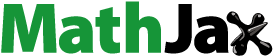
Abstract
Background
The ever-increasing resistance to antimicrobial agents among bacteria associated with nosocomial infections indicate the necessity of new antimicrobial therapy. The nanoparticles are considered as new drug delivery systems to increase the efficiency and decrease the unfavourable effects of the antimicrobial agents.
Methods
Herein we report the preparation and characterization of mesoporous silica nanoparticles (MSNs) loaded with meropenem against carbapenem-resistant Enterobacteriaceae. The antimicrobial effect of meropenem-loaded MSNs was determined against Enterobacteriaceae using the minimum inhibitory (MIC) method. The biocompatibility of meropenem-loaded MSNs was studied by the impact on the haemolysis and sedimentation rates of human red blood cells (HRBCs). Cytotoxicity of the meropenem-loaded MSNs was studied by the MTT test (hBM-MSC cell viability).
Results
The meropenem-loaded MSNs have shown antibacterial activity on all isolates at different MIC values lower than MICs of meropenem. Free MSNs did not show any significant antibacterial effect. Meropenem-loaded MSNs have no significant effect on haemolysis and ESR of HRBCs. The viability of hBM-MSC cells treated with serial concentrations of meropenem-loaded MSNs was 92–100%.
Conclusion
Due to the desirable biocompatibility, low cytotoxicity and the improved antibacterial effect, MSNs can be considered as a promising drug delivery system for meropenem as a potential antimicrobial agent.
Introduction
Antibiotic-resistant pathogens are the cause of different clinical challenges worldwide [Citation1,Citation2]. The emergence and the spread of the antimicrobial-resistant bacteria causing healthcare-associated and community-acquired infections which are related to the levels of antimicrobial agents administration [Citation3]. Infections due to multidrug-resistant Enterobacteriaceae are an increasing problem worldwide [Citation4]. Enterobacteriaceae are an opportunist group of bacteria that cause different infections such as urinary tract infections, soft tissue infections and sepsis especially in health care centres [Citation5–7]. Recently the emergence of resistant Enterobacteriaceae to wide-spectrum antibiotics limited clinicians to choice of appropriate antibiotic therapy. Carbapenems, including imipenem, meropenem, doripenem, and ertapenem, are the most common option for the treatment of infections caused by these resistant pathogens [Citation8]. Carbapenems are wide-spectrum β-lactam antibiotics with strong effects on severe/complicated infections caused by resistant bacteria, which are considered as the last reliable option for life-threatening nosocomial infections [Citation9]. However, the emergence of Enterobacteriaceae with resistance to carbapenems limited their effectivity and is considered as a remarkable menace to global health [Citation10]. The outcomes of infections caused by carbapenems resistant pathogens are poor and few options remain for the treatment of these virulent organisms. Therefore, novel options other than antibiotics are essential for reducing the risk of antibiotic-resistant bacteria. Recently, nanoparticles as drug delivery systems have gained considerable attention in the field of antibacterial therapy [Citation11]. Mesoporous silica nanoparticles (MSNs) are of the most common noncarriers used for this aim [Citation12,Citation13]. MSNs have several characteristics, which make them favourable drug delivery systems. Some of these characteristics are appropriate surface area, biocompatibility, adjustable pore size, high thermal and mechanical stability, versatile surface chemistry allowing chemical functionalization, and low cost of production and ease of large scale production [Citation14–17]. The aims of this study were to prepare meropenem-loaded MSNs and to determine its physicochemical, biocompatibility and cytotoxicity properties together with its antimicrobial effects on carbapenem-resistant Enterobacteriaceae.
Materials
Human bone marrow stem cells (hBM-MSCs) were purchased from the Pasteur Institute of Iran. Foetal bovine serum (FBS), Dulbecco’s modified Eagle’s medium (DMEM), trypsin, penicillin G and streptomycin were achieved from Gibco (Life Technologies, Carlsbad, CA, USA). Meropenem, Dimethylsulphoxide (DMSO), MTT (3-[4,5-dimethyl-thiazol-2-yl]-2,5-diphenyl-tetrazoliumbromide were bought from Sigma-Aldrich (Darmstadt, Germany). Cation adjusted Mueller-Hinton broth (CAMHB) and Tryptic Soy Broth (TSB) were purchased from Merck Co. (Darmstadt, Germany).
Methods
Synthesis of MSNs
The synthesis of MSNs carried out as previously demonstrated by Hanafi-Bojd et al. [Citation18]. First, NaOH (2 M) and deionized water were mixed and cetyl trimethyl ammonium bromide (CTAB) was dissolved in this mixture under stirring for 30 min at 80 °C. Then, TEOS was added to CTAB solution and stirring continued for 20 min at 80 °C. At this stage, 3-(trihydroxysilyl) propyl methylphosphonate was added to the solution and was stirred at 80 °C for 2 h. After this time, the solution was cooled down to reach room temperature. For CTAB removal, the synthesized MSNs were refluxed in acidic methanol for 48 h.
Meropenem-loaded MSNs
For meropenem loading on MSNs nanoparticles, 10 mg of MSNs was added to 5 mL of meropenem methanol/water solution (2 mg/mL). After 24 h stirring at room temperature, the solution was centrifuged and was washed with deionized water. For determination of meropenem loading efficiency, 1 mg of the meropenem- loaded MSNs nanoparticle was suspended (1 mg) in 2 mL phosphate-buffered saline (PBS, pH 7.4). Samples were incubated in a shaking incubator at 200 rpm at 37 °C and the amount of meropenem was measured in the supernatant at 297 nm using a UV–visible spectrophotometer after removing particles by centrifugation (19000 × g for 20 min). Then, using the previously prepared calibration standard curve (10–50 µg/mL), the drug loading and entrapment efficiency of meropenem -loaded MSNs were determined using the following equations [Citation19,Citation20]:
Particle size, morphology and surface charge
The mean diameter of meropenem-loaded MSNs and MSNs nanoparticles were determined by the Dynamic Light Scattering (DLS) method by a Zetasizer Nano-ZS equipment (Malvern Instruments Ltd., UK). Before detection of size, an aqueous solution of meropenem-loaded MSNs and MSNs were dispersed by sonication (Power 500 W, amplitude 20%, reaction time: 30 min, pulse on: 2 s and pulse off: 2 s in an ice-bath) at 25 ± 1 C. SEM and TEM microscopy were used to assess the morphology and structure of the prepared nanoparticles. SEM analysis was performed by a Scanning Electron Microscope (SEM, TESCAN, Warrendale, PA). The dry powder of the nanoparticles was fixed via an adhesive tape and then sputter-coated with gold. For TEM analysis (JEM-2100F; JEOL, Tokyo, Japan), samples were prepared by dropping a solution of nanoparticles in deionized water on a carbon-coated copper TEM grid. Surface charge of the nanoparticles was determined by zeta potential assessment using zeta-sizer (Malvern, UK) at 25 °C. Briefly, the fresh aqueous suspension of nanoparticles was diluted and perfused into the capillary cell of zeta-sizer.
Release of meropenem from MSNs
Meropenem loaded-MSNs were suspended (10 mg) in 100 mL phosphate-buffered saline (PBS, pH 7.4). Sample was incubated in a shaking incubator at 100 rpm at 37 °C. At eight time points (0, 1, 2, 4, 6, 8, 10 and 12 days), 2 mL of the medium was collected and then replaced with the same volume of PBS. The amount of meropenem was then measured by ultraviolet spectrophotometry at 297 nm (n = 3) for each point after removing particles by centrifugation (19000 × g for 20 min).
Bacterial isolates
Ten non-duplicated clinical carbapenem-resistant isolates of Enterobacteriaceae were used in this study. The identification of Enterobacteriaceae was carried out by Gram staining and standard bacteriological methods. The microbroth dilution test was performed to determine of meropenem inhibitory concentration (MIC) in the Cation-adjusted Mueller-Hinton broth (CAMHB) according to the clinical and laboratory standards institute (CLSI) guideline [Citation21].
The antibacterial activity of meropenem-loaded MSNs
The antibacterial effects of meropenem, MSNs and meropenem-loaded MSNs were determined by the MIC (the broth microdilution technique) determination in CAMHB. The broth microdilution was performed according to the CLSI guidelines [Citation21]. The MIC was defined as the lowest level of a compound that completely inhibited the growth of an isolate as detected by the unaided eye.
Biocompatibility of meropenem-loaded MSNs
Haemolysis assay. The biocompatibility of meropenem-loaded MSNs was studied by haemolysis assay on human red blood cells (HRBCs). Firstly, 2 mL of fresh blood was collected into the haematocrit tube. Then, to collect the HRBCs, the blood was centrifuged at 3000 rpm for 5 min and then washed three times with PBS (pH: 7.4). The serial concentrations of meropenem-loaded MSNs was added to tubes contained 0.5 mL of 10 times diluted HRBCs.
The tubes were incubated at 37 °C for 3 h. PBS and water were used as negative and positive controls of haemolysis, respectively. The morphology of HRBCs was studied using optical microscopy with a magnification of ×100. All tubes were centrifuged at 5000 rpm for 5 min and 200 μl of each tube supernatant was transferred into the wells of a microplate. To determine the rate of haemolysis, released haemoglobin by each concentration of meropenem-loaded MSNs was measured using ELISA plate-reader at 540 nm. The following equation was used to calculate the haemolysis rate:
where OD (s), OD (–) and OD (+) are absorbance of the samples, negative control and the positive control supernatants, respectively [Citation22].
Erythrocyte sedimentation rate (ESR) assay
The effect of meropenem-loaded MSNs on human blood samples was studied by erythrocyte sedimentation rate (ESR). Firstly, 128 μg/mL of meropenem-loaded MSNs was added to blood samples in a western green tube containing 3.2% sodium citrate and was left at room temperature for 60 min. A western green tube containing 0.25 mL of PBS (pH 7.4) was considered as a negative control. Then, the level of the erythrocyte column was measured as the erythrocyte sedimentation rate in mm/h [Citation23].
In vitro cytotoxic effects of meropenem-loaded MSNs
Cytotoxicity effect of the meropenem-loaded MSNs on hBM-MSC cells was determined by the MTT assay (3-(4, 5-dimethylthiazol-2-yl)-2,5-diphenyl tetrazolium bromide). Firstly, hBM-MSC cells were transferred into 96-well microtiter plates at a density of 10000 cells/well. After 24 h incubation, the culture medium was removed from wells and 100 mL/well of meropenem-loaded MSNs serial dilutions (1–128 μg/mL) in antibiotic-free RPMI was added to wells. The microtiter plates were incubated at 37 °C for 24 h. Then, 20 μL of MTT was transferred to each well to reach a final concentration of 0.5 mg/mL and incubated for 4 h. After, removal of unreacted MTT, 200 μL/well of dimethylsulphoxide (DMSO) was added for dissolving the stain. The absorbance of the solubilized stain was determined at 570 and 690 nm. All stages were performed in triplicate. The cell viability was calculated by the following equation [Citation24]:
Statistical analysis
Data were analysed using the one-way Analysis of Variation (ANOVA) in the Statistical Package for the Social Sciences (SPSS) software version 20. Values of p < .05 were considered significant.
Results and discussion
Characterization
The increasing frequency of resistance to antimicrobial agents and the necessity of inhibition in the first steps of microbial colonization indicate the requirement to introduce the new therapeutic options for eradication and management of infections. MSNs and other mesoporous silica-based nanomaterials have commonly been investigated in antimicrobial studies loaded with different agents and biocides. It is important to notice that the nanoparticle size and morphology and thus, the interaction of the particle with the bacterial surface have significant effects on its antimicrobial activities [Citation25].
The size distributions of the MSNs nanoparticle and meropenem-loaded MSNs are shown in . The average diameters of the blank MSNs and meropenem-loaded MSN particles were 68.43 ± 1.2 nm and 110.6 ± 0.87 nm, respectively. The porous structure and size of MSNs were characterized using TEM. In this study, the zeta potential of free MSNs and meropenem loading MSNs were −12 mV and −11.7 mV, respectively (c and 1d). Zeta potential values provide information related to the charge of prepared nanomaterials. Zeta potential is a helpful parameter for anticipating stability and also interaction with biological surfaces [Citation20]. It has demonstrated that a negative zeta potential has a desirable effect on the interaction of nanoparticles with some bacteria and improves the biological activities [Citation26,Citation27].
Figure 1. Particle size distribution (PSD) and the zeta potential (ZP) amounts of the prepared nanoparticles: (a) PSD of free MSNs, (b) PSD of meropenem-loaded MSNs, (c) ZP of free MSNs and (d) ZP of meropenem-loaded MSNs.
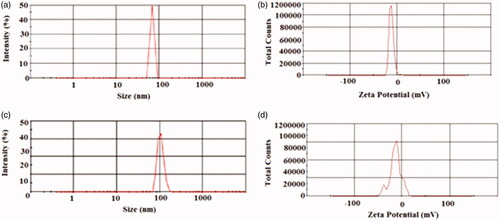
The mesoporous structure of MSNs is shown in and . The SEM results showed the rod-shaped morphology for the prepared nanoparticles. TEM images confirmed the mesoporous structure as well as the rod-shaped morphology for the prepared nanoparticles. In addition, we found the diameter detected by electronic microscopes (EM) was larger than DLS. Indeed, these are two completely different approaches based on different principle, EM is intuitive technique by detecting nanoparticles under electronic microscopes, while DLS used the principle of dynamic light scattering to sense diffusion coefficient of nanoparticles. Besides, owing to the production of the hydration film while nanoparticles were dispersed in dispersion medium, so the size detected by DLS was different from SEM [Citation28]. The reports have shown that rod- or filament-shaped nanoparticles had a longer circulation half-life in vivo compared with spherical particles. Besides, it was reported that long-rod nanoparticles have minimal uptake by the RES and long blood circulation half-lives [Citation28–30]. Zhao et al. in an ex vivo optical imaging study reported a longer residence time in the gastrointestinal tracts of animal models for rod nanoparticles than spherical nanoparticles. According to in vivo biodistribution results, the liver, and kidney mostly took up the entire orally-administered MSNs. Long rod nanoparticles were showed a high potential to overcoming fast clearance via reticuloendothelial system as well as a longer presence in the blood flow in comparison to short rod nanoparticles and spherical nanoparticles. The spherical nanoparticles indicated a faster renal clearance than rod nanoparticles [Citation28]. According to the results of in vitro antibacterial effects, the rod-shaped nanoparticles are more effective against microbial pathogens than cubic shaped [Citation31].
The drug loading and entrapment efficiency are the characteristics of a drug delivery system which are important to estimate the usability of carriers [Citation32]. The drug loading and entrapment efficiency of meropenem on MSNs were 18.4% and 38.9%, respectively. The drug loading efficiency by MSNs were reported 11–37% for polymyxin B [Citation13], 11.7–18.7% for tetracycline [Citation12], 18.3% for ampicillin [Citation33] and 31% for gentamicin [Citation34]. Differences in loading efficiency are most likely because of the physicochemical characterization of noncarriers and cargo [Citation12]. Meropenem loaded-MSNs () showed approximately 40% release of meropenem during the first day, and much less during the subsequent day (20%). Furthermore, meropenem showed sustained-release profile until the 12th day. We propose that the release of the adsorbed drug molecules in the first day leads to a fast releasing profile and the stronger electrostatic interactions between the meropenem and MSNs is the reason for the sustained release profile until the 12th day [Citation23]. Braun et al., studied the correlation between loading efficiency and interaction of MSNs with bacteria. Their study showed that MSNs with high loading of antibiotic (but with weak interaction of drug and MSNs) did not show good interaction with the bacteria membrane. However, MSNs with a low but strong interaction of drug and MSNs was able to interact with the bacteria membrane in a slow-release profile [Citation35].
Antibacterial effects
The meropenem-loaded MSNs have shown an inhibitory effect on all tested bacterial isolates with various inhibitory concentrations. The MICs of meropenem-loaded MSNs were lower than the MICs of meropenem against all tested bacteria (). The MIC of meropenem-loaded MSNs was lower in the isolates with the low level of meropenem MIC. The MIC ranges of meropenem and meropenem-loaded MSNs were 4–64 μg/mL and 1–16 μg/mL, respectively. Free MSNs did not show a significant antibacterial effect. Membrane interactions of MSNs have been reported to be significantly dependent on nanoparticle properties [Citation35]. The suggested loading of meropenem in MSNs could affect cell membrane transport mechanisms in microorganisms to increase the antibacterial properties. Such possessions of meropenem can be associated with altered permeability in the plasma membrane and interaction with different agents inside the microorganism [Citation36]. The studies also have specified that the antimicrobial loaded NPs may interact with bacteria via two probable mechanisms: (1) it may fuse with bacteria’s cell wall or membrane and release the antimicrobial agent within them; (2) it also may adsorb to the cell wall to act as a depot to continuously release antimicrobial agent [Citation37].
Figure 5. Haemolysis of human red blood cells (HRBCs) in the presence of different concentrations (1–128 μg/mL) of meropenem-loaded MSNs.
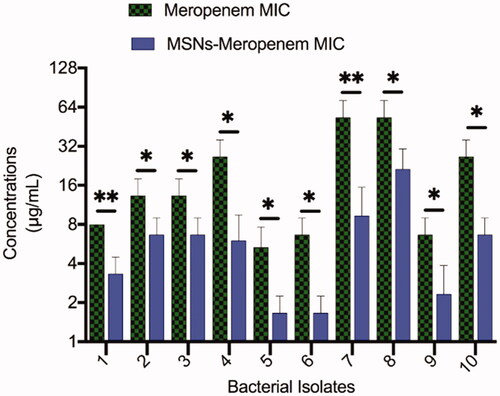
The usefulness of silica-based mesoporous nanomaterial has been reported as an effective delivery system of several antibiotics such as vancomycin, rifampicin and linezolid [Citation38]. Koneru et al. prepared the tetracycline loaded MSNs and studied its antimicrobial effects on Escherichia coli and reported that tetracycline loaded MSNs more effective against bacteria than free tetracycline [Citation12]. MSNs have also been reported to decrease the cytotoxicity of polymyxin B that is presented some toxicity in eukaryotic cells. However, it did not increase the antimicrobial capacity of polymyxin B [Citation13]. MSNs have also been used to delivery of lysozyme [Citation39], essentials oils [Citation40], peracetic acid [Citation41], silver nanocrystals [Citation42] and chlorhexidine [Citation43].
We found a synergic antimicrobial effect between meropenem and MSNs. This synergic interaction may be due to the interaction of MSNs with bacterial growth, which may increase the cellular uptake of meropenem by bacteria cells and resulting in increased inhibition of bacterial cell wall synthesis and ultimately bacterial killing [Citation35].
Haemolysis assay
To study the level of RBCs of MSNs and meropenem-loaded MSNs which leading to 50% lysis of RBCs were treated with different concentrations from 1 to 128 µg/mL for 3 h. As shown in , the haemolysis rates increase in a dose-dependent manner. It is important to study the biocompatibility of nanomaterial with blood cells to understand the effective intravenous usage of antimicrobial agent-loaded nanoparticles for therapy. The haemolysis levels of HRBCs are directly influenced by the amount of MSNs in a wide range of 0–500 μg/mL [Citation44]. Similar to this study, some studies were reported low haemolytic effects of silica nanoparticles on RBCs in low concentration [Citation45,Citation46]. He et al. were reported at the concentration of 500 µg/mL a significant haemolysis is observable and the haemolysis rate extends up to about 14.2% [Citation44]. Such an elevated haemolytic effect of MSNs would be very unfavourable for in vivo usage. The haemolytic effect of silica is associated with reactive oxygen species (ROS) and silanol groups surface at the surface of particles. MSNs also interact electrostatically with membrane proteins and have a high affinity to tetra-alkyl ammonium groups on the surface of RBCs. The presence of ROS on the surface of MSNs and electrostatic interactions induce the harmful effects on RBCs, which are dependent on the zeta potential of particles. Erythrocyte sedimentation rate (ESR) is a hematological variable that is assessed in the exposure to nanostructures to test the biocompatibility [Citation47]. To study the cellular aggregation potential of meropenem-loaded MSNs, the ESR was tested in the exposure of meropenem-loaded MSNs. For the application of meropenem-loaded MSNs in vivo condition, there is possible that the particles will interact with the blood content such as erythrocyte. In this study, ESR was not changed in the exposure to 128 μg/mL of meropenem-loaded MSNs and was found to be at a normal range in comparison to negative control. This result shows the interaction of meropenem-loaded MSNs to the HRBCs is negligible and non-toxic and it is a non-toxic hemocompatible particle.
Cytotoxic effects of meropenem-loaded MSNs
Cytotoxicity of biologically active antibacterial compounds should be studied as an essential stage before in vivo application [Citation40]. In order to assess the cytotoxic effect of meropenem-loaded MSNs, the in vitro viability of the hBM-MSC cells in the exposure of meropenem-loaded MSNs was determined by MTT assay at a serial concentration of 1 − 128 μg/mL which inhibited growth of bacteria. The viability of hBM-MSC cells treated with meropenem-loaded MSNs was 92–100% in all exposed levels from 1 to 128 μg/mL that shown meropenem-loaded MSNs has low cytotoxicity and favourable compatibility in concentrations that it leads to antibacterial activity (). The cell viability was 79–100% in the exposure to bare MSNs that was lower than meropenem-loaded MSNs. Some studies have reported that the cytotoxic effects of MSNs with a variety of cell lines in vitro are fairly high [Citation48]. Different uptake and subsequent cytotoxic effects of MSNs were observed in different cell lines. The difference in response to a biologically active agent between the some cell lines may be due to the difference in the physiological status of each [Citation49]. According to the results of Menono N. and Leong D.T. study, the MSNs are uptake by MCF-7 cells and induced cytotoxic effects in a time and concentration-dependent mode of action. While BJ cells exhibited no significant uptake of MSNs in 24 h and showed a normal cell growth in the exposure of 2.5, 25 and 250 µg/mL of MSNs [Citation50]. Similar to our results, another study was shown no cytotoxicity of MSNs even in the high concentration up to 240 μg/mL [Citation45]. The cytotoxicity effects of silica nanoparticles are results of stimulating ROS formation, pro-inflammatory events and DNA damage, which are size and dose dependent [Citation51,Citation52]. The higher toxicity of bare MSNs than meropenem loaded MSNs in our study may be due to the molecular stabilization of MSNs by meropenem.
Conclusion
Generally, MSNs can be considered as an effective drug delivery system with a great potential in infectious disease treatment. According to our finding, meropenem-loaded MSNs shows good physicochemical possessions, desirable biocompatibility properties and the low in vitro cytotoxicity. Besides, the MIC of meropenem-loaded MSNs was lower than the MIC of meropenem against carbapenem-resistant Enterobacteriaceae. The interaction of meropenem-loaded MSNs with bacteria may be occurred via fusing with bacteria’s cell wall/membrane or adsorption to the cell wall to act as a depot antimicrobial agent. Therefore, MSNs can be considered as a promising drug delivery system against carbapenem-resistant bacteria. However, the use of MSNs for infectious diseases compared to other diseases like cancer is still at an early stage of understanding. The future in vivo investigation on the biological properties and toxicity of meropenem-loaded MSNs is essential for its future clinical studies application.
Authors’ contributions
MM, MY and HG have made a contribution to the research steps or the drafting of the manuscript. SM and SS had contribution to the drafting and scientifically revision of the manuscript. SM and SS are the corresponding authors of the manuscript. All authors read and approved the final manuscript.
Acknowledgements
This article was written based on a dataset from a thesis registered at Tabriz University of Medical Sciences (number 61543). The Vice Chancellor for Research at Tabriz University of Medical Sciences provided financial support for this research that is greatly acknowledged.
Disclosure statement
No potential conflict of interest was reported by the authors.
Data availability statement
The raw/processed data required to reproduce these findings cannot be shared at this time as the data also forms part of an ongoing study.
References
- Memar MY, Ghotaslou R, Samiei M, et al. Antimicrobial use of reactive oxygen therapy: current insights. Infect Drug Resist. 2018;11:567–576.
- Gupta A, Mumtaz S, Li C-H, et al. Combatting antibiotic-resistant bacteria using nanomaterials. Chem Soc Rev. 2019;48(2):415–427.
- Barzegari A, Kheyrolahzadeh K, Hosseiniyan Khatibi SM, et al. The battle of probiotics and their derivatives against biofilms. Infect Drug Resist. 2020;13:659–672.
- Giske CG, Monnet DL, Cars O, et al. Clinical and economic impact of common multidrug-resistant gram-negative bacilli. Antimicrob Agents Chemother. 2008;52(3):813–821.
- Alhashem F, Tiren-Verbeet NL, Alp E, et al. Treatment of sepsis: what is the antibiotic choice in bacteremia due to carbapenem resistant Enterobacteriaceae? World J Clin Cases. 2017;5(8):324–332.
- Akhi MT, Ghotaslou R, Beheshtirouy S, et al. Antibiotic susceptibility pattern of aerobic and anaerobic bacteria isolated from surgical site infection of hospitalized patients. Jundishapur J Microbiol. 2015;8(6): e20309.
- Alizadeh N, Rezaee MA, Kafil HS, et al. Detection of carbapenem-resistant Enterobacteriaceae by chromogenic screening media. J Microbiol Methods. 2018;153:40–44.
- Jacob JT, Klein E, Laxminarayan R, et al. Vital signs: carbapenem-resistant Enterobacteriaceae. MMWR. 2013;62(9):165.
- Wang Q, Zhang Y, Yao X, et al. Risk factors and clinical outcomes for carbapenem-resistant Enterobacteriaceae nosocomial infections. Eur J Clin Microbiol Infect Dis. 2016;35(10):1679–1689.
- Schwaber MJ, Carmeli Y. Carbapenem-resistant Enterobacteriaceae: a potential threat. Jama. 2008;300(24):2911–2913.
- Sharifi S, Fathi N, Memar MY, et al. Anti‐microbial activity of curcumin nanoformulations: new trends and future perspectives. Phytother Res. 2020;34(8):1926–1946.
- Koneru B, Shi Y, Wang Y-C, et al. Tetracycline-containing MCM-41 mesoporous silica nanoparticles for the treatment of Escherichia coli. Molecules. 2015;20(11):19690–19698.
- Gounani Z, Asadollahi MA, Meyer RL, et al. Loading of polymyxin B onto anionic mesoporous silica nanoparticles retains antibacterial activity and enhances biocompatibility. Int J Pharm. 2018;537(1–2):148–161.
- Wang Y, Zhao Q, Han N, et al. Mesoporous silica nanoparticles in drug delivery and biomedical applications. Nanomedicine. 2015;11(2):313–327.
- Bharti C, Nagaich U, Pal AK, et al. Mesoporous silica nanoparticles in target drug delivery system: a review. Int J Pharm Investig. 2015;5(3):124–133.
- Bhar R, Kanwar R, Mehta S. Surface engineering of nanoparticles anchored meso-macroporous silica heterostructure: an efficient adsorbent for DNA. Mater Chem Phys. 2020;255:123541.
- Sunar S. The future potentials of mesoporous silica nanoparticles. J Adv Chem Pharma Mat. 2020;3(2):241–243.
- Hanafi-Bojd MY, Jaafari MR, Ramezanian N, et al. Surface functionalized mesoporous silica nanoparticles as an effective carrier for epirubicin delivery to cancer cells. Eur J Pharm Biopharm. 2015;89:248–258.
- Hanafi-Bojd MY, Ansari L, Mosaffa F, et al. The effect of mesoporous silica nanoparticles loaded with epirubicin on drug-resistant cancer cells. Nanomed J. 2017;4(3):135–141.
- Maleki Dizaj S, Lotfipour F, Barzegar-Jalali M, et al. Ciprofloxacin HCl-loaded calcium carbonate nanoparticles: preparation, solid state characterization, and evaluation of antimicrobial effect against Staphylococcus aureus. Artif Cells Nanomed Biotechnol. 2017;45(3):535–543.
- Weinstein MP. Methods for dilution antimicrobial susceptibility tests for bacteria that grow aerobically. National Committee for Clinical Laboratory Standards. 2018; 38(2): 89–92.
- Memar MY, Adibkia K, Farajnia S, et al. Biocompatibility, cytotoxicity and antimicrobial effects of gentamicin-loaded CaCO3 as a drug delivery to osteomyelitis. J Drug Delivery Sci Technol. 2019;54:101307.
- Achilli C, Grandi S, Ciana A, et al. Biocompatibility of functionalized boron phosphate (BPO4) nanoparticles for boron neutron capture therapy (BNCT) application. Nanomedicine. 2014;10(3):589–597.
- Rahimi M, Safa KD, Salehi R. Co-delivery of doxorubicin and methotrexate by dendritic chitosan-g-mPEG as a magnetic nanocarrier for multi-drug delivery in combination chemotherapy. Polym Chem. 2017;8(47):7333–7350.
- Díaz-García D, Ardiles P, Prashar S, et al. Preparation and study of the antibacterial applications and oxidative stress induction of copper maleamate-functionalized mesoporous silica nanoparticles. Pharmaceutics. 2019;11(1):30.
- Cooper J, Hunt J. The significance of zeta potential in osteogenesis. in Annual Meeting-Society for Biomaterials in Conjunction with the International Biomaterials Symposium. 2006.
- Smeets R, Kolk A, Gerressen M, et al. A new biphasic osteoinductive calcium composite material with a negative zeta potential for bone augmentation. Head Face Med. 2009;5(1):13.
- Zhao Y, Wang Y, Ran F, et al. A comparison between sphere and rod nanoparticles regarding their in vivo biological behavior and pharmacokinetics. Sci Rep. 2017;7(1):1–11.
- Geng Y, Dalhaimer P, Cai S, et al. Shape effects of filaments versus spherical particles in flow and drug delivery. Nat Nanotechnol. 2007;2(4):249–255.
- Li D, Tang Z, Gao Y, et al. A bio‐inspired rod‐shaped nanoplatform for strongly infecting tumor cells and enhancing the delivery efficiency of anticancer drugs. Adv Funct Mater. 2016;26(1):66–79.
- Vega-Jiménez AL, Vázquez-Olmos A, Acosta-Gío E, et al. In vitro antimicrobial activity evaluation of metal oxide nanoparticles. in Nanoemulsions-Properties, Fabrications and Applications. 2019. IntechOpen. 84369.
- Kamba SA, Ismail M, Hussein-Al-Ali SH, et al. In vitro delivery and controlled release of doxorubicin for targeting osteosarcoma bone cancer. Molecules. 2013;18(9):10580–10598.
- Liu Y, Liu X, Xiao Y, et al. A multifunctional nanoplatform based on mesoporous silica nanoparticles for imaging-guided chemo/photodynamic synergetic therapy. RSC Adv. 2017;7(49):31133–31141.
- Seleem MN, Munusamy P, Ranjan A, et al. Silica-antibiotic hybrid nanoparticles for targeting intracellular pathogens. Antimicrob Agents Chemother. 2009;53(10):4270–4274.
- Braun K, Pochert A, Lindén M, et al. Membrane interactions of mesoporous silica nanoparticles as carriers of antimicrobial peptides. J Colloid Interface Sci. 2016;475:161–170.
- Chung KT, Wong TY, Wei CI, et al. Tannins and human health: a review. Crit Rev Food Sci Nutr. 1998;38(6):421–464.
- Samiei M, Farjami A, Dizaj SM, et al. Nanoparticles for antimicrobial purposes in Endodontics: a systematic review of in vitro studies. Mater Sci Eng C Mater Biol Appl. 2016;58:1269–1278.
- Molina-Manso D, Manzano M, Doadrio JC, et al. Usefulness of SBA-15 mesoporous ceramics as a delivery system for vancomycin, rifampicin and linezolid: a preliminary report. Int J Antimicrob Agents. 2012;40(3):252–256.
- Wang Y, Nor YA, Song H, et al. Small-sized and large-pore dendritic mesoporous silica nanoparticles enhance antimicrobial enzyme delivery. J Mater Chem B. 2016;4(15):2646–2653.
- Balaure PC, Boarca B, Popescu RC, et al. Bioactive mesoporous silica nanostructures with anti-microbial and anti-biofilm properties. Int J Pharm. 2017;531(1):35–46.
- Carmona D, Lalueza P, Balas F, et al. Mesoporous silica loaded with peracetic acid and silver nanoparticles as a dual-effect, highly efficient bactericidal agent. Microporous Mesoporous Mater. 2012;161:84–90.
- Liong M, France B, Bradley KA, et al. Antimicrobial activity of silver nanocrystals encapsulated in mesoporous silica nanoparticles. Adv Mater. 2009;21(17):1684–1689.
- Lu M-M, Wang Q-J, Chang Z-M, et al. Synergistic bactericidal activity of chlorhexidine-loaded, silver-decorated mesoporous silica nanoparticles. Int J Nanomed. 2017;12:3577–3589.
- He Q, Zhang J, Shi J, et al. The effect of PEGylation of mesoporous silica nanoparticles on nonspecific binding of serum proteins and cellular responses. Biomaterials. 2010;31(6):1085–1092.
- Chen Y, Chen H, Zhang S, et al. Multifunctional mesoporous nanoellipsoids for biological bimodal imaging and magnetically targeted delivery of anticancer drugs. Adv Funct Mater. 2011;21(2):270–278.
- Slowing II, Wu C-W, Vivero-Escoto JL, et al. Mesoporous silica nanoparticles for reducing hemolytic activity towards mammalian red blood cells. Small. 2009;5(1):57–62.
- Dhar S, Mali V, Bodhankar S, et al. Biocompatible gellan gum-reduced gold nanoparticles: cellular uptake and subacute oral toxicity studies. J Appl Toxicol. 2011;31(5):411–420.
- Murashov V, Harper M, Demchuk E. Impact of silanol surface density on the toxicity of silica aerosols measured by erythrocyte haemolysis. J Occup Environ Hyg. 2006;3(12):718–723.
- Yu T, Malugin A, Ghandehari H. Impact of silica nanoparticle design on cellular toxicity and hemolytic activity. ACS Nano. 2011;5(7):5717–5728.
- Menon N, Leong DT. Cytotoxic effects of phosphonate-functionalized mesoporous silica nanoparticles. ACS Appl Mater Interfaces. 2016;8(3):2416–2422.
- Duan J, Yu Y, Li Y, et al. Toxic effect of silica nanoparticles on endothelial cells through DNA damage response via Chk1-dependent G2/M checkpoint. PLoS One. 2013;8(4):e62087.
- Napierska D, Thomassen LCJ, Lison D, et al. The nanosilica hazard: another variable entity. Part Fibre Toxicol. 2010;7(1):39.