirement of “green” synthetic methodologies without the use of toxic solvents. Dynamic light scattering (DLS) measurement indicated that the particle sizes of HA-ss-ATRA/TPENH2 HNPs ranged from 410 to 830 nm (), but about 60% of nanoparticle sizes were in the range of 585 to 699 nm. The emblematic SEM and TEM images of HA-ss-ATRA/TPENH2 HNPs showed that the nanoparticles were of almost spherical shape with satisfactory dispersibility (). The size estimated by SEM was a little smaller than by DLS because of different sample inspection conditions. Dissolution in aqueous solution during DLS sample preparation caused swelling of hydrophilic nanoparticles.
In vitro drug release triggered by glutathione
The effect of disulfide bonds on the drug release behaviour of the nanoparticles was evaluated at 37 °C in the presence and absence of GSH. Nanoparticles that showed a slow release of DOX from HA-ss-ATRA/TPENH2 HNPs were observed in the presence of 10 µM GSH. As shown in , just 4.6% of DOX was released in the first 4 h and roughly 39.9% released within 26 h. Similarly, the group of HA-ATRA/TPENH2 nanoparticles with 20 mM GSH also revealed an inefficient release of DOX. For example, about 7.68% of DOX was released after 4 h and approximately 43.01% released within 26 h. In contrast, the release of DOX from HA-ss-ATRA/TPENH2 HNPs was significantly accelerated in the presence of 10 mM GSH. A great deal of DOX was released in just 5 h and about 73.73% released after 26 h. Moreover, the release of DOX from HNPs was also significantly accelerated in the presence of 20 mM GSH. About 93.7% DOX was released after 26 h. Our results indicate that the redox-sensitive HA-ss-ATRA/TPENH2 HNPs may be stable without releasing the anticancer drugs circulating within blood vessels but released internally by cancer cells.
In vitro cytotoxicity studies
According to the site-specific delivery of DOX by nanoparticles, the in vitro anticancer efficacy of DOX-loaded HA-ss-ATRA/TPENH2 HNPs, DOX-loaded HA-ATRA/TPENH2 HNPs and free DOX were investigated on HepG-2 (CD44 overexpression) and 293 T cells. Cell viability was evaluated after incubation with several concentrations of free DOX, and sensitive and insensitive DOX-loaded HNPs containing a corresponding DOX content, for 24 h, 48 h and 72 h. After 24 h incubation, a comparison was made between DOX-loaded HA-ss-ATRA/TPENH2 and free DOX at an equivalent concentration gradient. shows the trend of cytotoxicity increasing with increase in drug concentration for all formulations. The difference was the cytotoxicity of DOX-loaded HA-ss-ATRA/TPENH2 HNPs being inferior to that of free DOX at equal concentrations. This may be attributed to the different intracellular uptake rate of free DOX (transported into cells and diffused into cytosol by a passive diffusion mechanism) and nanoparticles (internalized by endocytosis). But inversely, after 48 h and 72 h incubation at 10 μg/ml concentration of DOX, the cytotoxicity of DOX-loaded HA-ss-ATRA/TPENH2 HNPs in showed an obvious rise compared to free DOX, which may based on the fact that greater amounts of DOX would accumulate in the cancer cells after encapsulation by nanoparticles than from free DOX. The result was also verified through further imaging analysis by fluorescence microscopy. Moreover, after 48 h incubation, as shown in , DOX-loaded HA-ss-ATRA/TPENH2 HNPs emerged with a more preferable anticancer effect and enhanced cytotoxicity than DOX-loaded HA-ATRA/TPENH2 HNPs, which could be attributed to the high concentrations of GSH and the corresponding reductases in the intracellular environment of HepG-2 cells. As shown in , the statistical analysis of the cell viability data was also provided to support that DOX-loaded HNPs displayed an enhanced antitumor efficiency than free DOX. Experiments proved that more potentials from sensitive nanoparticles than insensitive nanoparticles for intracellular delivery of DOX. Furthermore, after 24 h incubation, shows blank HA-ss-ATRA/TPENH2 HNPs displaying high cell viability and no marked cytotoxicity against 293 T cells and HepG-2 cells, indicating its good biocompatibility characteristics. Importantly, the DOX-loaded HNPs displayed dramatically enhanced virulence to cancer cells compared to the controls and free DOX. Synthetically, redox-sensitive HA-ss-ATRA/TPENH2 HNPs have a great potential in in vitro cancer therapy, and are fairly safe as anticancer drug delivery systems for clinical treatment.
Figure 5. Cell viability of HepG-2 cells treated with DOX-loaded HA-ss-ATRA/TPENH2 HNPs and free DOX after 24 h (A). Cell viability of HepG-2 cells treated with DOX-loaded HA-ss-ATRA/TPENH2 HNPs and free DOX after 24 h, 48 h and 72 h (B). Cytotoxicity of blank HA-ss-ATRA/TPENH2 HNPs against HepG-2 cells and 293T cells (C). Cell viability of HepG-2 cells treated with DOX-loaded HA-ss-ATRA/TPENH2 HNPs and DOX-loaded HA-ATRA/TPENH2 HNPs (D). Cell survival fractions were assessed by MTT assay. Data represent mean ± SD (n = 5).
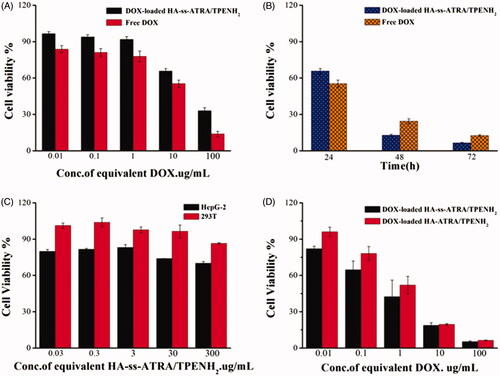
Table 1. IC50 of Free DOX and DOX-loaded HNPs for HepG-2 cells.
As shown in , the trend of cytotoxicity was increasing with an increase in drug concentration for all formulations. The cytotoxicity of DOX-loaded HA-ss-ATRA/TPENH2 HNPs for 293 T cells was inferior to that of HepG-2 cella at equal concentrations after incubating with HepG-2 cells and 293 T cells. Due to the presence of the disulfide bond in HA-ss-ATRA/TPENH2 HNPs, DOX-loaded HA-ss-ATRA/TPENH2 HNPs can rapidly cleave and release the drug in the presence of a high concentration of GSH in HepG-2 cells to achieve the purpose of killing the cancer cells. However, DOX-loaded HA-ss-ATRA/TPENH2 HNPs could not be rapidly cleaved and release the drug in the normal intracellular environment of 293 T cells, thus showing less obvious cytotoxicity. The result proved that DOX-loaded HNPs could not be harmful for 293 T cells.
Figure 6. Cell viability of HepG-2 and 293T cells treated with DOX-loaded HA-ss-ATRA/TPENH2 HNPs after 48 h.
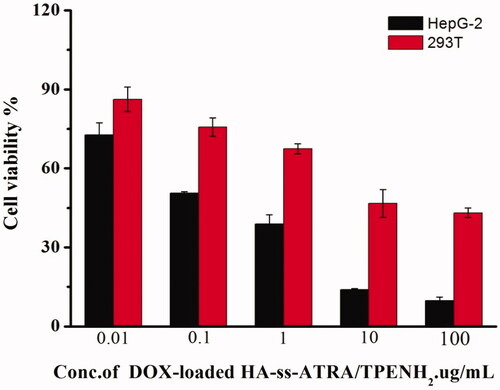
In the present study, we mainly focused on multifunctional drug delivery system for in vitro use, and we think that in vitro experiments may not be optimal, but should be sufficient to draw a conclusion that the functionalized nanoparticles has potential applications on delivering DOX in cancer therapy, in vivo animal experiments are ongoing and will continue in future work.
In vitro imaging analysis
To monitor the in vitro distribution and cellular uptake of the nanoparticles, 10 μg/ml blank HA-ss-ATRA/TPENH2 HNPs, free DOX and DOX-loaded HA-ss-ATRA/TPENH2 HNPs were incubated with HepG-2 cells for 8 h. TPENH2 with AIE characteristic as a probe endowed nanoparticles unique fluorescence. As shown in , blue fluorescence was clearly observed and distributed intensively in the cytoplasm after incubating with blank HA-ss-ATRA/TPENH2 HNPs, due to the overexpressed receptors from HepG-2 cells. However, the blue fluorescence areas were not observed in the nucleus, suggesting that the nanoparticles cannot penetrate the nuclear membrane. This demonstrated that the nanoparticles were internalized by cells and readily centralized in the endosome. Furthermore, after 8 h incubation with free DOX, showed that red fluorescence was distributed evenly within the cytoplasm and nucleus. In contrast, shows that red fluorescence was distributed homogeneously in the cytoplasm and more deeply than when incubated with DOX-loaded HA-ss-ATRA/TPENH2 HNPs. Importantly, the fluorescence areas where the red fluorescence was concentrated were consistent with the area where the blue fluorescence was concentrated, which intuitively demonstrated that DOX-loaded HNPs would accumulate more DOX in cancer cells to enhance the anticancer effect compared to free DOX. Synthetically, the results revealed that the HA-ss-ATRA/TPENH2 HNPs as anticancer drugs delivery systems have potential biological imaging applications.
Figure 7. Fluorescence microscopy images of HepG-2 cells incubated with HA-ss-ATRA/TPENH2 HNPs (A), free DOX (B) and DOX-loaded HA-ss-ATRA/TPENH2 HNPs (C) for 8 h. For each panel, left to right show the UW, GW, bright and merge field images. Scale bars correspond to 10 μm in all the images.
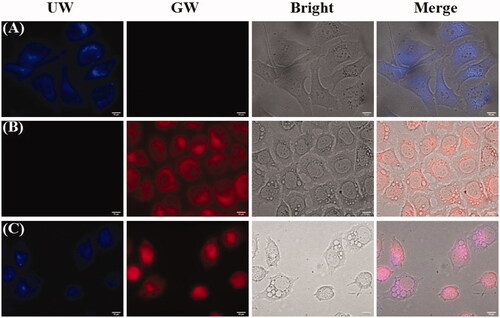
To prove performance on the time-dependent cell imaging of nanoparticles, we incubated HepG-2 cells with HA-ss-ATRA/TPENH2 nanoparticles for 12 h, and traced them at 4 h, 6 h, 8 h and 10 h by a fluorescence microscopy. When incubated for 4 h, as shown in , the nanoparticles were internalized by endocytosis and were transported within the cytoplasm. The majority of nanoparticles were present in the cytoplasm instead of at sites near the nucleus. When incubated for 6 h, as shown in , almost all nanoparticles accumulated near but did not enter, the nucleus. When incubated for 8 h, blue fluorescence (as shown in ) near the nucleus caused the quenching. This phenomenon revealed that nanoparticles were disintegrating and releasing DOX in the high-GSH-concentration environment of the cancer cell. After incubating for 10 h, showed just a small amount of blue fluorescence sited near the nucleus, and the fluorescence intensity of the nanoparticles was weaker compared to . This is due to most of the nanoparticles having disintegrated and completed their mission of drug delivery.
Conclusions
In conclusion, we designed and applied multifunctional HA-ss-ATRA/TPENH2 HNPs as novel nanocarriers to realize the targeted delivery and controlled release of DOX with enhanced antitumor efficiency and real-time intracellular imaging. The HNPs which can be self-assembled easily in an all-aqueous environment are comparatively stable and less toxic. Through HA receptor-mediated action, as well as EPR effects, HNPs transport DOX, targeting the cancer cells over normal cells. According to an in vitro drug release study, DOX-loaded HNPs showed a significantly accelerated release in the presence of 20 mM GSH compared with 10 µM GSH. As shown by in vitro cytotoxicity studies, the DOX-loaded HNPs displayed dramatically enhanced virulence to cancer cells over the DOX-loaded HA-ATRA/TPENH2 HNPs and free DOX. Moreover, real-time function bioimaging, which overcomes photobleaching of the background and the ACQ effect, was successfully used to accurately locate and trace HNPs within dynamic environments under a fluorescence microscope. We believe that this multifunctional nanogels drug delivery system will offer potential value for the development of new targeted and stimuli-responsive drug nanocarriers with unique AIE characteristics for efficient cancer therapy.
Disclosure statement
No potential conflict of interest was reported by the authors.
Additional information
Funding
References
- Jin J, Guo M, Liu J. Graphdiyne nanosheet-based drug delivery platform for photothermal/chemotherapy combination treatment of cancer. ACS Appl Mater Interfaces. 2018;10:8436–8442.
- Gu X, Kwok RTK, Lam JWY, et al. AIEgens for biological process monitoring and disease theranostics. Biomaterials. 2017;146:115–135.
- Hanahan D, Weinberg RA. The hallmarks of cancer. Cell. 2000;100:57–70.
- Mrówczyński R. Polydopamine-based multifunctional (nano)materials for cancer therapy. ACS Appl Mater Interfaces. 2018;10:7541–7561.
- Qi Y, Min H, Mujeeb A, et al. Injectable hexapeptide hydrogel for localized chemotherapy prevents breast cancer recurrence. ACS Appl Mater Interfaces. 2018;10:6972–6981.
- Chen Q, Meng F, Wang L, et al. A polymorphism in ABCC4 is related to efficacy of 5-FU/capecitabine-based chemotherapy in colorectal cancer patients. Sci Rep. 2017;7:7059.
- Zhou Z, Jafari M, Sriram V, et al. Delayed sequential co-delivery of gefitinib and doxorubicin for targeted combination chemotherapy. Mol Pharm. 2017;14:4551–4559.
- Abe J, Yamada Y, Takeda A, et al. Cardiac progenitor cells activated by mitochondrial delivery of resveratrol enhance the survival of a doxorubicin-induced cardiomyopathy mouse model via the mitochondrial activation of a damaged myocardium. J Control Release. 2018;269:177–188.
- Song YF, Liu DZ, Cheng Y, et al. Charge reversible and mitochondria/nucleus dual target lipid hybrid nanoparticles to enhance antitumor activity of doxorubicin. Mol Pharm. 2018;15:1296–1308.
- Zhai Q, Chen Y, Xu J, et al. Lymphoma immunochemotherapy: targeted delivery of doxorubicin via a dual functional nanocarrier. Mol Pharm. 2017;14:3888–3895.
- Tang J, Zhang R, Guo M, et al. Nucleosome-inspired nanocarrier obtains encapsulation efficiency enhancement and side effects reduction in chemotherapy by using fullerenol assembled with doxorubicin. Biomaterials. 2018;167:205.
- Song W, Musetti SN, Huang L. Nanomaterials for cancer immunotherapy. Biomaterials. 2017;148:16–30.
- Overchuk M, Zheng G. Overcoming obstacles in the tumor microenvironment: Recent advancements in nanoparticle delivery for cancer theranostics. Biomaterials. 2018;156:217–237.
- Chen Y, Su M, Li Y, et al. Enzymatic PEG-poly(amine-co-disulfide ester) nanoparticles as pH- and redox-responsive drug nanocarriers for efficient antitumor treatment. ACS Appl Mater Interfaces. 2017;9:30519–30535.
- Luo D, Goel S, Liu HJ, et al. Intrabilayer (64)Cu labeling of photoactivatable, doxorubicin-loaded stealth liposomes. ACS Nano. 2017;11:12482–12491.
- Zhao CX. Multiphase flow microfluidics for the production of single or multiple emulsions for drug delivery. Adv Drug Deliv Rev. 2013;65:1420–1446.
- Lu Y, Ozcan S. Green nanomaterials: On track for a sustainable future. Nano Today. 2015;10:417–420.
- Lai WF, He ZD. Design and fabrication of hydrogel-based nanoparticulate systems for in vivo drug delivery. J Control Release. 2016;243:269–282.
- Raemdonck K, Demeester J, Smedt SD. Advanced nanogel engineering for drug delivery. Soft Matter. 2009;5:707–715.
- Lai WF, Shum HC. Hypromellose-graft-chitosan and its polyelectrolyte complex as novel systems for sustained drug delivery. ACS Appl Mater Interfaces. 2015;7:10501.
- Siangsanoh C, Ummartyotin S, Sathirakul K, et al. Fabrication and characterization of triple-responsive composite hydrogel for targeted and controlled drug delivery system. J Mol Liq. 2018;256:90–99.
- Wang C, Zhang G, Liu G, et al. Photo- and thermo-responsive multicompartment hydrogels for synergistic delivery of gemcitabine and doxorubicin. J Control Release. 2017;259:149–159.
- Dong X, Zou S, Guo C, et al. Multifunctional redox-responsive and CD44 receptor targeting polymer-drug nanomedicine based curcumin and alendronate: synthesis, characterization and in vitro evaluation. Artif Cells Nanomed Biotechnol. 2017;1:1–10.
- Xu W, Qian J, Hou G, et al. Hyaluronic acid-functionalized gold nanorods with pH/NIR dual-responsive drug release for synergetic targeted photothermal chemotherapy of breast cancer. ACS Appl Mater Interfaces. 2017;9:36533–36547.
- Chi Y, Yin X, Sun K, et al. Redox-sensitive and hyaluronic acid functionalized liposomes for cytoplasmic drug delivery to osteosarcoma in animal models. J Control Release. 2017;261:113–125.
- Ravar F, Saadat E, Gholami M, et al. Hyaluronic acid-coated liposomes for targeted delivery of paclitaxel, in-vitro characterization and in-vivo evaluation. J Control Release. 2016;229:10–22.
- Santos TCd, Hernández R, Rescignano N, et al. Nanocomposite chitosan hydrogels based on PLGA nanoparticles as potential biomedical materials. Eur Polym J. 2018;99:456–463.
- Naahidi S, Jafari M, Logan M, et al. Biocompatibility of hydrogel-based scaffolds for tissue engineering applications. Biotechnol Adv. 2017;35:530–544.
- Wang HQ, Tan H, Hua S, et al. High efficiency conversion of regenerated cellulose hydrogel directly to functionalized cellulose nanoparticles. Macromol Rapid Commun. 2017;38:1700409.
- Ahmadi Nasab N, Hassani Kumleh H, Beygzadeh M, et al. Delivery of curcumin by a pH-responsive chitosan mesoporous silica nanoparticles for cancer treatment. Artif Cells Nanomed Biotechnol. 2018;46:75–81.
- Zhao J, Yang Y, Han X, et al. Redox-sensitive nanoscale coordination polymers for drug delivery and cancer theranostics. ACS Appl Mater Interfaces. 2017;9:23555–23563.
- Maiti C, Parida S, Kayal S, et al. Redox-responsive core-cross-linked block copolymer micelles for overcoming multidrug resistance in cancer cells. ACS Appl Mater Interfaces. 2018;10:5318–5330.
- Li J, Ma YJ, Wang Y, et al. Dual redox/pH-responsive hybrid polymer-lipid composites: synthesis, preparation, characterization and application in drug delivery with enhanced therapeutic efficacy. Chem Eng J. 2018;341:450–461.
- Han L, Zhang XY, Wang YL, et al. Redox-responsive theranostic nanoplatforms based on inorganic nanomaterials. J Control Release. 2017;259:40–52.
- Hadipour Moghaddam SP, Saikia J, Yazdimamaghani M, et al. Redox-responsive polysulfide-based biodegradable organosilica nanoparticles for delivery of bioactive agents. ACS Appl Mater Interfaces. 2017;9:21133–21146.
- Tsang M-K, Wong Y-T, Hao J. Cutting-edge nanomaterials for advanced multimodal bioimaging applications. Small Methods. 2018;2:1700265.
- Li J, Liu K, Chen H, et al. Functional built-in template directed siliceous fluorescent supramolecular vesicles as diagnostics. ACS Appl Mater Interfaces. 2017;9:21706–21714.
- Wang G, Ma Y, Wei Z, et al. Development of multifunctional cobalt ferrite/graphene oxide nanocomposites for magnetic resonance imaging and controlled drug delivery. Chem Eng J. 2016;289:150–160.
- Zhan Y, Shi S, Ehlerding EB, et al. Radiolabeled, antibody-conjugated manganese oxide nanoparticles for tumor vasculature targeted positron emission tomography and magnetic resonance imaging. ACS Appl Mater Interfaces. 2017;9:38304–38312.
- Cormode DP, Si-Mohamed S, Bar-Ness D, et al. Multicolor spectral photon-counting computed tomography: in vivo dual contrast imaging with a high count rate scanner. Sci Rep. 2017;7:4784.
- Xue X, Xu J, Wang PC, et al. Subcellular behaviour evaluation of nanopharmaceuticals with aggregation-induced emission molecules. J Mater Chem C. 2016;4:2719–2730.
- Wang Y, Chen M, Alifu N, et al. Aggregation-induced emission luminogen with deep-red emission for through-skull three-photon fluorescence imaging of mouse. ACS Nano. 2017;11:10452–10461.
- Shi J, Li Y, Li Q, et al. Enzyme-responsive bioprobes based on the mechanism of aggregation-induced emission. ACS Appl Mater Interfaces. 2018;10:12278–12294.
- Sun X, Zebibula A, Dong X, et al. Targeted and imaging-guided in vivo photodynamic therapy for tumors using dual-function, aggregation-induced emission nanoparticles. Nano Res. 2018;11:2756–2770.
- Yeom J, Chang S, Park JK, et al. Synchrotron x-ray bioimaging of bone regeneration by artificial bone substitute of megagen synthetic bone and hyaluronate hydrogels. Tissue Eng C Methods. 2010;16:1059–1068.
- Lee Y-T, Chang Y-T, Chen C-T, et al. The first aggregation-induced emission fluorophore as a solution processed host material in hybrid white organic light-emitting diodes. J Mater Chem C. 2016;4:7020–7025.