Abstract
In the present study, the application of mesoporous silica nanoparticles (MSNPs) loaded with recombinant EspA protein, an immunogen of enterohaemorrhagic E. coli, was investigated in the case of BALB/c mice immunization against the bacterium. MSNPs of 96.9 ± 15.9 nm in diameter were synthesized using template removing method. The immunization of mice was carried out orally and subcutaneously. Significant immune responses to the antigen were observed for the immunized mice when rEspA-loaded MSNPs were administered in both routes in comparison to that of the antigen formulated using a well-known adjuvant, i.e. Freund's. According to the titretitre of serum IL-4, the most potent humoral responses were observed when the mice were immunized subcutaneously with antigen-loaded MSNPs (244, 36 and 14 ng/dL of IL-4 in the serum of mice immunized subcutaneously or orally by antigen-loaded MSNPs, and subcutaneously by Freund’s adjuvant formulated-antigen, respectively). However, the difference in serum IgG and serum IgA was not significant in mice subcutaneously immunized with antigen-loaded MSNPs and mice immunized with Freund’s adjuvant formulated-antigen. Finally, the immunized mice were challenged orally by enterohaemorrhagic E. coli cells. The amount of bacterial shedding was significantly reduced in faecesfaeces of the animals immunized by antigen-loaded MSNPs in both subcutaneous and oral routes.
Introduction
Nanoparticles (NPs) are progressively used in a variety of industries and medicine [Citation1]. Because of many desired properties, different types of NPs have been evaluated as carriers for delivery of vaccines and drugs [Citation2,Citation3]. Vaccine delivery is of a great importance affecting profoundly the vaccine performance. Finding a proper carrier for vaccines has always been a great challenge, especially in the case of subunit vaccines, where the antigen(s) generally is not able to stimulate a potent immune response, alone. Having many desired properties, such as proper size, the ability to escape from lysosomes, keeping the vaccine safe from harsh conditions of the body fluids, etc., NPs have gained many attentions in this regard [Citation4–6]. Polymeric NPs [Citation7,Citation8], liposomes [Citation9,Citation10], virus-like particles (VLPs) [Citation11,Citation12], dendrimers [Citation13,Citation14], inorganic NPs [Citation15,Citation16], etc., have been evaluated as vaccine delivery vehicles and adjutants in recent years. Among the other NPs, mesoporous silica nanoparticles (MSNPs) have many advantages in this regard: the possibility of determining a desired size, that makes it possible to be efficiently taken up by antigen presenting cells (APCs) [Citation17], the possibility of sustained release of the antigen [Citation18], biocompatibility [Citation19,Citation20], biodegradability [Citation21] and the ease of fabrication and storage are the main features of this type of NPs. However, comprehensive studies on the effects of various aspects of the MSNPs, chemical and physical properties on the performance of the MSNPs as immunogenic materials carriers are needed to be able to recommend these types of NPs as suitable carriers for the formulation of vaccines. In this study, we investigate the efficacy of these NPs in combination with a recombinant antigen, rEspA, in immunization of mice, as the animal model, against enterohaemorrhagic E. coli (EHEC).
Also called Shiga toxin producing E. coli (STEC), EHEC are causes of a fatal illness, which the most lethal symptom is the life-threatening hemolytic uremic syndrome (HUS) [Citation22]. The outbreak and sporadic cases of the disease, especially in children under 5 years of age, have been reported from all over the world [Citation23]. Vaccination against the bacteria is an appropriate strategy to prevent the illness. Although, currently there is no approved human vaccine against the bacteria, many studies have identified some potent antigens, which the antibody against them will be increased in naturally infected patients [Citation24,Citation25], and, therefore, they are good choices for developing an efficient subunit vaccine. In this regard, the immune responses to St × 1, St × 2, lipopolysaccharide (LPS), and locus of enterocyte attachment and effacement (LEE)-encoded proteins, mainly Tir, intimin, EspA, and EspB, have been examined in many studies [Citation26–28].
As a product of LEE pathogenicity island, EspA is one of the main bricks of a filamentous protein which is expressed on the bacterial surface, where it connects the bacterial and epithelial cells to each other [Citation29,Citation30]. Being expressed on the surface of the bacteria, the protein will be accessible to the humoral immune system. The issue has been examined previously and the antigenicity of the protein has been confirmed in several reports [Citation31–33]. The main aim of this study is to investigate the efficacy of MSNPs in improving the immunogenicity of the rEspA antigen in comparison to that of a commercially available adjuvant, i.e. Freund’s adjuvant, as schematically illustrated in .
Materials and methods
Materials
Ni-NTA resin was purchased from ShineGene Company (China). Cetyltrimethylammonium bromide (CTAB), tetraethylorthosilicate (TEOS), 3–(2-aminoethyl amino) propyltrimethoxysilane (AAS, 97 vol%), dimethylformamide (DMF), hydrochloric acid, ethanol (99.9%), and glacial acetic acid were purchased from Merck (Germany). N-hydroxysuccinimide (NHS) and N-(3-dimethylaminopropyl)- ethylcarbodiimide hydrochloride (EDC) were received from Sigma-Aldrich (St. Louis, MO, USA). Water was deionized using Q-check controller system (OES Co., USA). pET28a(+) containing espA gene was received from IHU. Female BALB/c mice (7-week old) were purchased from Razi Vaccine and Serum Research Institute (Karaj, Iran).
rEspA protein expression, purification and confirmation
The pET28a(+) plasmid containing espA gene was transformed into competent E. coli BL21(DE3) cells. After the confirmation of transformation, expression of rEspA protein was induced at 37°C by addition of IPTG, at a final concentration of 1 mM. After 4 h, the cells were collected by centrifuging at 8000×g for 2 min. Then, the collected cells were sonicated (amplitude 75, 4 times of 10 s with 30 s intervals). Since the protein was produced as inclusion bodies, the supernatant of the sonicated solution was discarded and the pellet was further lysed by addition of lysis buffer (NaH2PO4 100 mM, Tris-Cl 10 mM, urea 8M) for 2 h with a gentle shaking at 37 °C. After centrifugation (13000×g for 30 min), the protein was purified by passing the supernatant using a Ni-NTA column. Dialysis against acetate buffer (50 mM, pH 5.5) was carried out for removing urea from the protein solution. In order to confirm the expressed protein, using anti-His tag antibody, western blotting was performed [Citation34].
MSNPs synthesis and characterization
Synthesis of MSNPs was performed according to the previously described method, based on template removing technique [Citation35]. Shortly, 0.1 g of CTAB was dissolved in 49 ml of deionized water containing 2 M sodium hydroxide. The mixture was kept on a stirrer with the temperature of 80 °C and constant stirring. After complete dissolving, 1 ml of TEOS was added in a dropwise manner and stirring continued for further 2 h. For removing CTAB, the as-synthesized particles refluxed in an alcoholic solution of hydrochloric acid with a ratio of HCl:EtOH equal to 1:10 (v/v) and were kept on the stirrer for 6 h. Then, the mixture was centrifuged and the pellet was washed with deionized water. For imaging, Hitachi S-5500 electron microscope operating at 30 kV was employed. Bright field scanning transmission electron microscopy (BF-STEM) was also employed for the imaging of the as-prepared MSNPs. The average size of the MSNPs and their size distribution were measured through the size measurement of at least 100 nanoparticles in STEM micrographs using microstructure measurement software. To determine the pore volume, surface area and pore size of MSNPs, Brunauer–Emmett–Teller (BET) isotherms were exploited. A Malvern Zetasizer Nano instrument (S90, UK) equipped with the dynamic light scattering (DLS) system, was used for the measurement of Zeta (ζ) potential values as well as the hydrodynamic diameters of the particles.
Protein adsorption and desorption profiles and kinetics
The net charge of rEspA and the theoretical pI of the protein was calculated using Protein Calculator tool (http://protcalc.sourceforge.net/), in different pH values. This information is used to choose the appropriate pH for the applied buffer in which the protein loading onto MSNPs will occur. Once the optimum pH was chosen, the adsorption tests were conducted in 1 ml of a suitable buffer at the optimum pH, using 500 μg MSNPs and various amounts of rEspA protein in the concentration range of 50–500 μg.
Then, the mixture was shaken at room temperature for 12 h. After centrifugation (5000×g for 30 min), the concentration of protein in the supernatant was measured using Bradford assay and the amount of adsorbed protein onto the MSNPs was estimated by subtracting the supernatant protein concentration from the initial rESPA concentration. Adsorption isotherms were plotted by GraphPad Prism 6 software (GraphPad Software Inc.). The experimental data were fitted in three models: Langmuir, Freundlich and Langmuir–Freundlich.
Protein release from MSNPs was investigated in three different environments, resembling gastric, intestinal and blood fluids [Citation36]. Desorption profile curves were also plotted in each environment by GraphPad Prism6 software.
Mice immunization protocols
Female BALB/c mice (8-week-old, 20 g each) were chosen for the immunization study. The mice were immunized in seven different groups; subcutaneously administered the recombinant rEspA protein in combination with Freund's adjuvant (P-Freudsc); subcutaneously administered the free rEspA protein (Free Proteinsc); subcutaneously administered rEspA-loaded MSNPs (P-MSNPssc); subcutaneously administered the bare MSNPs (Bare MSNPssc); orally administered rEspA-loaded MSNPs (P-MSNPsoral); orally administered the free rEspA protein (Free Proteinoral); and finally, orally administered bare MSNPs (Bare MSNPsoral). Vaccine administration was done four times for each mouse in two-week intervals. For each group of samples, 12 mice were used.
Humoral response analyses
Seven days after second, third and fourth administrations, bleeding was done and the serum was collected for serum IgG and IgA assays. For faecal IgA assay, ten days after the fourth administration, faecal samples were collected and IgA was extracted by the method described by Peters et al. [Citation37]. Indirect enzyme-linked immunosorbent assay (ELISA) was performed to evaluate IgG and IgA antibodies levels in serum and faeces, respectively. The procedure was as described by Voller et al. [Citation38]. Shortly, 4 µg of rEspA was coated in each well. For IgG assay, following the addition of serum to each well and subsequent washing, anti-mouse HRP conjugated-IgG (Sigma, Germany) was added to the wells. For IgA assessment, the same protocol was employed, except that the secondary antibody was anti-mouse IgA, HRP conjugate (Sigma, Germany). The end point titre value (the reciprocal of the highest dilution that has a reading above the cutoff [Citation39]) was determined for each sample.
The concentration of IL-4 in the blood of mice groups was assessed for further evaluation of humoral immunity stimulation. For this purpose, 7 days after the last immunization, bleeding was done and serum was collected. The assessment of IL-4 was performed by R&D IL-4 cytokine assay kit [Citation40].
Challenge analysis
The mice were challenged with 1 × 109 CFU of EHEC O157: H7 (ATCC: 35218 containing espA) 21 days after the last immunization [Citation41]. The day after challenge, and two days intervals after this day, the faeces of the challenged animals were cultured on Sorbitol McConky agar, supplemented with 2.5 mg/L of potassium tellurite and 0.05 mg/L of cefixime, for the detection and quantification of E. coli O157: H7 bacterial cells.
Statistical analysis
All results are the mean of three independent experiments ± standard error mean. Plots were obtained using Prism version 6. Data were analyzed by SPSS version 23. Statistically significant differences calculated using Duncan's method with a p ≤ .05 considered significant.
Results
rEspA protein expression and purification
espA gene was expressed in E. coli BL21 (DE3) by IPTG induction (to a final concentration of 1 mM) and the expression was analyzed by SDS-PAGE. The result showed the presence of a 15 kDa recombinant protein (). Since the protein has been expressed as inclusion bodies, the purification was performed under denaturing conditions [Citation42]. Western blot analysis, using anti-His tag antibody, confirmed the expression of the recombinant protein ().
MSNPs synthesis and characterization
Images obtained by BF-STEM showed that the as-prepared MSNPs are monodisperse and spherical with an average diameter of 96 ± 15 nm (. In addition, DLS technique was also employed to measure the hydrodynamic diameter of the nanoparticles. The results showed that the hydrodynamic diameter of the MSNPs is 113.6 ± 12 nm, which is larger than that obtained from the electron microscopy images.
Figure 2. BF-STEM image of the as-prepared MSNPs (A) and size distribution histogram of the synthesized MSNPs (B).
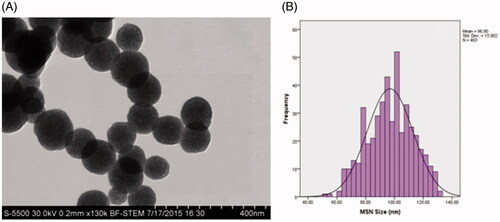
Zeta potential measurement showed that MSNPs have a zeta potential value of −16.1 mV, which indicates that, the MSNPs possess negatively charged surfaces.
Protein adsorption onto MSNPs and determination of adsorption isotherm
The net charge of the protein in different pH values obtained using the theoretical calculation is presented in . From these data, the theoretical pI of rEspA protein was calculated as 8.5. To achieve a suitable adsorption of the protein onto negatively-charged MSNPs, acetate buffer (50 mM, pH 5.5) was chosen for the adsorption studies, in which, rEspA molecules are expected to possess positive net charge in this pH ().
Figure 3. (A) The net charge of the protein in buffers of different pH values. As it can be seen, in acidic buffers, the protein is highly positively charged. (B) Adsorption isotherm of rEspA onto MSNPs. The adsorption was conducted in 1 ml of acetate buffer (50 mM, pH = 5.5) using 500 μg MSNPs and various amounts of rEspA protein, ranging from 50 to 500 μg. (C) Comparison of the protein release profiles from MSNPs different environments: SBF (circles), SIF (square) and SGF (triangle). During the experiments, microtubes were agitated at 150 rpm in 37 °C.
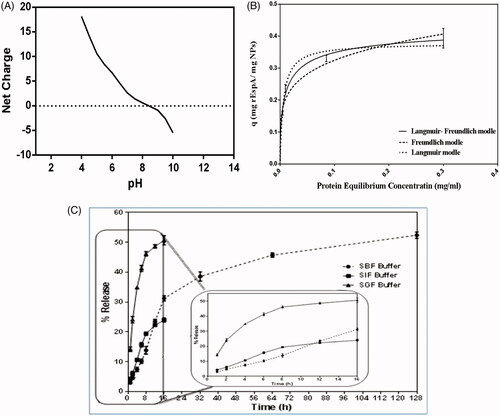
The kinetics of the protein adsorption onto MSNPs was plotted using data obtained for the protein adsorption values in terms of the protein equilibrium concentration (). The plot shows that the protein adsorption best fitted to the Langmuir–Freundlich model.
From this test it was also revealed that the maximum adsorption capacity of MSNPs for rEspA (0.33 mg rEspA/mg MSNPs) is obtained when the applied protein to MSNPs mass ratio was 1:2.5.
Study of the desorption profiles of adsorbed rEspA protein from the MSNPs was performed in 3 different environments, including simulated gastric fluid (SGF), simulated intestinal fluid (SIF) and simulated body fluid (SBF) environments, in 16, 16 and 128-h time periods, respectively. Choosing different periods of times for each buffer is arisen from this fact that the expected residence time is different in each environment. As it is shown in , the highest rate of released protein was observed in SGF (50.67% in 16 h). In SIF and SBF the amount of released protein were 24% after 16 h and 52.4% after 128 h, respectively. It seems that the main reason for the superior desorption of the protein from MSNPs in SGF is due to its acidic condition in comparison to two other fluids. This can considerably negate the electrostatic attractive interactions between protein molecules and silanol groups of MSNPs leading to very high desorption rate of the adsorbed proteins.
Humoral immunity assessment
Serum IgG and IgA and faecal IgA assays
Humoral immunity was assessed by serum IgG and IgA assays. represents the end point titre of the raised antibody against rEspA in each group. As it can be seen, serum IgG was raised significantly in all test groups which received the protein, regardless of the sample composition. While bare MSNPs had no effect on the IgG level. The highest values of the IgG level were observed for P-Freundsc and P-MSNPssc samples indicating that the route of administration has a significant impact on the IgG rise. Very similar results were obtained for the IgA level in the serum ().
Figure 4. The oral (A) and subcutaneous (B) administration of MSNPs-formulated rEspA to mice and the obtained results on Serum IgG (C), serum IgA (D), and faecal IgA (E) titres of test and control groups. The titre of the antibodies was obtained by indirect ELISA in which rEspA was coated on the wells and mouse conjugated antibodies were used for the detection. For abbreviation please see the text.
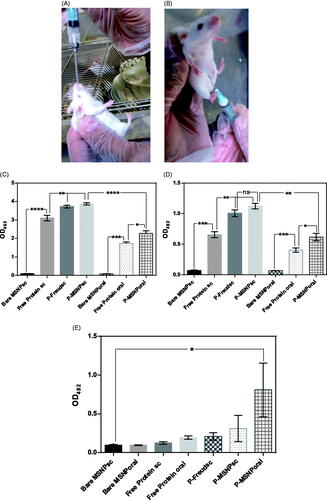
For evaluating the mucosal immunity, the faecal IgA was assessed. The results of the assessment are represented in . Expectedly, the IgA titre in faeces of the group which received protein-loaded MSNPs orally (P-MSNPsoral) was significantly higher than that of the other groups. Similar to serum IgG and IgA assays, the administration of bare MSNPs, orally or subcutaneously did not lead to any significant level of IgA in faeces.
Cytokine assay
IL-4, a Th2 cytokine, was assessed as an indicator of humoral immune responses. The results show that the level of the cytokine in the blood of mice which received protein-loaded MSNPs subcutaneously (P-MSNPssc) is strikingly increased, while no significant increase is observed in other routes ().
Challenge
In order to evaluate the protectivity effect of the raised antibodies, animal groups were challenged by oral administration of 1010 CFU of live pathogenic EHEC bacteria. The results showed that the extent of reduction in the load of bacteria in the faeces of mice groups administered with MSNPs-formulated rEspA, i.e. orally immunized with protein-loaded MSNPs (P-MSNPsoral), subcutaneously immunized with protein-loaded MSNPs (P-MSNPssc), as well as subcutaneously immunized with protein + Freund's adjuvant (P-Freundsc) is much higher than those of other groups (), indicating higher values of protection caused by formulations in which MSNPs or Freud adjuvant have been applied.
Discussion
Delivery of vaccines by carriers has many advantages. Protecting the vaccines from harsh conditions in the body and the sustained release are two important advantages of using vaccine carriers. Many different types of carriers have been used for vaccine delivery in recent years, however, because of unique and desirable features, nanoparticles have acquired special interests. Silica nanoparticles have recently attracted a great deal of attention as vaccine carriers [Citation43].
In the present study, the potential of MSNPs in delivery of an antigen (EspA) was investigated. We showed that administration of the EspA-loaded MSNPs through oral or subcutaneous routes can reduce the shedding of enterohaemorrhagic E. coli in immunized mice, in an extent comparable with the administration of this antigen formulated with one of the most common and commercially available adjuvant, i.e. Freund's adjuvant.
In the first step, the spherical MSNPs with an average diameter of ∼97 nm were synthesized using template removing method. NPs of this size range are suitable for vaccine delivery due to their easy uptake by immune cells via endocytosis [Citation44]. In our previous research, analysis of the porosity of the NPs using measurement of nitrogen adsorption-desorption isotherms by multipoint Brunauer–Emmett–Teller (BET) apparatus showed that the average diameter of the pores is about 4 nm (data not shown). This indicates that EspA protein (whit a diameter of about 2 nm) can penetrate into the NPs, so it can be protected from harsh conditions in vivo.
Highest values of the protein adsorption onto negatively charged MSNPs was obtained when acetate buffer pH 5.5, was used, due to increasing of the electrostatic interactions between the MSNPs and positively charged protein molecules under this condition. Further analysis of the adsorption results showed that the protein adsorption is best fitted to the Langmuir–Freundlich model. This indicates that there is more than one layer of adsorbed protein onto the NPs [Citation45], a phenomenon that is expectable owing to the existence of different hydrophobic and hydrophilic moieties on the protein, which accelerates the aggregation of the protein.
Protein desorption profiles from MSNPs were investigated in three different environments, i.e. fasted-state SGF, fasted-state SIF and SBF. The first two environments were exploited to study the release status following oral administration, and the third environment was used for investigating the protein desorption following subcutaneous administration of the immunogenic materials. The largest desorption rate was seen in the fasted-state SGF, where there is a burst release in the first hour, a phenomenon that is not observed in another two environments (SIF and SBF). However, the in silico calculated net charge of the protein is very high (about +22.9) in the gastric fluid with pH 2 and slower release profiles of that from the negatively charged surfaces, such as MSNPs, was expected. This contradiction can be attributed to the high concentration of salts in the fasted-state SGF in comparison to those of two other buffers, and consequently weaker protein adsorption onto MSNPs.
The challenge by live pathogenic bacteria was carried out to evaluate the protection efficiency of the raised antibodies. In order to investigate humoral immunity stimulation, serum IgG and IgA titres in the sera of animals were assessed. The results showed that the level of these antibodies in the sera of the immunized mice (all immunized groups) significantly increases and is much larger than those of other samples (p < .001). These results indicate that the subcutaneous administration of vaccine is more efficient than the oral route in stimulating the humoral immune system, as it was expected. Interestingly, the formulation of protein-based vaccine with MSNPs leads to the similar values of the immune system stimulation obtained when formulation was carried out with very strong Freund’s adjuvant. So, since the administration of the candidate vaccines with Freund's adjuvant may cause irritation in the site of injection, it seems that these NPs have the potential to be used as adjuvant instead of Freund's.
Assessment of IL-4 level in sera of the examined mice, as an indicator of humoral immunity stimulation, showed that the subcutaneous administration of MSNPs-formulated EspA protein presents the highest values of the stimulation in comparison to other samples. These results were in agreement with the results obtained by Hirai et al. [Citation46]. In that work, it was shown that the IL-4 level in mice that receive the antigen formulated with amorphous silica nanoparticles (with an average diameter of ∼300 nm) significantly increases in comparison to the group that receives the free antigen.
The assessment of the IgA titre in faeces of the immunized mice showed that the mucosal immunity just for those treated with P-MSNPsoral is provoked and its extent is significantly higher than that of the free protein (p < .05). The phenomenon is expectable, because the administration of vaccines via oral route stimulates the gut immune system more efficiently than other routes. However, in the challenge study, shedding was not significantly different among the MSNPs-formulated samples in comparison to that of the formulated with Freund’s adjuvant. These results indicate that IgG antibodies penetration to the gut lumen and consequently its ability to neutralize the pathogen in there is completely dependent on the formulation of EspA using MSNPs or Freund’s adjuvant regardless of the formulation approach. Although the pathogen was not completely removed from the gut of any tested groups after 15 days, however, the decrease of the colonization and shedding was significant in comparison to that of the sample treated with the free protein (p < .01).
In summary, the results of the present study recommend MSNPs as an appropriate carrier for the formulation of recombinant subunit vaccines. Further studies are required to elucidate the mechanism governing the stimulation of the immune system by MSNPs-formulated proteins as well as to indicate the biocompatibility aspects of the MSNPs-based adjuvants.
Acknowledgements
Authors sincerely thank to National Institute of Genetic Engineering and Biotechnology (project No. 450) and Baquiyatallah University of Medical Sciences for animal house facilities.
Disclosure statement
No potential conflict of interest was reported by the authors.
References
- Zhang L, Gu F, Chan J, et al. Nanoparticles in medicine: therapeutic applications and developments. Clin Pharmacol Ther. 2008;83:761–769.
- Hajizade AF, Ebrahimi A-H, Salmanian A, et al. Nanoparticles in vaccine development. J Appl Biotechnol Rep 2015;1:125–134.
- Yih T, Al‐Fandi M. Engineered nanoparticles as precise drug delivery systems. J Cell Biochem. 2006;97:1184–1190.
- Kammona O, V, Bourganis T, Karamanidou C, et al. Recent developments in nanocarrier-aided mucosal vaccination. Nanomedicine. 2017;12:1057–1074.
- López-Sagaseta J, Malito E, Rappuoli R, et al. Self-assembling protein nanoparticles in the design of vaccines. Comput Struct Biotechnol J. 2016;14:58–68.
- Zhao L, Seth A, Wibowo N, et al. Nanoparticle vaccines. Vaccine. 2014;32:327–337.
- Rahimian S, Kleinovink JW, Fransen MF, et al. Near-infrared labeled, ovalbumin loaded polymeric nanoparticles based on a hydrophilic polyester as model vaccine: In vivo tracking and evaluation of antigen-specific CD8+ T cell immune response. Biomaterials. 2015;37:469–477.
- Tiyaboonchai W. Chitosan nanoparticles: a promising system for drug delivery. วรสารมหาาวทยาลย นเรศวร (Naresuan Univ J) 2013;11:51–66.
- Fan Y, Sahdev P, Ochyl LJ, et al. Cationic liposome–hyaluronic acid hybrid nanoparticles for intranasal vaccination with subunit antigens. J Control Release. 2015;208:121–129.
- Swaminathan G, Thoryk EA, Cox KS, et al. A novel lipid nanoparticle adjuvant significantly enhances B cell and T cell responses to sub-unit vaccine antigens. Vaccine. 2016;34:110–119.
- Jain NK, Sahni N, Kumru OS, et al. Formulation and stabilization of recombinant protein based virus-like particle vaccines. Adv Drug Deliv Rev. 2015;93:42–55.
- Treanor JJ, Atmar RL, Frey SE, et al. A novel intramuscular bivalent norovirus virus-like particle vaccine candidate—reactogenicity, safety, and immunogenicity in a phase 1 trial in healthy adults. J Infect Dis. 2014;210:1763–1771.
- Lu F, Mencia A, Bi L, et al. Dendrimer-like alpha-D-glucan nanoparticles activate dendritic cells and are effective vaccine adjuvants. J Control Release. 2015;204:51–59.
- Blanco E, Guerra B, Beatriz G, et al. Full protection of swine against foot-and-mouth disease by a bivalent B-cell epitope dendrimer peptide. Antivir Res. 2016;129:74–80.
- Niikura K, Matsunaga T, Suzuki T, et al. Gold nanoparticles as a vaccine platform: influence of size and shape on immunological responses in vitro and in vivo. ACS Nano. 2013;7:3926–3938.
- Jin H, Qian Y, Dai Y, et al. Magnetic enrichment of dendritic cell vaccine in lymph node with fluorescent-magnetic nanoparticles enhanced cancer immunotherapy. Theranostics. 2016;6:2000.
- Bachmann MF, Jennings GT. Vaccine delivery: a matter of size, geometry, kinetics and molecular patterns. Nat Rev Immunol. 2010;10:787–796.
- Slowing II, Trewyn BG, Lin VS-Y. Mesoporous silica nanoparticles for intracellular delivery of membrane-impermeable proteins. J Am Chem Soc. 2007;129:8845–8849.
- Asefa T, Tao Z. Biocompatibility of mesoporous silica nanoparticles. Chem Res Toxicol. 2012;25:2265–2284.
- Trewyn BG, Nieweg JA, Zhao Y, et al. Biocompatible mesoporous silica nanoparticles with different morphologies for animal cell membrane penetration. J Chem Engin. 2008;137:23–29.
- Kempen PJ, Greasley S, Parker KA, et al. Theranostic mesoporous silica nanoparticles biodegrade after pro-survival drug delivery and ultrasound/magnetic resonance imaging of stem cells. Theranostics 2015;5:631.
- Siegler R, Oakes R. Hemolytic uremic syndrome; pathogenesis, treatment, and outcome. Curr Opin Pediatr. 2005;17:200–204.
- Karch H, Mellmann A, Bielaszewska M. Epidemiology and pathogenesis of enterohaemorrhagic Escherichia coli. Berliner Und Munchener Tierarztliche Wochenschrift 2008;122:417–424.
- Li Y, Frey E, Mackenzie AM, et al. Human response to escherichia colio157: h7 infection: antibodies to secreted virulence factors. Infect Immun. 2000;68:5090–5095.
- Moriel DG, Bertoldi I, Spagnuolo A, et al. Identification of protective and broadly conserved vaccine antigens from the genome of extraintestinal pathogenic Escherichia coli. Proc Natl Acad Sci. 2010;107:9072–9077.
- Marcato P, Griener TP, Mulvey GL, et al. Recombinant Shiga toxin B-subunit-keyhole limpet hemocyanin conjugate vaccine protects mice from Shigatoxemia. Infect Immun. 2005;73:6523–6529.
- Gu J, Liu Y, Yu S, et al. Enterohaemorrhagic Escherichia coli trivalent recombinant vaccine containing EspA, intimin and Stx2 induces strong humoral immune response and confers protection in mice. Microbes Infect. 2009;11:835–841.
- Hajizade AF, Ebrahimi J, Amani A, et al. Design and in silico analysis of pentavalent chimeric antigen against three enteropathogenic bacteria: enterotoxigenic E. coli, enterohemorragic E. coli and Shigella. Biosci Biotech Res Comm. 2016;9:225–239.
- Knutton S, Rosenshine I, Pallen MJ, et al. A novel EspA‐associated surface organelle of enteropathogenic Escherichia coli involved in protein translocation into epithelial cells. EMBO J. 1998;17:2166–2176.
- Ebel F, Podzadel T, Rohde M, et al. Initial binding of Shiga toxin‐producing Escherichia coli to host cells and subsequent induction of actin rearrangements depend on filamentous EspA‐containing surface appendages. Mol Microbiol. 1998;30:147–161.
- Jenkins CH, Chart H, Smith E, et al. Antibody response of patients infected with verocytotoxin-producing Escherichia coli to protein antigens encoded on the LEE locus. J Med Microbiol. 2000;49:97–101.
- Mahony D, Cavallaro AS, Stahr F, et al. Mesoporous silica nanoparticles act as a self‐adjuvant for ovalbumin model antigen in mice. Small. 2013;9:3138–3146.
- Tu J, Boyle AL, Friedrich H, et al. Mesoporous silica nanoparticles with large pores for the encapsulation and release of proteins. ACS Appl Mater Interfaces. 2016;8:32211–32219.
- Mahmood T, Yang P-C. Western blot: technique, theory, and trouble shooting. North Am J Med Sci. 2012;4:429.
- Taebnia N, Morshedi D, Doostkam M, et al. The effect of mesoporous silica nanoparticle surface chemistry and concentration on the α-synuclein fibrillation. RSC Adv. 2015;5:60966–60974.
- Marques MR, Loebenberg R, Almukainzi M. Simulated biological fluids with possible application in dissolution testing. Dissolution Technol. 2011;18:15–28.
- Peters I, Calvert E, Hall E, et al. Measurement of immunoglobulin concentrations in the faeces of healthy dogs. Clin Diagn Lab Immunol. 2004;11:841–848.
- Voller A, Bartlett A, Bidwell D. Enzyme immunoassays with special reference to ELISA techniques. J Clin Pathol. 1978;31:507–520.
- Frey A, Di Canzio J, Zurakowski D. A statistically defined endpoint titre determination method for immunoassays. J Immunol Methods. 1998;221:35–41.
- Silva D, Ponte CG, Hacker MA, et al. A whole blood assay as a simple, broad assessment of cytokines and chemokines to evaluate human immune responses to Mycobacterium tuberculosis antigens. Acta Tropica. 2013;127:75–81.
- Judge NA, Mason HS, O'Brien AD. Plant cell-based intimin vaccine given orally to mice primed with intimin reduces time of Escherichia coli O157: H7 shedding in faeces. Infect Immun. 2004;72:168–175.
- Hoffmann A, Roeder R. Purification of his-tagged proteins in non-denaturing conditions suggests a convenient method for protein interaction studies. Nucl Acids Res. 1991;19:6337.
- Gordon S, Teichmann E, Young K, et al. In vitro and in vivo investigation of thermosensitive chitosan hydrogels containing silica nanoparticles for vaccine delivery. Eur J Pharm Sci. 2010;41:360–368.
- Xiang SD, Scholzen A, Minigo G, et al. Pathogen recognition and development of particulate vaccines: does size matter? Methods. 2006;40:1–9.
- Chen X. Modeling of experimental adsorption isotherm data. Information. 2015;6:14–22.
- Hirai T, Yoshikawa T, Nabeshi H, et al. Amorphous silica nanoparticles size-dependently aggravate atopic dermatitis-like skin lesions following an intradermal injection. Part Fibre Toxicol. 2012;9:3.