Abstract
Here we presented a novel micelle self-assembled from amphiphiles with π-conjugated moieties (OEG-DPH). The π-conjugated structural integrity of the micelles enabled stable encapsulation of Nile Red (NR, model drug). The self-assembly behaviour of the amphiphiles and the release profile of NR loaded micelles were investigated. Spherical core-shell structured NR loaded micelles with low CMC of 57 μg/mL and the efficient intracellular delivery process was monitored. This research provided a way to fabricate stable polymeric micelles and develop a practical nanocarrier for therapeutics delivery.
Introduction
Ordered states of molecules contribute to the formation of various nanoarchitectures with significant properties for wide applications [Citation1]. Ordered architectures self-assembled from amphiphilic molecules have been widely used for therapeutics delivery. Polymeric micelles, most of which were self-assembled from block copolymers, are the representative vehicles for drug delivery [Citation2,Citation3]. They have attracted much interest to chemists and biomaterials scientist [Citation4]. Polymeric micelles with short hydrophilic segments (molecular weight less than 2000) were rare studied due to the more dynamic property, which resulted difficulties in controlling architecture, morphology and delivery property [Citation5]. Fabricating dynamic stable polymeric micelles with low molecular weight hydrophilic segments is a challenge in supramolecular researches as well as practical applications.
The characteristics of polymeric micelles including driven forces and balance between hydrophilicity and hydrophobicity were the key factors to be considered in the fabrication of micelles [Citation6]. Besides hydrophobic force, many other interactions such as electronic interaction [Citation7], hydrogen bond [Citation8], host-guest [Citation9] and π-π interactions [Citation10] were utilised in constructing micellar systems not only to improve the patterns of vehicles and cargos but also to explore new strategies and methods for therapeutics delivery. Studies have demonstrated that π-π stacking interaction was one of the main driving forces for the supramolecular self-assembly in organic solvents due to the highly ordered arrangement and strong interaction within π-π conjugated moieties [Citation11]. As we know, most of anticancer drugs containing aromatic moieties [Citation12], which is understandable that the increase of interactions between anticancer drugs and the core of polymeric micelles will equip a greater thermodynamic driving force for drug loading [Citation13,Citation14]. Carbon nanomaterials were successfully fabricated carriers to absorb aromatic cargos via π-π interaction, which exhibited promising bio-applications [Citation15]. Replacement of normal hydrophobic chain with aromatic moiety in polymeric micelles would provide packed and stable interiors, which was favourable for drug encapsulation. We are interested in developing supramolecular designs for polymeric micelles with short hydrophilic chains and π-conjugated moieties to explore the π-conjugation effects on the self-assembly and drug release.
Herein, we reported a facile concept to prepare polymeric micelles with acyl-phenylhydrazone derivative (DPH), which was investigated in anion recognition and coordination chemistry [Citation16–18], as centralised aromatic pre-plane [Citation19–21], and hydrophilic oligo(ethylene glycol) (OEG) motifs as corona in the periphery to ensure the solubility balance (shown in Scheme 1(a)) [Citation22,Citation23]. The self-assembly of the micelles were investigated. Nile Red (NR) was used as a model drug and probe to explore the drug loading and release property of the micelles.
Materials and methods
Materials
Methoxypoly (ethylene glycol) (mPEG, Mw = 1000 g/mol), oligo(ethylene glycol) (OEG, Mw = 350 g/mol), Methylparaben, terephthalaldehyde, hydrazine and methylbenzenesulfonyl (TsCl) were obtained from Sigma-Aldrich Co. Nile Red and triethylamine were bought from MKL Biochem. Ltd. 100 × mycillin, Dulbecco’s modified Eagle’s medium (DMEM), foetal bovine serum (FBS) and cell counting kit-8 (CCK-8) were obtained from HyClone Inc. and used for cell experiments. NIH 3T3 fibroblasts and Hela human cervical cancer cells were obtained from Shanghai Institute of Cell Research, Chinese Academy of Sciences. All the solvents inorganic salts were purchased from Chengdu Kelong Chemical Co. (China) and purified before used.
Characterisations
The structure of products was detected by 1H NMR (Bruker AV II-400 MHz) with DMSO-d6 and D2O (1 mg/mL) as solvents, respectively. The molecular weight of the products was measured by matrix assisted laser desorption ionisation time-of-light (MALDI-TOF, Autoflex MALDI-TOF/TOF) mass spectrometry. The particle size and size distribution of OEG-DPH micelles (aqueous solution, 0.5 mg/mL) were determined by dynamic light scattering (DLS, Malvern Zetasizer Nano ZS). The measurements were repeated at different time points for monitoring the stability of NPs in water. Transmission electron microscopy (TEM, FEI Tecnai G2 F20 S-TWIN) was employed to observe the morphology of the micelles. The TEM samples were prepared by dipping copper grids with Formvar films into the freshly prepared micelles solution and dried overnight at room temperature. The sample was stained with osmium tetroxide or phosphotungstic acid (negative).
Synthesis of π-conjugated amphiphile OEG-DPH (molecule 3)
The synthetic route of OEG-DPH was shown in Scheme 1(a), the aromatic part (DPH) was obtained through a straightforward procedure via Schiff’s reaction between 4-hydroxybenzhydrazide and 1, 4-phthalaldehyde. The molecule OEG-DPH was yielded after OEG coupling.
Synthesis of OEG-OTs: p-Toluenesulfonyl chloride (3.3 g, 17.3 mmol) in CH2Cl2 (30 ml) was added dropwise in the solution of oligo (ethylene glycol) (OEG, Mn = 350, 3.3 g, 8.6 mmol) and triethylamine (4.6 ml, 34.6 mmol) in CH2Cl2 (30 ml). The reaction was stirred overnight at room temperature and then washed with 1 M HCl (3 × 50 ml) and brine (50 ml). The solution was dried over MgSO4 and the solvent was evaporated in vacuum to give crude product. The crude product was purified by column chromatography (silica, 10% methanol in CH2Cl2) to receive pure OEG-OTs as colourless oil (2.6 g, 4.9 mmol, 57%).
Synthesis of 1 and 2: Hydrazine hydrate (80%, 5 ml) was added dropwise in the solution of methyl 4-hydroxybenzoate (1.5 g, 9.8 mmol) in methanol (5 ml) in an ice bath. The mixture was stirred for 48 h at room temperature. White precipitate was isolated by centrifugation. The powder was washed with methanol and vacuum-dried overnight affording pure compound 1 (1.02 g, 6.7 mmol, 68%). The mixture of 1 (0.5 g, 3.3 mmol) and 1,4-Phthalaldehyde (0.21 g, 1.57 mmol) was dissolved in dry DMF, catalytic amount of acetic acid was added slowly at room temperature under nitrogen atmosphere. After raising the temperature to 70°C, the reaction was stirred overnight. The colour of the solution changed from colourless to pale yellow. The solvent was evaporated in vacuum and the crude product was purified by washing with warm methanol, pale yellow powder 2 was received (0.38 g, 0.94 mmol, 60%).
Synthesis of 3: A mixture of OEG-OTs (0.28 g, 0.52 mmol), 2 (0.11 g, 0.25 mmol) and K2CO3 (0.345 g, 2.5 mmol) was stirred overnight at 90 °C in dry DMF (5 ml). The reaction mixture was poured into water (15 ml) and extracted with CH2Cl2 (3 x 20 ml). The combined organic layers were washed with brine (50 ml), dried over MgSO4 and evaporated in vacuum. The crude product was purified by precipitation in warm diethyl ether and column chromatography (silica, 5% ethanol in CH2Cl2) to afford pure compound OEG-DPH (0.2 g, 0.18 mmol, 72%). MS: m/z calcd for: 1134, found: 1157.5 [M + Na]+.
Preparation and characterisation of OEG-DPH micelles
Blank OEG-DPH micelles were prepared by a self-assembly method. Briefly, 2 ml of deionised water was added in a solution of OEG-DPH (1 mg) in THF (0.1 ml) rapidly under vigorous stirring. The mixture was stirred at room temperature overnight to ensure the complete evaporation of THF. The solution (0.5 mg/mL) was stored in the refrigerator for following study.
Critical micellization concentration (CMC) of OEG-DPH micelles was determined using Nile Red as fluorescent probe. The Nile red dye was hydrophobic and exhibits strong fluorescence in the presence of intact micelles but was poorly soluble and minimally fluorescent if released into an aqueous environment when micelles destabilised [Citation21]. Different micelles dilutions (from 0.0001 to 0.5 mg/mL) were prepared from the 0.5 mg/mL stock solution. 10 μL of fresh Nile red solution in THF (6.3 × 10−4 M) was added to 1 ml of each sample under vigorous stirring and incubated overnight in dark. Fluorescence emission spectra (excitation at 550 nm) were recorded on a F-7000 spectrofluorometer (Hitachi Co. Japan). The values at 627 nm were collected for the calculation of critical micellization concentration.
Loading properties of OEG-DPH micelles
Nile Red (NR) was used to study the release behaviour of cargo-loaded OEG-DPH micelles. Aggregate dispersions of NR loaded micelles were prepared via a similar procedure as previous CMC measurement. UV measurement was performed to explore the loading process. Similarly, fluorescence analysis of Nile Red release was carried out using PBS (pH 7.4, 10 mM) instead of deionised water to form micelles (0.5 mg/mL of OEG-DPH with 3.14 × 10−5 mM NR). After incubated overnight, the samples were kept for additional 30 min at 37 °C in shaking bed, then the fluorescence spectra of the dispersions were collected. Fluorescence emission (excitation at 550 nm) of suspension was collected at each desired time point. The remaining percentage of NR was calculated by dividing readout fluorescence with initial fluorescence at 627 nm. The release experiments were conducted in triplicate.
Cellular uptake
Hela cells were cultured in DMEM medium. The mediums supplemented with 10% foetal bovine serum and 1% penicillin-streptomycin at 37 °C in a humidified atmosphere with 5% CO2. The cells were harvested with 0.02% EDTA and 0.025% trypsin and rinsed. The resulted cell suspension was used in the following experiments.
The cells (6 × 104 cells/mL) were harvested and seeded in 96-well plates with 100 μL mediums for 24 h incubation before the tests. OEG-DPH micelles in culture medium were added to the medium-removed 96-well plates with different concentrations (from 500 to 10 μg/mL) and incubated for 48 h. The culture medium was removed and the wells were rinsed with PBS (pH 7.4). MTT in PBS (5 mg/mL, 10 μL) was added to each well. After the cells were incubated for additional 4 h, the medium was removed carefully, 100 μL DMSO was added in each well. The absorbance was measured in a Thermo scientific MK3 at the wavelength of 492 nm.
Hela cells at a logarithm phase were seeded on (diameter = 35 mm) glass dishes at a cell density of 1 × 104/mL, respectively. After incubated for 24 h, NR (10 μL DMSO/1 ml DMEM) and NR-loaded micelles were dissolved and diluted in DMEM. The mixture was added into the medium-removed glass dishes. After incubated at 37 °C for 2 h, the medium was removed and the dishes were rinsed with PBS (pH 7.4). The cells were observed with confocal laser scanning microscopy (CLSM, Leica TCP SP5). NR was excited at 550 nm.
Results and discussion
The structure of OEG-DPH was confirmed by 1H NMR () and MS spectra (Scheme 1(c)). The potent solvating effects of DMSO were well known to diminish or even abolish many supramolecular interactions [Citation24,Citation25] thus, the pattern in showed the free dissolution of OEG-DPH, where protons appeared in narrow and sharp peaks belonging to either aromatic or OEG parts. The presence of aromatic moiety was known to facilitate the self-assembly of molecules via π-π interaction in water [Citation26]. Upon dissolution in D2O, the dispersion of OEG-DPH was markedly different. It was known that, when amphiphiles were analysed by 1H NMR measurement in D2O, the signal of hydrophobic segment showed a strong attenuation or even completely disappeared due to the formation of core-corona like aggregates in which the hydrophobic part was buried [Citation27,Citation28]. Similarly, as shown in , the peaks assigned to the aromatic segments decreased visibly and became broad, while the protons in OEG chain exhibited significant and sharp shape. This result suggested the core-shell type micelles were formed in aqueous condition.
Figure 1. 1H NMR spectra of OEG-DPH in DMSO-d6 (a) and D2O (b) for the self-assembly study. The insert graph was the magnified part from 8.2–6.8 ppm. XRD patterns of the aromatic part DPH, mPEG1K, and OEG-DPH micelles (c).
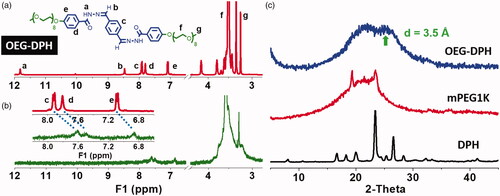
Meanwhile, we noticed signal shifting in the 1H NMR spectrum when the solvent DMSO-d6 was replaced by D2O. Specifically, the signal of proton H (f) in OEG unit shifted to downfield position (Δδ = 0.13 ppm), whereas the aromatic protons H (c, d, e) neighboured to the OEG unit shifted to upfield (Δδ = 0.16–0.2 ppm). Normally, the increase of solvent polarity leads to the deshielding effect of fully soluble parts, which will result in downfield shifting. However, the striking evidence of intermolecular π-π stacking within the aromatic segment appearing as shielding effect led to upfield shifting to protons H (c, d, e) [Citation29,Citation30]. The FTIR spectra of OEG-DPH treated with DMSO and H2O (Figure S1) indicated that the peak assigned to the skeletal vibration of aromatic C=C bonds (1575 cm−1) was greatly decreased after the self-assembly in water, that was attributed to the formation of π-π interaction between benzene rings [Citation31]. According to the XRD pattern in , a peak at 2θ = 25.4° with a d value of 3.5 Å appeared and it was a characteristic peak for the aromatic-aromatic interaction [Citation32,Citation33].
In the free molecular state in DMSO, OEG-DPH displayed significant absorption of λmax at 350 nm in UV-vis spectrum. The absorption peak was blue-shifted to 331 nm () after the self-assemblies were formed in water. This hypsochromic absorption shift was a typical indication for the H-aggregation of linear chromophores [Citation34], implying the strong interactions between the π-conjugated planes, which provided robust driven force for micellization. Dynamic light scattering (DLS) measurement was used to investigate the stability of the micelles (). The mean diameter of micelles was stable and kept at about 30 nm for even 19 days standing. The high stability was ascribed to the steady and compact π-conjugated core of micelles, which also maintained the same size of micelles in PBS (Figure S2). The micelles were monodispersed with a narrow size distribution in DLS measurement. The colloid solution was homogeneous and exhibited bright Tyndall effect (). Transmission electron microscopy (TEM) images displayed that the micelles were spherical particles of approximately 30 nm in diameter with the concentration of 0.5 mg/mL in aqueous solution (). The TEM photographs displayed clear core-shell structure of the micelles. The size of OEG-DPH micelles in TEM was consistent with the DLS result. The micelles experienced a certain degree of aggregation in the TEM images. It was because of the low melting point of OEG350, the attack of electrons on samples increased the temperature to melt OEG shell layer.
Figure 2. UV-vis spectra of OEG-DPH with the concentration of 100 μg/mL in DMSO and water (a). Plots of the mean micelle size in different standing time (b). Insert: graph of the size distribution in DLS measurement, photos of the micelles in aqueous solution with Tyndall effect. TEM image of OEG-DPH micelles (c). Insert: magnified core-shell structure image of the micelles.
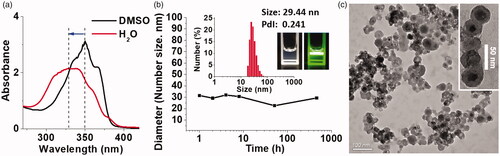
The quantitative micellization characterisation was followed by measuring the CMC with NR as a fluorescent probe. NR is a hydrophobic dye. It could be used as an indicator for micellization investigation to generate fluorescence when it was sequestered inside the hydrophobic pocket of micelles [Citation35]. As shown in , the maxima of fluorescence emission shifted from about 645 nm to 627 nm in the process of loading, indicating the transfer of NR from a hydrophilic environment to the hydrophobic core of micelles [Citation36]. The values at 627 nm were collected to calculate CMC, the calculated CMC was 57 μg/mL (). Additionally, the CMC value was rechecked by DLS measurement, which showed the existence of micelles at the concentration of 50 μg/mL with a low “Count rate” (testing parameter, monitoring the effective aggregation behaviour) [Citation37]. This result implied that the OEG-DPH micelles were formed in a low concentration and could maintain their stability in dilute condition.
Figure 3. Plot of fluorescence intensity (627 nm) of NR vs concentration of OEG-DPH with the excitation wavelength at 550 nm (a). Inserted photograph shows the solutions of NR with OEG-DPH; Fluorescence emission spectra (b) and UV-vis spectra (c) of NR dispersing in the OEG-DPH solutions with different concentrations. Plot of intensity ratio of A578 to A538 vs different concentration of OEG-DPH (d). The bottom scheme showed the simulation of NR loading in micelles (I: 0.01–0.05 mg/mL; II: 0.05 mg/mL; III: 0.05–0.3 mg/mL).
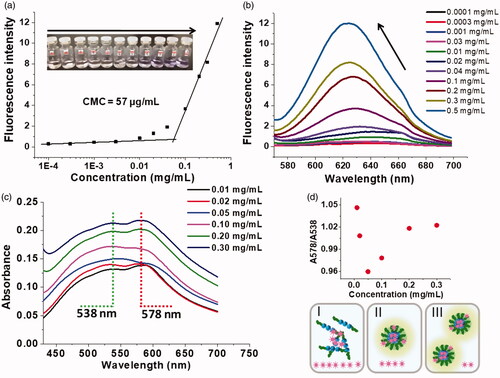
The NR loading process was also supervised by UV-vis measurement. Interestingly, we found two obvious peaks in NR/OEG-DPH micelle solution (), which was different from the pattern in free NR solution. As a solvatochromic dye, NR could be used to probe the polarity of microenvironment. This behaviour of NR revealed that the absorption and emission maxima strongly depended on the polarity of the environment (solvent) [Citation38]. In methanol, NR was dissolved and showed a narrow absorption band at 554 nm, while no evident signal was observed in water due to its hydrophobicity. We chose mPEG1k aqueous solution with the concentration of 0.5 mg/mL as a control to probe the condition and aggregation of NR, the suspension showed a broad and very low absorbance maximised at 535–540 nm (shown in Figure S3). The unusual hypsochromic shift originated from H-aggregation demonstrated the absorption of NR molecules into PEG clew in polar environment. This signal agreed with the absorbance of NR/micelles solution at 538 nm. Actually, in our previous CMC study, we found that the emission intensity began to raise in hypsochromic region at low micelle concentration, that was also the evidence for H-aggregates formation [Citation39]. As papers reported [Citation40,Citation41], the red shift at 578 nm in was ascribed to the π-π interactions between aromatic guest molecule and aromatic structure of carriers. Nile Red, in particular, exhibited an absorbance at more bathochromic region in the hydrophobic environment. We therefore assumed that in the presence of micelle solution, NR tended to form H-aggregates sticking in the OEG shell layer while other NR molecules were encapsulated in the hydrophobic core and experienced strong π-π interactions with the aromatic structure of the micelles, it was in accord with the maximum absorption at 538 and 578 nm, respectively. Moreover, we found that the intensity ratio of these two peaks was slightly changed when NR was added in the solutions with different micelle concentrations (from 0.01 to 0.3 mg/mL). As shown in , we divided the pattern into three parts (I, II, III). It was understandable that II (0.05 mg/mL) was the essential change to be considered as the critical micellization point, which agreed with the CMC test. With the concentration increase, more and more NR molecules tended to be encapsulated into the core of micelles, thus, the absorbance at 578 nm was enhanced, it was reflected in part III. The decrease in the ratio of 578 nm to 538 nm could be explained as below. NR molecules were apt to form H-aggregates in the micelle solution below CMC, and only the NR molecules incorporated with the aromatic moieties in OEG-DPH amphiphile could be dissolved. This was the essential difference between mPEG1k solution and free OEG-DPH amphiphile solution, in which we could detect two signals originated from NR molecules in different environments. The freer OEG-DPH molecules as hydrotrope were fixed, the more H-aggregates were dissolved, leading to the totally increase of absorbance, especially at 538 nm. This intermolecular interaction was not strong enough to self-assemble at such low concentration, the incorporation between OEG-DPH amphiphile and NR H-aggregates just facilitated the absorbance on the surface. This might be the main reason of weak absorbance increase at 578 nm in part I. The situation began to change after the micellization.
The release behaviour of cargo-loaded micelles, which would reflect the combining capacity and delivery application to guest hydrophobic molecules, was carried out using NR as a model drug. showed the normalised fluorescence of NR released from NR-loaded micelles in PBS (pH 7.4) at 37 °C. The blank OEG-DPH micelles solution would not disturb the results of testing as lack of emission intensity at 627 nm. As time went on, the fluorescence decreased gently. Nearly 40% of the dye molecules quenched from NR loaded micelles in the first 24 h, and the mostly quenching needed more than 120 h. These cargo-loaded micelles exhibited milder and more sustained release than that of typical polymeric delivery system at the same molecular weight level [Citation42]. This release behaviour was attributed to the combination of π-π and hydrophobic interactions within the micelles, which enhanced the interactions between drug and carriers to avoid the rapid diffusion of the cargo. It was clearly visible in the emission spectra () that a slight blue shift of maxima from 627 nm to 621 nm appeared in the process of release. As described previously, the solvatochromic characteristic of NR was usually utilised to monitor the microenvironment it stayed. The maximum of fluorescence emission at 621 nm was considered as the expression of NR in the centre of micelle core after the prior surrounding NR release. This phenomenon exhibited the outside-in diffusion process of the release of NR from the micelles.
Figure 4. Fluorescence of Nile Red upon release from NR/OEG-DPH micelles in PBS (pH 7.4) (a). Inserted photos showed the loading process. Ex = 550 nm. Fluorescence emission spectra of NR/OEG-DPH with the time increase (b). Confocal microscopy images of Hela cells treated with Nile Red self-aggregates (10 μL DMSO/mL DMEM) and NR/OEG-DPH (0.1 mg/mL) for 2 h (c). Nile red was excited at 550 nm. The scale bar was 10 μm.
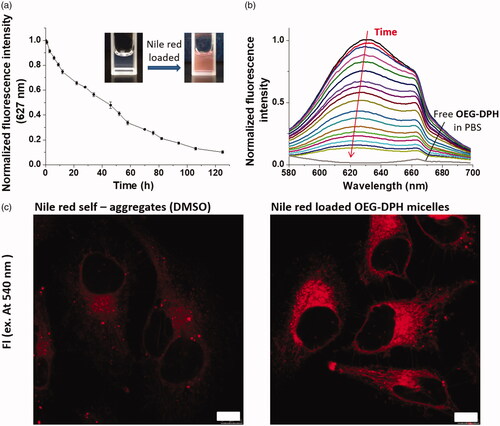
In the subsequent experiment, the preliminary bio-application of NR loaded OEG-DPH micelles were carried out for cellular uptake. Nile Red is a dye to stain intracellular lipophilic regions [Citation33]. As shown in , NR diffused into the cytoplasm and exhibited clear red fluorescence with partial precipitate dots due to the poor solubility. Importantly, it was found that the red fluorescence of NR was significantly increased after encapsulated in micelles, suggesting the enhanced solubility and intracellular delivery of NR. The efficient cellular uptake and good non-cytotoxicity (Figure S4) of the micelles were provided their potential bio-applications.
Conclusions
In conclusion, we have rationally designed a small amphiphile with π-conjugated moiety and short PEG chain. The self-assembly behaviour of the amphiphiles in aqueous solution was studied. The amphiphiles associated into core-shell structured micelles via π-π and hydrophobic interactions with low CMC of 57 μg/mL. Nile red was successfully encapsulated in the micelles, the loading process was monitored by fluorescence and UV-vis measurements. The release profile showed that NR loaded micelles exhibited sustained NR release due to the enhancement of interaction between NR and the π-conjugated core, which performed efficient intracellular delivery. This work provided a strategy to fabricate stable polymeric micelles via the self-assembly driven by the combination of hydrophobic and π-π interactions. It developed a novel practical nanocarrier for therapeutics delivery.
Supplemental Material
Download PDF (167.8 KB)Disclosure statement
The authors report no conflicts of interest. The authors alone are responsible for the content and writing of this article.
Additional information
Funding
References
- Lee S, Oh S, Lee J, et al. Stimulus-responsive azobenzene supramolecules: fibers, gels, and hollow spheres. Langmuir. 2013;29(19):5869–5877.
- Dai J, Han S, Ju F, et al. Preparation and evaluation of tumour microenvironment response multistage nanoparticles for epirubicin delivery and deep tumour penetration. Artif Cells Nanomed Biotechnol. 2018;46(sup2):860–873.
- Mai Y, Eisenberg A. Self-assembly of block copolymers. Chem Soc Rev. 2012;41(18):5969–5985.
- Ge R, Cao J, Chi J, et al. NIR-guided dendritic nanoplatform for improving antitumor efficacy by combining chemo-phototherapy. IJN. 2019;14:4931–4947.
- Wang H, Yang C, Wang L, et al. Self-assembled nanospheres as a novel delivery system for taxol: a molecular hydrogel with nanosphere morphology. Chem Commun. 2011;47(15):4439–4441.
- Yoo J, Doshi N, Mitragotri S. Adaptive micro and nanoparticles: temporal control over carrier properties to facilitate drug delivery. Adv Drug Deliv Rev. 2011;63(14–15):1247–1256.
- Wang C, Chen Q, Wang Z, et al. An enzyme-responsive polymeric superamphiphile. Angew Chem Int Ed. 2010;49(46):8612–8615.
- Leenders CMA, Albertazzi L, Mes T, et al. Supramolecular polymerization in water harnessing both hydrophobic effects and hydrogen bond formation. Chem Commun. 2013;49(19):1963–1965.
- Tyagi R, Malhotra S, Thünemann AF, et al. Investigations of host–guest interactions with shape-persistent nonionic dendritic micelles. J Phys Chem C. 2013;117(23):12307–12317.
- Liang Y, Huo Q, Lu W, et al. Fluorescence resonance energy transfer visualization of molecular delivery from polymeric micelles. J Biomed Nanotechnol. 2018;14(7):1308–1316.
- Song B, Wang Z, Chen S, et al. The introduction of π–π stacking moieties for fabricating stable micellar structure: formation and dynamics of disklike micelles. Angew Chem. 2005;117(30):4809–4813.
- D’Emanuele A, Attwood D. Dendrimer–drug interactions. Adv Drug Deliv Rev. 2005;57:2147–2162.
- Bickerton S, Jiwpanich S, Thayumanavan S. Interconnected roles of scaffold hydrophobicity, drug loading, and encapsulation stability in polymeric nanocarriers. Mol Pharmaceutics. 2012;9(12):3569–3578.
- Dong X, Guo X, Liu G, et al. When self-assembly meets topology: an enhanced micelle stability. Chem Commun. 2017;53(27):3822–3825.
- Huo Q, Liang Y, Lu W, et al. Integrated metalloproteinase, pH and glutathione responsive prodrug-based nanomedicine for efficient target chemotherapy. J Biomed Nanotechnol. 2019;15(8):1673–1687.
- Rajamalli P, Prasad E. Tunable morphology and mesophase formation by naphthalene-containing poly(aryl ether) dendron-based low-molecular-weight fluorescent gels. Langmuir. 2013;29(5):1609–1617.
- Rajamalli P, Prasad E. Low molecular weight fluorescent organogel for fluoride ion detection. Org Lett. 2011;13(14):3714–3717.
- Agarwal C, Prasad E. Detection of Cu(ii) and NO by ‘on-off’ aggregation in poly(aryl ether) dendron derivatives. New J Chem. 2012;36(9):1859–1865.
- von Delius M, Geertsema EM, Leigh DA, et al. Synthesis and solid-state structure of a hydrazone-disulfide macrocycle and its dynamic covalent ring-opening under acidic and basic conditions. Org Biomol Chem. 2010;8(20):4617–4624.
- Charkoudian LK, Pham DM, Franz KJ. A pro-chelator triggered by hydrogen peroxide inhibits iron-promoted hydroxyl radical formation. J Am Chem Soc. 2006;128(38):12424–12425.
- Kunitake T, Okahata Y, Shimomura M, et al. Formation of stable bilayer assemblies in water from single-chain amphiphiles. Relationship between the amphiphile structure and the aggregate morphology. J Am Chem Soc. 1981;103(18):5401–5413.
- Shen Y, Jin E, Zhang B, et al. Prodrugs forming high drug loading multifunctional nanocapsules for intracellular cancer drug delivery. J Am Chem Soc. 2010;132(12):4259–4265.
- Wang J, Sun X, Mao W, et al. Tumor redox heterogeneity-responsive prodrug nanocapsules for cancer chemotherapy. Adv Mater. 2013;25(27):3670–3676.
- Bolla MA, Tiburcio J, Loeb SJ. Characterization of a slippage stopper for the 1,2-bis(pyridinium)ethane–[24]crown-8 ether [2]pseudorotaxane motif. Tetrahedron. 2008;64(36):8423–8427.
- Greenland BW, Bird MB, Burattini S, et al. Mutual binding of polymer end-groups by complementary [small pi]-[small pi]-stacking: a molecular “Roman Handshake. Chem Commun. 2013;49(5):454–456.
- Kar T, Debnath S, Das D, et al. Organogelation and hydrogelation of low-molecular-weight amphiphilic dipeptides: pH responsiveness in phase-selective gelation and dye removal. Langmuir. 2009;25(15):8639–8648.
- Li Z, Zhang Z, Liu KL, et al. Biodegradable hyperbranched amphiphilic polyurethane multiblock copolymers consisting of poly(propylene glycol), poly(ethylene glycol), and polycaprolactone as in situ thermogels. Biomacromolecules. 2012;13(12):3977–3989.
- Lundy BB, Convertine A, Miteva M, et al. Neutral polymeric micelles for RNA delivery. Bioconjugate Chem. 2013;24(3):398–407.
- Rajamalli P, Atta S, Maity S, et al. Supramolecular design for two-component hydrogels with intrinsic emission in the visible region. Chem Commun. 2013;49(17):1744–1746.
- Wang XJ, Xing LB, Wang F, et al. Multistimuli responsive micelles formed by a tetrathiafulvalene-functionalized amphiphile. Langmuir. 2011;27(14):8665–8671.
- Xu J, Wang L, Zhu Y. Decontamination of bisphenol A from aqueous solution by graphene adsorption. Langmuir. 2012;28(22):8418–8425.
- Nanda J, Biswas A, Banerjee A. Single amino acid based thixotropic hydrogel formation and pH-dependent morphological change of gel nanofibers. Soft Matter. 2013;9(16):4198–4208.
- Shao H, Nguyen T, Romano NC, et al. Self-assembly of 1-D n-type nanostructures based on naphthalene diimide-appended dipeptides. J Am Chem Soc. 2009;131(45):16374–16376.
- Shin S, Gihm SH, Park CR, et al. Water-soluble fluorinated and PEGylated cyanostilbene derivative: an amphiphilic building block forming self-assembled organic nanorods with enhanced fluorescence emission. Chem Mater. 2013;25(16):3288–3295.
- Klaikherd A, Nagamani C, Thayumanavan S. Multi-stimuli sensitive amphiphilic block copolymer assemblies. J Am Chem Soc. 2009;131(13):4830–4838.
- Bigot J, Charleux B, Cooke G, et al. Tetrathiafulvalene end-functionalized poly(N-isopropylacrylamide): a new class of amphiphilic polymer for the creation of multistimuli responsive micelles. J Am Chem Soc. 2010;132(31):10796–10801.
- Wang C, Wang G, Wang Z, et al. A pH-responsive superamphiphile based on dynamic covalent bonds. Chem Eur J. 2011;17(12):3322–3325.
- Bhatia S, Mohr A, Mathur D, et al. Biocatalytic route to sugar-PEG-based polymers for drug delivery applications. Biomacromolecules. 2011;12(10):3487–3498.
- Cheng Y, Hao J, Lee LA, et al. Thermally controlled release of anticancer drug from self-assembled γ-substituted amphiphilic poly(ε-caprolactone) micellar nanoparticles. Biomacromolecules. 2012;13(7):2163–2173.
- Yang X, Zhang X, Liu Z, et al. High-efficiency loading and controlled release of doxorubicin hydrochloride on graphene oxide. J Phys Chem C. 2008;112(45):17554–17558.
- Lai Y, Lei Y, Xu X, et al. Polymeric micelles with [small pi]-[small pi] conjugated cinnamic acid as lipophilic moieties for doxorubicin delivery. J Mater Chem B. 2013;1(34):4289–4296.
- Cui C, Xue Y, Wu M, et al. Cellular uptake, intracellular trafficking, and antitumor efficacy of doxorubicin-loaded reduction-sensitive micelles. Biomaterials. 2013;34(15):3858–3869.