Abstract
Gramicidin is a well-known antibiotic and recently was reported to induced tumour cell death, however, little is understood about the molecular mechanism of gramicidin as a therapeutic agent for solid tumours. Here, we investigated the role of gramicidin in cholangiocarcinoma cells. We found that gramicidin A inhibits cholangiocarcinoma cell growth and induced the necrotic cell death. We used next generation sequencing to analyse gene expression profiles of cholangiocarcinoma cells treated with gramicidin. We identified 265 differentially expressed genes in cholangiocarcinoma cells between PBS treatment and gramicidin treatment. EGR4 was confirmed to be a target of gramicidin-induced cell growth inhibition. Furthermore, we demonstrated that downregulation of EGR4 in cholangiocarcinoma cells leads to restraining tumour cell growth. Of note, EGR4 was expressed at highest levels in cholangiocarcinoma tissues among 17 types of human cancers, and EGR4 expression positively correlated with several growth factors associated with cholangiocarcinoma. Our findings ascertain that EGR4 is a potential target in cholangiocarcinoma and suppressing EGR4 by gramicidin establish an essential mechanism for bile duct carcinoma progression.
Introduction
Cholangiocarcinoma is a highly invasive malignant neoplasm derive from biliary duct epithelial cells. According to the location of the pathological structure, it can be divided into two types: extrahepatic cholangiocarcinoma (eCCA) and intrahepatic cholangiocarcinoma (iCCA) [Citation1]. The former refers to malignant tumours of bile duct epithelial origin located from the left and right hepatic ducts to the lower end of the common bile duct, which are more common in clinic, accounting for about 9/10 of the total number of CCA; the latter refers to malignant tumours of bile duct epithelial cells occurring below the second branch of the intrahepatic bile duct [Citation2]. Patients with cholangiocarcinoma usually present at late stages of the disease, therefor these cancers remain difficult to diagnose and treat and their prognosis is generally poor and the 5 years survival rate for malignant cholangiocarcinoma was 5% and 17% for intra and extrahepatic ducts cancer [Citation3–5]. With the advance of high-throughput sequencing and the Tumour Genome Atlas (TCGA) data analysis of cholangiocarcinoma, the molecular heterogeneity and driver mutations of the different types of cholangiocarcinoma are identified. In addition to the known tumour related genes TP53, KRAS and SMAD4, several inactivating mutation genes including BAP1, ARID1A, PBRM1, MLL3, ROBO2, RNF43 and PEG3 were identified in iCCA or opisthorchis viverrini-related cholangiocarcinoma [Citation6,Citation7]. Thus, the search for therapeutic targets and effective pharmacological treatment in cholangiocarcinoma still need to be explored.
Gramicidin are a class of polypeptide substances, which are extracted from the culture of bacillus brevis and the family members include gramicidin A, gramicidin B, gramicidin C, gramicidin D and gramicidin S [Citation8,Citation9]. The gramicidin A is the best characterised channel forming ionophore and two monomers dimerise to form a functional nanopore within the lipid bilayer [Citation10]. The formation of gramicidin A channel renders biological membranes permeable to specific cations which disrupts cellular ionic homeostasis and then changes the permeability of cells, so that cells can not normally carry out metabolic activities and be inhibited or even died [Citation11]. For quite a long time, the research on gramicidin A is more about making full use of its cationic channel characteristics as a carrier of related substances or modifying it to make it a better intramembrane transport. Recently in renal cell carcinoma, gramicidin A was found to block tumour growth and tumour cell metabolic dysfunction [Citation10,Citation12,Citation13]. However, use of gramicidin A as an antitumor agent has not been widely evaluated on other solid tumours.
In the present study, we reported that gramicidin inhibits cell growth and induce necroptotic cell death in cholangiocarcinoma. Further study demonstrated that EGR4 is downregulated in cholangiocarcinoma cells treated with gramicidin and silencing of EGR4 reduce the cell proliferation in cholangiocarcinoma cells. Clinical sample analysis shown that EGR4 is highly expressed in cholangiocarcinoma and positively correlated with growth factors, which suggest that EGR4 is a key positive regulator in human cholangiocarcinoma and downregulating EGR4 by gramicidin provides a therapeutic application for cholangiocarcinoma.
Materials and methods
Cell culture and reagents
The human cholangiocarcinoma cell line RBE and HuCCT1 was obtained and authenticated from the Shanghai Institute of Cell Biology, Chinese Academy of Sciences (Shanghai, China). Cells were cultured in Dulbecco’s Modified Eagle’s Medium (DMEM) supplemented with 10% foetal bovine serum (FBS), 2 mM L-glutamine, and 5% penicillin/streptomycin at 37 °C in a humidified atmosphere with 5% CO2 in air. Gramicidin (CAS no 1405-97-6, purity: 99%) was purchased from Target Molecule Corp (Shanghai, China).
Patients data
We downloaded EGR4 and NFKB1/EGFR/PIK3CA expression data from a TCGA data online analysis tool (starbase.sysu.edu.cn) [Citation14,Citation15] and for the correlation analyses between EGR4 and NFKB1/EGFR/PIK3CA gene expression, Pearson correlation coefficients were calculated. To analyse the expression of EGR4, tumour tissues, tumour adjacent tissues and normal tissues were obtained from 10 cholangiocarcinoma patients. All patients were from the department of hepatobiliary pancreatic surgery, the First Affiliated Hospital of Guangzhou Medical University (Guangzhou, China). The study protocol was approved by the Clinical Research Ethics Committee of the Guangzhou Medical University and Guangzhou University of Chinese Medicine. Written informed consent was obtained from all subject before study.
RNA preparation and sequencing
RBE cells were treated with gramicidin for 12 h and total RNA was extracted using the TRI Reagent and a Direct-zolTM RNA Kit (Zymo Research, Irvine, CA) following the manufacturer’s instructions. Quality and quantity of RNA were assessed on an Agilent Bioanalyzer 2100 (Agilent Technologies, Santa Clara, CA) to ensure an A260/A280 ratio in the range of 1.8–2.0 and an rRNA ratio (28S/18S) >1.0. DNA was removed by DNase I. Poly(A) RNAs were purified by using a Dynabeads mRNA Purification Kit (Invitrogen, Carlsbad, CA) according to the manufacturer’s instructions. Poly(A) RNAs were fragmented at 94 °C for 5 min before being reverse transcribed to cDNA with random primers. cDNA fragments were blunted and ligated to sequencing adaptors. Libraries were sequenced with the High-Seq 2000 Illumina sequencing platform by a sequencing service company (RIBOBIO, Guangzhou, China).
Quantitative real-time PCR
Total cellular RNA was isolated by using Trizol (Invitrogen) according to the manufacturer's recommendations. RNA was reverse transcribed with the iScript Reverse Transcription Supermix kit (Bio-Rad, Hercules, CA, USA) following the manufacturer's protocol. qPCR for EGR4 detection was performed using SYBR Green Supermix (Bio-Rad Laboratories) and a CFX96 Real-time PCR detection system (Bio-Rad Laboratories). We analysed each sample in duplicate with Gapdh as a reference.
Cell transfection
For knockdown of EGR4, the shRNA1-EGR4: GCTACAGCGGTAGCTTCTTCA; shRNA2-EGR4: GGACCAAGATTGAGGACTTGC; and nonsense sequence to EGR4 (shRNAControl): GGTGTGCAGTTGGAATGTA were synthesised and ligase into psiF-copGFP vectors (System Biosciences, Mountain View, CA). 293 T cells were seeded in a 10 cm plate and transfected with 5 μg pRSV-rev, 5 μg pMDL-rre, 5 μg pMD2.G, and 10 μg shRNA plasmid construct. Then the cells were cultured at 37 °C for 48 h, and the virus was harvested by centrifuging the cells at 2000 g for 10 min followed by filtrating it through 0.45 μm filter. The RBE and HuCCT1 cells were transduced with 500 μL of the virus in the presence of 8 μg/mL of polybrene for 6 h, and then change the medium. After 48 h, the cells were collected and performed the western blot assay to ensure silencing was achieved.
Cell proliferation and colony formation assay
Cell proliferation was determined by using Cell Counting Kit-8 (CCK-8) kit (Dojindo Molecular Technologies, Inc). Briefly, cells were seeded in 96-well plate and transfected with shRNAEGR4. Cell colony formation assay, 5 × 103 cells were suspended in 0.3% agar in DMEM supplemented with 1% penicillin/streptomycin, 1% L-glutamine, and 10% FBS and overlaid on 0.5% agar in the same medium in six-well plates and maintained in an incubator for 4 weeks. Colonies were stained with 0.005% crystal violet, and >0.1 mm in diameter were scored by microscopic examination and photographed with a dissection microscope.
Western blot
For western blotting analyses, cells were lysed with RIPA buffer (Cell Signalling), supplemented with protease inhibitor (Calbiochem, Billerica, MA, USA). Protein concentrations were measured with the Pierce BCA Protein Assay Kit according to the manufacturer's manual (Thermo Fisher Scientific, Waltham, MA, USA). Soluble proteins were subjected to SDS-polyacrylamide gel electrophoresis before being transferred to polyvinylidene difluoride (PVDF) membranes for blotting. The membrane was blocked with 5% non-fat dry milk for 1 h and incubated with primary antibody at 4 °C overnight. The antibodies of EGR4 (A2910) and β-actin (AC006) were purchased from ABclonal Technology (Wuhan, China). After incubated with primary antibody, the membranes were incubated with either horseradish peroxidase conjugated anti-rabbit or anti-mouse secondary antibody. The immunoreactive proteins were visualised with SuperSignal West Dura Chemiluminescent (Thermo Fisher Scientific, USA). Gel pictures were scanned by automatic chemiluminescence imaging analysis system Tanon4600 and acquired using TanonPVCam software (Tanon, China).
Data availability and correlation analysis
The Cancer Genome Atlas (TCGA) RNA-seq data for lung adenocarcinoma and squamous cell carcinoma of cancer patients were downloaded from starbase.sysu.edu.cn [Citation14].
Statistical analyses
All statistical analysis was carried out using SPSS16.0 software (SPSS Inc. Chicago, IL, USA). An unpaired two-tailed Student’s t-test was performed for two-group comparisons and one-way analysis of variance (ANOVA) analysis was performed for multiple group comparisons. For the correlation analyses Pearson correlation coefficients were calculated. The statistical significance was set at a p-value < .05 and values were presented as means ± SD.
Results
Gramicidin inhibited cholangiocarcinoma cell growth and induced necrotic cell death
Gramicidin is a linear 15 residue peptide of amino acids and functions as an antibiotic by disrupt cellular transmembrane potential (. To explore the role of gramicidin in cholangiocarcinoma cell proliferation, we treated two cholangiocarcinoma cell lines, RBE and HuCCT1, with gramicidin. We found that cholangiocarcinoma cell lines were sensitive to gramicidin treatment and the IC50 for both cell lines was as lower as 50 nM (. In addition, the two type of cholangiocarcinoma cell lines were all shown the necrotic cell death demonstrated as floating cellular debris (. To further analyse the cytotoxicity of gramicidin, we detect the necrotic cell death by using Calcein AM (green, live cells) and ethidium homodimer-1 (red, necrotic dead cells) staining. As shown in , RBE and HuCCT1 cells exhibited necrotic dead cells after gramicidin treatment.
Figure 1. Gramicidin inhibit cell growth and induce necroptotic cell death in cholangiocarcinoma. (A) Chemical structures of gramicidin. Cell viability was determined using CCK-8 assay in RBE (B) and HuCCT1 (C) treated with gramicidin at indicated concentrations and times. (D) To detect necrotic cell death, RBE cells were stained with Calcein AM (green, live cells) and ethidium homodimer-1 (red, necrotic cell death) 12 h after gramicidin treatment. Scale bars = 200 μm. Values represented the mean ± SD of three independent experiments. *p < .05 and **p < .01 indicate a significant difference between none treatment (0 nM) and gramicidin treatment (1 nM, 5 nM, 10 nM, 50 nM and 100 nM).
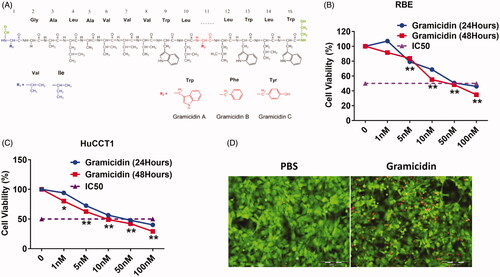
RNA-sequencing revealed that EGR4 is downregulated in cholangiocarcinoma cells treated with gramicidin
To identify the potential therapeutic targets in cholangiocarcinoma cells treated with gramicidin, we performed RNA-sequencing (RNA-seq) using total RNA isolated from RBE cells treated with PBS or gramicidin for 12 h. After quality filtering and normalising the raw sequencing data, we identified differentially expressed genes (DEGs) based on the following criteria: fold change >2 or <0.5, p-value <.05. By comparing the reads per kilobase per million (RPKM) values between PBS and gramicidin treatment, a total of 265 DEGs (64 upregulated and 201 downregulated) were identified in RBE cells treated with gramicidin. Ingenuity Pathway Analysis (IPA) shown that most of enriched canonical pathway was involved the role of granulocyte adhesion, agranulocyte adhesion and IL-17A. Of note, several cholesterol biosynthesis pathways were enriched between PBS and gramicidin treatment, which indicated the possible key role of lipid metabolism pathway in cholangiocarcinoma cell growth (). Next, we found that early growth response protein 4 (EGR4) is one of the most down-regulated gene in identified DEGs (. Next, we performed quantitative real-time PCR (qPCR) to validate the RNA-seq data and found the mRNA of EGR4 was dramatically down-regulated in both RBE and HuCCT1 cholangiocarcinoma cells (. Accordingly, the protein level of EGR4 was significantly lower in RBE and HuCCT1 cells after gramicidin treatment (. These results implicate that gramicidin inhibits cholangiocarcinoma cell growth by downregulating EGR4 and EGR4 is a potential target in cholangiocarcinoma.
Figure 2. RNA sequencing revealed that EGR4 is a potential therapy target for RBE and HuCCT1 cells. (A) Ingenuity pathway analysis of biological functions in RBE cells treated with gramicidin. (B) RNA-seq analysis of RBE cells treated with PBS or gramicidin. Egr4 was the top outlier gene among the downregulated genes (left, green). Egr4 mRNA expression in RBE (C) or HuCCT1 (D) cells treated with gramicidin (50 nmol/L) at the indicated time point. Egr4 protein expression in RBE (E) or HuCCT1 (F) cells treated with gramicidin (200 nmol/L) at the indicated time point. Values represented the mean ± SD of three independent experiments. *p < .05 and **p < .01 indicate a significant difference between non-treatment (0 h) and treatment with gramicidin (6 h, 24 h, 48 h).
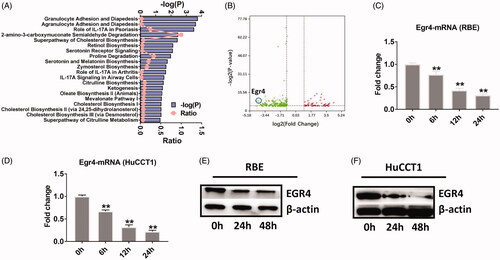
Inhibition of EGR4 reduces cellular proliferation in cholangiocarcinoma cell lines
To determine whether EGR4 is a potential target in cholangiocarcinoma, we first measured the expression of EGR4 in noncarcerous epithelial cell line 16HBE and chalangiocarcinoma cell lines RBE and HuCCT1. We found that EGR4 was highly expressed in chalangiocarcinoma cell lines as compared with 16HBE cells as determined by qPCR (. Next, we introduced shRNA of EGR4, which led to downregulation of EGR4 protein expression in RBE and HuCCT1 cells (. We found that EGR4 inhibition with shRNA-EGR4 at Day1, Day2, Day3 and Day4 significantly reduced the cell growth and colony formation of RBE and HuCCT1 cells (). To further demonstrate gramicidin inhibits cell proliferation through EGR4, we introduced shRNA of EGR4 into RBE and HuCCT1 cells. And we found there is no significant difference of cell proliferation in RBE and HuCCT1 cells between untreatment and gramicidin treatment, when EGR4 was knockdown with shRNA (. Our data provide the evidence that inhibition of EGR4 expression contributes to attenuated cellular growth and further demonstrate that gramicidin significantly downregulate EGR4 expression and inhibit cholangiocarcinoma cell growth through EGR4.
Figure 3. The effects of EGR4 on cell proliferation in RBE and HuCCT1. (A) qPCR analyses of EGR4 expression in cholangiocarcinoma cell lines (RBE and HuCCT1) compared to a noncancerous cell line (16HBE). (B) Expression of GFP from the shRNAControl or shRNAEGR4 vector in RBE and HuCCT1 cells. Scal bars = 100 μm. (C) Western blotting analyses of EGR4 expression in RBE cell transfected with shRNAControl (shControl) or shRNAEGR4 (shEGR4). RBE (D) and HuCCT1 (E) cells were transfected with shControl or shEGR4, and incubated for 1 day, 2 days, 3 days and 4 days after transfection, and cell viability was determined by counting cells labelled with CCK-8. (F) RBE and HuCCT1 cells were transfected with shControl or shEGR4 and foci were stained after 21 days of growth. RBE (G) and HuCCT1 (H) cells were transfected with shControl or shEGR4 and cells were further treated with gramicidin for 24 h. *p < .05 and **p < .01 indicate a significant difference between shControl transfection and shEGR4 transfection. NS indicate no significant.
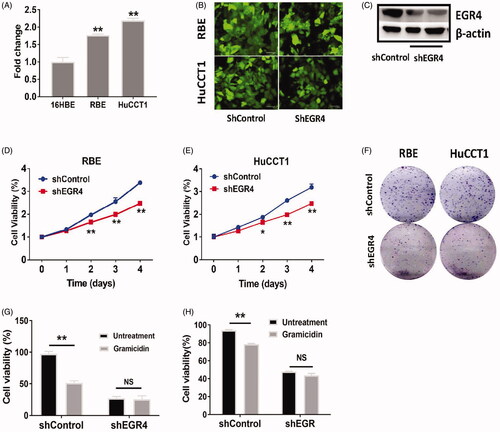
Expression of EGR4 in human cholangiocarcinoma
We obtained EGR4 expression profiles in various types of human cancers and adjacent normal tissues from a TCGA data online analysis tool (starbase.sysu.edu.cn) [Citation14]. As shown in , EGR4 was expressed at highest levels in cholangiocarcinoma tissues–14.54-fold upregulation (. We next analysed expression of EGR4 in human cholangiocarcinoma in the TCGA database. EGR4 expression was significantly higher in cholangiocarcinoma tissues than that in normal tissues (. We then determined whether the expression level of EGR4 is associated with patient survival. However, we did not find significant relationship between EGR4 expression and patient survival (p = .07) and the reason is possible due to the less sample size (, 36 cancer and 9 normal samples in cholangiocarcinoma). In consistent with TCGA data, EGR4 was up-regulated in 8 of 10 clinical cholangiocarcinoma patients (. Correspondingly, the protein level of EGR4 was highest expressed in tumour tissue compared to its adjacent and normal tissues (. Furthermore, we analysed the correlative expression of EGR4 and several growth factors which are implicated in cholangiocarcinoma carcinogenesis. We found that positive correlation between EGR4 and these growth factors including NFKB1 (r = 0.447, p = .00627, ), EGFR (r = 0.4, p = .0157, ), PIK3CA (r = 0.349, p = .0371, . Taken together, our data indicate EGR4 is a potential target in cholangiocarcinoma.
Figure 4. EGR4 expression in human cholangiocarcinoma patients. (A) EGR4 expression in 17 types of cancers compared to their counterpart normal tissue samples from TCGA database. (B) EGR4 expression in human cholangiocarcinoma samples compared to normal samples in the TCGA database. (C) Kaplan-Meier analysis (log-rank test) of overall survival of human cholangiocarcinoma samples from TCGA database based on the expression of EGR4. Highly expressed mRNA (D) and Protein (E) in human cholangiocarcinoma were validated in an independent cohort. (F)–(H) Correlation analyses between EGR4 and several growth factors, NFKB1 (F), EGFR (G), and PIK3CA (H) which are implicated in cholangiocarcinoma carcinogenesis in the TCGA database. *p < .05 and **p < .01 indicate a significant difference between normal tissue and tumour tissue. NS indicate no significant.
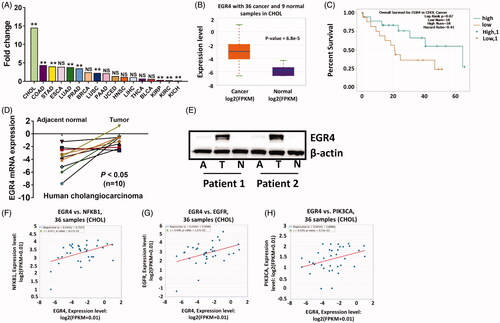
Discussion
Gramicidin A is a well-known antibiotic that is pivotal in forming ion channel ionophore, rendering biological membranes permeable to specific cations which disrupts cellular ionic homeostasis and eventually leads to cell death. Recent findings revealed that gramicidin A possesses anticancer properties in renal cell carcinoma, which indicate the therapeutic potential for the treatment of other solid tumours. In the present work, we investigated the role of gramicidin A in cholangiocarcinoma cells. Consisted with previous study in renal cell carcinoma, our data shown gramicidin A significantly inhibit two cholangiocarcinoma cell lines, RBE and HuCCT1, growth and induce necrotic cell death. Of note, compared to renal cell carcinoma cell lines treated with gramicidin A, which the IC50 is from 0.1 μmol/L to 0.7 μmol/L [Citation10], the cholangiocarcinoma cells exhibit more sensitivity to gramicidin treatment and the IC50 for both cell lines treated with gramicidin A is around 0.05 μmol/L. Our results suggest that gramicidin A have the therapeutic potential for the treatment of cholangiocarcinoma.
Next-generation sequencing reveals the complexity and heterogeneity of the different types of cholangiocarcinoma and the potential druggable targets in different subtypes of cholangiocarcinoma. For example, 47% of the cholangiocarcinoma exhibit loss or mutation of CDKN2 gene and inactivating mutations result in deregulation of cell cycle [Citation16]. Mutations of fibroblast growth factor (FGF) accounts in 10 up to 45% of all intrahepatic cholangiocarcinoma and FGF receptor inhibitors shown anti-tumour activity and entered early clinical trials [Citation17]. By using RNA-sequencing, we identify the potential therapeutic targets in cholangiocarcinoma cell lines treated with gramicidin. We have identified 64 upregulated genes and 201 downregulated genes in gramicidin treatment compared to the PBS control treatment. As determined by IPA analysis, metabolic dysfunction and cholesterol biosynthesis were the most significant canonical pathway perturbed by gramicidin. These results have demonstrated the critical role of metabolic signalling pathways regulated by gramicidin. Most importantly, we have found EGR4 is one of the most down-regulated genes in cholangiocarcinoma treated with gramicidin, suggesting that deregulating the expression of EGR4 is a pivotal event during tumour cell growth and could be therapeutic target for cholangiocarcinoma. EGR4 is a DNA-binding, zinc-finger transcription factor and depletion of EGR4 expression significantly suppressed growth of small cell lung cancer, which may therefore be a target for developing anticancer drugs for tumour patients [Citation18]. In patients with cholangiocarcinoma, EGR4 expression is abundance in tumour tissues as compared to tumour adjacent and normal tissues. Our analysis of TCGA data confirms that EGR4 is most highly expressed in cholangiocarcinoma patient compared with normal tissues. As EGR4 can be interacted with the specific nuclear mediator NF-κB p50 to form a complex to control transcription of genes [Citation19], and overexpression of EGR4 downregulate JNK signalling pathway [Citation20], we analysed the correlation between EGR4 and growth factors. The expression of EGR4 shows highly positive correlation with NFKB1, EGFR and PIK3CA, suggesting the critical role of EGR4 in regulating cellular growth in solid tumours, especially in cholangiocarcinoma.
In summary, we demonstrate the anti-tumour role of gramicidin in cholangiocarcinoma and present a novel target, EGR4 dependent mechanism for cholangiocarcinoma cell growth, and further describe the clinical significance of EGR4 overexpression in cholangiocarcinoma. Suppressing EGR4 by gramicidin could be a therapeutic target for preventing and curing the hepatobiliary cancer.
Disclosure statement
All authors declare that they have no competing interests.
Additional information
Funding
References
- Squadroni M, Tondulli L, Gatta G, et al. Cholangiocarcinoma [Review]. Cr Rev Oncol-Hem. 2017;116:11–31.
- Le Faouder J, Gigante E, Leger T, et al. Proteomic landscape of cholangiocarcinomas reveals three different subgroups according to their localization and the aspect of Non-Tumor liver. Prot Clin Appl. 2019;13(1):e1800128.
- Patel T. Cholangiocarcinoma–controversies and challenges [Review]. Nat Rev Gastroenterol Hepatol. 2011;8(4):189–200.
- Lafaro KJ, Cosgrove D, Geschwind JF, et al. Multidisciplinary care of patients with intrahepatic cholangiocarcinoma: updates in management [review]. Gastroent Res Pract. 2015;2015:860861.
- Morement H, Harrison R, Taylor-Robinson SD. The multidisciplinary team meeting in the UK from the patients' perspective: comments and observations from cholangiocarcinoma patients and their families. Int J Gen Med. 2017;10:305–310.
- Ong CK, Subimerb C, Pairojkul C, et al. Exome sequencing of liver fluke-associated cholangiocarcinoma [Research Support, Non-U.S. Gov't]. Nat Genet. 2012;44(6):690–693.
- Jiao Y, Pawlik TM, Anders RA, et al. Exome sequencing identifies frequent inactivating mutations in BAP1, ARID1A and PBRM1 in intrahepatic cholangiocarcinomas [Research Support, N.I.H., Extramural Research Support, Non-U.S. Gov't]. Nat Genet. 2013;45(12):1470–1473.
- Dubos RJ, Cattaneo C. Studies on a bactericidal agent extracted from a soil bacillus: Iii. preparation and activity of a protein-free fraction. J Exp Med. 1939;70(3):249–256.
- Kelkar DA, Chattopadhyay A. The gramicidin ion channel: a model membrane protein [Research Support, Non-U.S. Gov't Review]. Biochim Biophys Acta. 2007;1768(9):2011–2025.
- David JM, Owens TA, Barwe SP, et al. Gramicidin A induces metabolic dysfunction and energy depletion leading to cell death in renal cell carcinoma cells [Research Support, N.I.H., Extramural Research Support, Non-U.S. Gov't]. Mol Cancer Ther. 2013;12(11):2296–2307.
- Majd S, Yusko EC, MacBriar AD, et al. Gramicidin pores report the activity of membrane-active enzymes [Research Support, N.I.H., extramural research support, Non-U.S. Gov't]. J Amer Chem Soc. 2009;131(44):16119–16126.
- David JM, Rajasekaran AK. Gramicidin A: a new mission for an old antibiotic. J Kidney Cancer VHL. 2015;2(1):15–24.
- David JM, Owens TA, Inge LJ, et al. Gramicidin A blocks tumor growth and angiogenesis through inhibition of hypoxia-inducible factor in renal cell carcinoma [Research Support, N.I.H., Extramural Research Support, Non-U.S. Gov't]. Mol Cancer Ther. 2014;13(4):788–799.
- Li JH, Liu S, Zhou H, et al. starBase v2.0: decoding miRNA-ceRNA, miRNA-ncRNA and protein-RNA interaction networks from large-scale CLIP-Seq data [Research Support, Non-U.S. Gov't]. Nucl Acids Res. 2014;42(D1):D92–7.
- Yang JH, Li JH, Shao P, et al. starBase: a database for exploring microRNA-mRNA interaction maps from Argonaute CLIP-Seq and Degradome-Seq data [Research Support, Non-U.S. Gov't]. Nucleic Acids Res. 2011;39(suppl_1):D202–9.
- Farshidfar F, Zheng S, Gingras MC, et al. Integrative genomic analysis of cholangiocarcinoma Identifies Distinct IDH-Mutant molecular profiles [research support, N.I.H., extramural research support, Non-U.S. Gov't]. Cell Rep. 2017;18(11):2780–2794.
- Sia D, Losic B, Moeini A, et al. Massive parallel sequencing uncovers actionable FGFR2-PPHLN1 fusion and ARAF mutations in intrahepatic cholangiocarcinoma [Research Support, N.I.H., Extramural Research Support, Non-U.S. Gov't]. Nat Commun. 2015;6(1):6087.
- Matsuo T, Dat Le T, Komatsu M, et al. Early growth response 4 is involved in cell proliferation of small cell lung cancer through transcriptional activation of its downstream genes [Research Support, Non-U.S. Gov't]. PloS One. 2014;9(11):e113606.
- Wieland GD, Nehmann N, Muller D, et al. Early growth response proteins EGR-4 and EGR-3 interact with immune inflammatory mediators NF-kappaB p50 and p65 [Research Support, Non-U.S. Gov't]. J Cell Sci. 2005;118(14):3203–3212.
- Niu RN, Shang XP, Teng JF. Overexpression of Egr2 and Egr4 protects rat brains against ischemic stroke by downregulating JNK signaling pathway. Biochimie. 2018;149:62–70.