Abstract
Hydrogel serve as bone tissue engineering have lately received great attention for their good biocompatibility and structures similar to natural extracellular matrices. However, a single component polymer hydrogel is generally detrimental to cell adhesion due to the weaker mechanical properties, which limits their application considerably. In an effort to overcome this disadvantage, we adopt an unconventional dual network hydrogels consisting of the polyethylene glycol diacrylate (PEGDA) covalent network, a thiolated chitosan (TCS) ion crosslinking network and thiolated halloysites (T-HNTs) as reinforcing filler. In addition, bone morphogenetic protein-2 (BMP-2) was loaded into the prepared dual network (DN) hydrogel to improve the bone regeneration function of the DN hydrogel. The resulting PEGDA/TCS/T-HNTs hydrogels showed favourable mechanical property, higher crosslinking density, the lower swelling degree, excellent biocompatibility and cell adhesion ability. The histomorphometric and immunohistochemical analyses revealed the excellent bone regeneration ability for composite hydrogel after implant into rat skull defect. Thus, our results indicated that composite scaffold can be applied as a new bone regeneration biomaterial to be applied as a local drug delivery system with good bone induction performance.
Graphic abstract
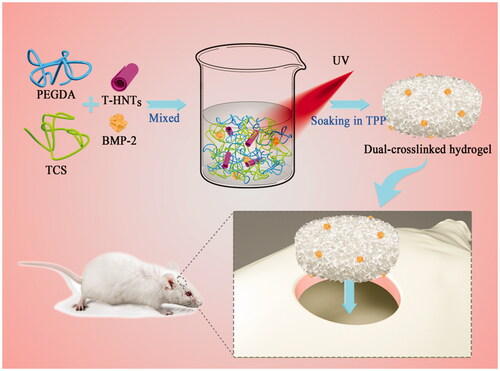
Introduction
Bone defects are common diseases in clinical surgery and are among the health problems that people consider highly important [Citation1]. Among various biological materials, hydrogels have received increasing attention in the field of bone tissue engineering due to their good biocompatibility and structures similar to those of natural extracellular matrices [Citation2–5]. However, a single synthetic polymer hydrogel is generally detrimental to cell adhesion, while natural polymer hydrogels have weaker mechanical properties, which limits their application considerably [Citation6]. Therefore, developing a multifunctional hydrogel that has both excellent mechanical strength and good osteogenic activity remains a serious challenge.
Currently, the construction of a dual network (DN) hydrogel consisting of two asymmetric networks is considered a promising strategy to fulfil high mechanical requirements [Citation7,Citation8]. One network is a rigid polymer network structure with high crosslink density, and the other network is a neutral network structure that maintains hydrogel integrity [Citation9]. For example, Gong et al. designed a swim bladder collagen/poly (N,N'-dimethylacrylamide) double-network hydrogel by a two-step physical/chemical cross-linking method [Citation10]. This DN hydrogel shows excellent mechanical properties comparable to natural cartilage and has good biocompatibility.
In addition, the introduction of nanoparticles into the hydrogel can also achieve an increase in mechanical properties [Citation11,Citation12]. It is well-known that the interaction between polymer molecules and nanoparticles plays a leading role in nanocomposite hydrogel mechanics [Citation13–15]. Halloysite nanotubes (HNTs), natural aluminosilicates with a unique hollow tubular structure, have a high specific surface area, high aspect ratio, good biocompatibility, nontoxicity and low cost [Citation16,Citation17]. Typically, HNTs are added to the polymer matrix as a reinforcing filler to improve the mechanical properties of the polymer due to the unique nanotubular structure [Citation18]. This unique property makes HNTs a good choice to enhance the mechanical properties of the hydrogel while giving the hydrogel a better ability to load bioactive materials.
This study shows the design of a DN hydrogel of a polyethylene glycol diacrylate (PEGDA) covalent network and a thiolated chitosan (TCS) ion crosslinking network by chemical/physical crosslinking. The mechanical properties and drug-loading ability of the prepared DN hydrogel were further improved by introducing thiolated HNTs (T-HNTs). In addition, bone morphogenetic protein-2 (BMP-2) [Citation19] with good osteoinductive properties was loaded into the prepared DN hydrogel to improve the biofunctionality of the composite gel. The dynamic disulphide bonds may generated among BMP2, TCS and T-HNTs, for the free cysteine residue with thiol groups of BMP2, sulfhydrylation-modified TCS and T-HNTs. This dynamic disulphide bonds can not only improve the interfacial interaction between T-HNTs and the polymer chain but also retain the BMP-2 loading into the hydrogel and for the sustained release ability. This study shows the design of a PEGDA/TCS/T-HNTs/BMP-2 hydrogel with excellent mechanical properties and good osteogenic activity and investigated its potential clinical application in orthopaedics.
Experimental section
Materials
Poly (ethylene glycol) diacrylate (PEGDA) (PEG, Mη = 6000) and Thiolated chitosan (TCS, Mw=55 ∼ 65 kDa, concentration of thiol: 215 µmol/g) were supplied by laboratory of Jinan University. Cysteine (standard calibration curve was shown in Figure S1), 2-hydroxy-4′-(2-hydroxyethoxy)-2-methylpropiophenone (Irgacure 2959) and Sodium tripolyphosphate (TPP) were purchased from Sigma-Aldrich. Thiolated Halloysite nanotubes (T-HNTs, concentration of thiol: 156 µmol/g) was fabricated by previous method [Citation20]. Bone morphogenetic protein-2 (BMP-2) active peptide was purchased from China Peptides Co., Ltd (Shanghai, China).
Preparation of PEGDA/TCS/T-HNTs composite hydrogels
The composite hydrogels were constructed via a two-step method. In the first step, T-HNTs (0.05, 0.10, 0.15, and 0.2 g) were dispersed separately in deionized water (10 ml) by magnetic stirring for 45 min and ultrasonic at 700 W for 45 min. Subsequently, 0.3 g TCS and 1.5 g PEGDA were added into the described above, continuously stirred overnight until fully dissolved, and then the 0.1% (w/v) Irgacure 2959 as a photoinitiator was dropwise added to produce a homogeneous mixed solutions consisting of 15 wt% PEGDA, 3 wt% TCS, and different concentration T-HNTs (0.5, 1.0, 1.5, and 2.0 wt%, respectively). The mixed solution above was poured into moulds and exposed to UV light (50 mW/cm2, 365 nm) for 10 min at room temperature. Secondly, the resulting hydrogels were immersed into 5% (m/v) TPP (ionic cross-linker) solution for 30 min to obtain physically cross-linked chitosan molecules. After ionic gelation technique (TPP as ionic cross-linker) and chemical (UV light) double crosslinking, the composite hydrogel was named PEGDA/TCS/T-HNTs hydrogel. In addition, to verify the enhance effect of T-HNTs, a control hydrogel (PEGDA/TCS) was also prepared by PEGDA and TCS via the same method as above.
Characterization of T-HNTs
The Fourier transform infra-red spectroscopy (FT-IR) spectroscopy of HNT and T-HNTs were performed with Vertex 33 spectrophotometer (Bruker, Karlsruhe, Germany). Their zeta potential of samples was measured using a dynamic light scattering (DLS, Malvern Zetasizer NanoZS4700) instrument operating with vertically polarized light from a 633 nm argon-ion laser. Morphology of HNT and T-HNTs were observed via field-emission scanning electron microscopy (FESEM, Ultra55, Carl Zeiss, Germany) under an accelerating voltage of 5 KV.
Characterization of PEGDA/TCS/T-HNTs composite hydrogels
Mechanical property
The tensile tests and compressive evaluation were used to investigate the mechanical behaviour hydrogel system. The details are available in supporting information S I.
Rheological performance
The rheological measurement of hydrogels were conducted through a rotational rheomete (DHR, TA Instruments, USA) equipped with a UV cross-linking instrument (OmniCure S2000) at room temperature, and operated in 20 mm parallel plate geometry and 1 mm gap distance. The details are available in supporting information S I.
SEM observation
The morphology of hydrogels was observed by scanning electron microscopy (SEM) (Zeiss EVO18, Oberkochen, Germany) with an accelerating voltage of 10 kV.
Swelling study
To determine the equilibrium swelling ratios (ESR) of the PEGDA/TCS/T-HNTs composite hydrogels, hydrogel samples were measured using a gravimetric method at 37 °C. The details are available in supporting information S I.
In vitro cell experiment
Mouse pre-osteoblastic cell line (MC3T3-E1) was used to study the cytocompatibility of PEGDA/TCS/T-HNTs hydrogel system. The details are available in supporting information S II.
ALP and Alizarin red staining
ARS (Alizarin red staining) and ALP staining were applied to study the mineral deposition ability of MC3T3-E1 cells. The details are available in supporting information S III.
Study of BMP-2 polypeptide release properties
To obtain the BMP2-loaded hydrogel, the hydrogel (5 mm diameter × 2 mm thickness) was first sterilized in 75% alcohol and washed in ultrapure water. Then, hydrogel immersed into the BMP2 solution (10 μg in 5 ml of phosphate-buffered saline) and kept at 4 °C overnight. The measurement of BMP2 release was carried out to study the stable immobilization of peptides in the polymer network [Citation21]. The details are available in supporting information S IV.
Animal experiments
Forty 12-week-old Sprague Dawley (SD) rats were used in this study. The average weight of the rats was 300 g. This study was approved by the Jinan university laboratory animal ethics, Guangzhou, China (20170912214418). A Zoletil 50 (15 mg/kg; Vibac, Carros, France) was used for general anaesthesia. The bone defect (5 mm in diameter and 2 mm in depth) was created using a trephine bur with saline irrigation. Then defects were implanted with A, B, C and D four kinds freeze-dried scaffolds (control, PEGDA/TCS, PEGDA/TCS/T-HNTs, and PEGDA/TCS/T-HNTs/BMP-2,). The size of the scaffolds is ∼5.0 mm in diameter and 2.0 mm in depth but keep a uniform BMP-2 quality of 10 μg. After grafting, the muscle and skin were closed with suture. For the control group, the rats were only created defect without implant nothing. Five rats from each group were sacrificed at 1, 2, and 3 months after surgery. Specimens were fixed in 10% formalin. Histological analysis was performed to evaluate new bone formation.
Histomorphometric analyses
Following fixation in 4% paraformaldehyde for 48 h, and subsequently decalcified in 10% EDTA for one month before embedding in paraffin, then, the implant area was cut into 5 μm thick sections and stained with haematoxylin and eosin (HE) and Masson's Trichrome (MT).
Identification of regenerated new bone by immunohistochemistry(IHC)
Human origin of engineered bone constructs following in vivo implantation was detected using mouse monoclonal antibody against BMP-2, OCD and CD31 (Abcam, Cambridge, MA, USA) at 3 months. Then, the tissues were performed with immunohistochemical staining of BMP-2, OCN and CD31 on tissue sections according to standard protocol
Statistical analysis
The data were presented as means ± standard deviation (SD). Statistical comparisons of data were performed using GraphPad Prism 6 (GraphPad Software) for one-way analysis. Values of all significant correlations (p < .05) were given with degree of significance indicated.
Results and discussion
Characterization of T-HNTs
Halloysite nanotubes (HNTs) is a biocompatible tubular inorganic material with negative surface on the outer surface and can be served as a promising drug carrier [Citation22,Citation23]. shows the FTIR spectra of HNTs and T-HNTs. Compared to HNTs, a new weak band appeared at 2610 cm−1 for T-HNTs, which is attributed to the stretching vibration of –SH [Citation24]. And the is zeta potential analysis of HNTs and T-HNTs. The surface of the HNTs has a charge of −22.1 mV, while the modified T-HNTs showed a charge of +2.3 mV. Theses above results demonstrate that the thiols group was successfully grafted into HNTs. In addition, morphology and structure of unmodified and modified HNTs was observed by SEM, as shown in , HNTs exhibits a unique long tubular like morphology, and the tubular structure of the T-HNTs is still clearly visible, indicating that the modified process does not damage the unique tubular structure of HNTs. This is consistent with the results reported in previous literature [Citation25].
Preparation of hydrogels
The fabrication of nanocomposite hydrogels is a desirable method that can enhance the mechanical behaviour of hydrogel [Citation26,Citation27]. In this work, PEGDA/TCS/T-HNTs hydrogel was designed and further cross-linked by two-step procedure of UV photopolymerization and TPP physical crosslinking. The synthesis schematic diagram was shown in . It's worth noting that mechanism of TPP as ionic cross-linker based on negatively charged groups reaction with positively charged amino groups of chitosan [Citation28]. TPP has the advantages of stable performance, simple process control and safety and have been s become a widely used technology in the preparation crosslinking chitosan hydrogel. shows a compression graph of the resulting hydrogels, which indicates good compression resistance for the hydrogel.
Mechanical and rheological properties of hydrogels
shows the typical tensile stress-strain curves of the prepared hydrogels. Compared to PEGDA/TCS sample, the tensile strength of PEGDA/TCS/T-HNTs composite hydrogel exhibited significant improvement with the introduction of T-HNTs from 0.5 to 2.0 wt%, and the tensile stress at fracture increased to 190, 245, 305, 360 KPa. The compressive mechanical is an important property for the hydrogel because it mostly suffers compressive stress in bone regeneration process. In compression curves (), it can be seen that the typical “J-shaped” curves resembled biological tissues. PEGDA/TCS/T-HNTs-2.0 displayed the lowest compressive strain at break (∼75.6%) and highest compressive strength (∼1.44 MPa). PEGDA/TCS/T-HNTs-1.5 displayed the compressive strain at break (∼79.1%) and compressive strength (∼1.16 MPa). Moreover, the hydrogels consist of HNTs (1.5 wt%) possessed larger deformation ability than PEGDA/TCS/T-HNTs-2.0 group, which is vital for implantation in skull defect. The mechanism of excellent tensile and compressive property of hydrogels can be explained by the network comprised of hard T-HNTs nanotube and soft PEGDA/TCS chain, the T-HNTs played as physical cross-linkers and crosslinking of thiol between TCS and T-HNTs further preventing the mechanical failure of hydrogel [Citation9,Citation26]. In addition, to verify the thiol crosslinking effect of T-HNTs, we compared the mechanical strength of PEGDA/TCS/T-HNTs-1.5 and PEGDA/TCS/HNTs-1.5 in Figure S2. It can be seen that both the maximum fracture strength and deformation were decreased indicating the tightly interaction between T-HNTs and TCS.
Figure 3. The typical (A) tensile and (B) compression stress-strain curves of PEGDA/TCS and PEGDA/TCS/T-HNTs with different contents of T-HNTs composite hydrogel, (C) the cyclic compressive stress-strain curves of PEGDA/TCS and PEGDA/TCS/T-HNTs-1.5 hydrogel at 70% strain, the rheological results of PEGDA/TCS and PEGDA/TCS/T-HNTs-1.5 hydrogels: (D) time sweeping of storage modulus (G′) and loss modulus (G′′) under a 50 mW/cm2 UV condition, (E) frequency-dependent (at a strain of 1%) and (F) strain-dependent (ω = 6.28 rad/s) oscillatory shear.
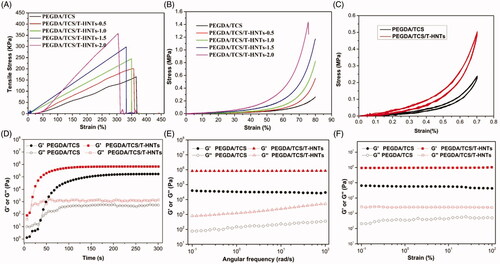
As the ratio of HNTs increased, the tensile and compression stress of PEGDA/TCS/HNTs hydrogel increased. However, the elongation at break was decreased. In addition, the compressive strength of PEGDA/TCS/T-HNTs-1.5 located in the range of the reported compressive strength values of human cancellous bone (∼0.15–13.7 MPa) [Citation29]. In view of the balance the superior strength and deformation ability of PEGDA/TCS/T-HNTs system, the PEGDA/TCS/T-HNTs-1.5 was selected as an optimal formula in further study. The PEGDA/TCS and PEGDA/TCS/T-HNTs hydrogels were subjected to fatigue hysteresis tests with 20 compressive cycles at strain of 70% (). The two hydrogels shows quite stable and well-maintained after 20 cycles without macroscopic fracture, and the narrow gap between the first compression/relaxation and last compression/relaxation curve of testing samples further demonstrated that they were compressible and reversible.
is the result of time sweeping of storage modulus (G′) and loss modulus (G′′) to investigate gelation performance. It can be found that the gelation time had no side effect with the addition of T-HNTs, and the gel point was about 20 s. The G′ and G″ values gradually rise with the prolongation of UV irradiation time, and reach equilibrium of modulus (Ge) at around 100 s. Subsequently, The G′ and G″ of as-prepared hydrogels were tested by oscillation-frequency () and sweeping () mode. The Ge value of the hydrogels were in a stable state and did not relax during the entire frequency (0.1–100 rad/s) or strain (0.1–100%) sweep, their Ge value of PEGDA/TCS and PEGDA/TCS/T-HNTs is 6.7 × 104 Pa, 9.1 × 105Pa obtained from frequency sweep curves, respectively, indicating that stable mechanical properties (formation of perfect three-dimensional polymer network), and there was an enhanced modulus of G′ and G″ after bringing in T-HNTs in the hydrogel substrate. These results prove that the incorporation of T-HNTs favourable enhanced the mechanical property of the composite hydrogels via physically-chemically crosslinking.
Morphology and swelling ratio of hydrogels
The SEM micrographs of PEGDA/TCS, PEGDA/TCS/T-HNTs-0.5 PEGDA/TCS/T-HNTs-1.0 PEGDA/TCS/T-HNTs-1.5 and PEGDA/TCS/T-HNTs-2.0 hydrogel were shown in , respectively. All hydrogels possessed good interconnected porous structures. However, the cross-section of PEGDA/TCS composite hydrogels crosslinked with T-HNTs displays a more regular structure and obvious smaller pores than that of the PEGDA/TCS hydrogel. As the T-HNTs concentration increased to 2 wt%, the average pore size decreased from 36.1 to 10.9 μm. This finding suggests that the HNTs were homogeneously dispersed in the network structure, leading to higher crosslinking density and uniform and smaller pore structure. According to a previous study [Citation30], a highly interconnected structure is important for tissue engineering scaffolds to promote the transportation of bioactive molecules and the migration of cells. The abovementioned results can also be confirmed by the swelling ratio of the prepared hydrogels. As is demonstrated in , the SR of the hydrogels decreased remarkably after the incorporation of T-HNTs. When the contents of T-HNTs increased from 0 to 2 wt%, the SR of PEGDA/TCS/T-HNTs hydrogels decreased from 194 to 75%. Thus, the PEGDA/TCS/T-HNTs-2.0 hydrogel possessed the highest crosslinking density and the lowest swelling degree among all the hydrogels, which is also beneficial to the transfer of stress, resulting in superior mechanical strength [Citation31].
The cytocompatibility of hydrogels
To investigate the cell compatibility cultured onto the PEGDA/TCS/T-HNTs hydrogels, the confocal laser scanning microscopy (CLSM) was performed to observe the adhered morphology after 1, 3, and 7 day (shown in ). PEGDA/TCS/T-HNTs group showed the perfect spread state, in which cells covered the entire surface after cultured 7 days and pseudopodia were highly clear. The favourable adhesion behaviour of PEGDA/TCS/T-HNTs hydrogels might brought by T-HNTs. In the previous reports, HNTs could enhance the adhesion and orientation of stem cells on PLA (polylactic acid) surface [Citation32]. In addition, the thiolated hydrogels might enhance the attachment of proteins via thioether formation [Citation33]. Rosenberg et al. verified involvement of exofacial thiols in fibronectin and collagen-mediated cell adhesion and disulphide-exchange occurred in adhesion process [Citation34]. Moreover, the Cell Counting Kit-8 (CCK-8) was used to measure cell viability. shows the proliferation of MC3T3-E1 cells cultured in vitro for 7 days. The results showed that the cell proliferation increased as the culture time was prolonged. The cells quantity was maximum in PEGDA/TCS/T-HNTs hydrogels surface which is significantly more than PEGDA/TCS group. The favourable proliferation ability of PEGDA/TCS/T-HNTs hydrogels might be brought from the HNTs and thiolation effect via adhesion process.
Figure 5. (A) CLSM micrographs of stained MC3T3-E1 cells showing morphology adhered on PEGDA/TCS, PEGDA/TCS/HNTs and PEGDA/TCS/T-HNTs hydrogels after 1, 3, and 7 days of culture (cytoskeleton and nucleus were stained with rhodamine-conjugated phalloidin (red) and DAPI (blue), respectively, and the scale bar is 50 µm); (B) the corresponding results of MC3T3-E1 cells. The values are represented as mean ± SD (n = 6). *p < .05, **p < .01.
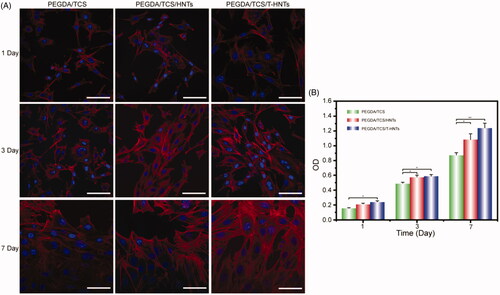
In vitro release behaviour of loading-BMP2 hydrogels
shows an in vitro BMP-2 release curve from PEGDA/TCS, PEGDA/TCS/HNTs and PEGDA/TCS/T-HNTs hydrogels. All the hydrogels system showed no significant burst release and could maintain a sustained release for nearly 24 days. When the time exceeded 24 h, the release curve reached a plateau, and almost no additional BMP2 was released. This is also a favourable phenomenon, because the BMP2 stayed inside the hydrogel, which could help the internal osteoblasts and stem cells to proliferate, differentiate and bone formation. The PEGDA/TCS/T-HNTs/BMP2 system cumulative release of BMP2 is almost 57%, whereas only 86% and 69% of the response value for PEGDA/TCS, PEGDA/TCS/HNTs. This result was probably due to the higher cross-linking density of PEGDA/TCS/T-HNTs/BMP2 hydrogel which can be regarded as a barrier against BMP2 release. Meanwhile, the thiol groups of TCS and T-HNTs interacted with the BMP-2 cysteine residue, resulting in the better stability of BMP-2 in the hydrogel network structure interior [Citation35]. This long-term and sustained release may be beneficial for the regeneration and repair in tissue engineering. The BMP2 loading capacity of three samples was 2.85, 4.79, and 5.81%, respectively. And the drug encapsulation efficiency of PEGDA/TCS/T-HNTs hydrogels was 88%, which is approximately 23 and 13% higher than PEGDA/TCS, PEGDA/TCS/HNTs group, respectively. The enhanced encapsulation efficiency of PEGDA/TCS/T-HNTs hydrogels was mainly due to the effect of the hydrogel structure and the interaction between the polysaccharide long chains and the proteins.
PEGDA/TCS/T-HNTs/BMP-2 regulated MC3T3-E1 cells ossification
Alizarin red s (ARS) and alkaline phosphatase (ALP) staining were also performed to generally observe the effects of PEGDA/TCS/T-HNTs/BMP-2 on mineralization and ALP activity. ARS and ALP staining () performed on day 7 showed that PEGDA/TCS/T-HNTs/BMP-2 hydrogel significantly enhanced the calcium deposition and ALP activity compared with the others group. The ARS semi-quantitative analysis () showed that calcium deposition in PEGDA/TCS/T-HNTs/BMP-2 group was 5.82-fold greater than the control group and 1.92-fold greater than the PEGDA/TCS/T-HNTs group on day 7. In addition, the semi-quantitative analysis of ALP () exhibited similar result for ARS. Our data showed that the significantly osteogenesis effects of PEGDA/TCS/T-HNTs/BMP-2 hydrogel on MC3T3-E1 cells, indicating that the designed hydrogel could be a potential osteogenic-induction scaffold for tissue engineering.
Histomorphometric analyses
In the HE staining image (), mature bone tissue was stained in deep red, and newly regenerated bone were stained in pink colour. When the hydrogels were implanted into SD rats cranial bone, no evident inflammation or teratoma were observed in the PEGDA/TCS, PEGDA/TCS/T-HNTs, and PEGDA/TCS/T-HNTs/BMP2 groups, indicating the hydrogels possessed well biocompatibility in vivo. In the PEGDA/TCS/T-HNTs/BMP2 group, the new-born bone has nearly formed a thin osseous bridge in the defect surface at 1 month and collagen fibres are arranged in order (dotted with blue arrows). In contrast, scarcely any new-born bones were formed in Control, PEGDA/TCS and PEGDA/TCS/T-HNTs group. For the PEGDA/TCS/T-HNTs group at 2 months, the strips-shaped bone has formed with a lot of marrow cavity (yellow oval dotted) inside the new-born bone interior. At the time of 3 months, the PEGDA/TCS/T-HNTs/BMP2 group showed new-born bone with tightly organized compact bone morphology with bits of blood capillary (blue oval dotted) inside indicating that the PEGDA/TCS/T-HNTs/BMP-2 facilitated bone regeneration speed. In addition, the degradation of hydrogel was also important for applying in clinics. At 1 month and 2 months, the PEGDA/TCS, PEGDA/TCS/T-HNTs and PEGDA/TCS/T-HNTs/BMP2 hydrogels could be founded in the defect area while the residual hydrogels were almost degreased completely at 3 months. This results kept consist with the previous study that the degradation of PEGDA composites hydrogels depended on the weight ratio and crosslinking time [Citation36–38].
Figure 8. The HE (A) and Masson (B) staining observations of bone regeneration in cranial bone defects in rats at 1, 2, and 3 months. The scale bar is 1 mm. The black arrow represents mature bone, blue arrow represents new-born bone, yellow colour represents residual hydrogel, yellow oval represents marrow cavity, blue oval represents blood capillary, green oval represents fibrous tissue.
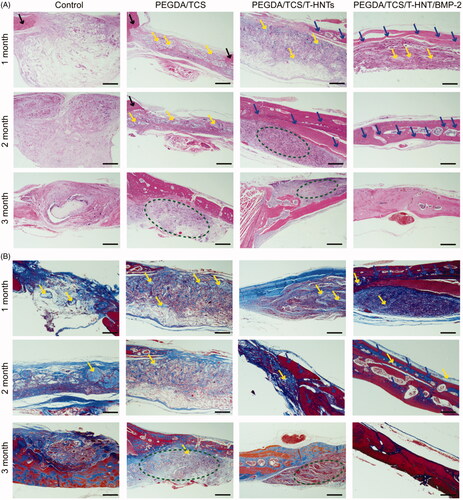
In Masson staining image (), the mature bone tissue was stained in red, and the under reconstructed bone was stained in blue. In the PEGDA/TCS/T-HNTs/BMP2 group, strip-shaped bone tissue area was stained red with blue inside, indicating that new bone was generated in the bone defect at 1 month. While, in the control group, the defect area was occupied by loose tissue which is very disadvantageous for new bone reconstruction. The marrow cavity appeared in group PEGDA/TCS/T-HNTs/BMP2 group at 2 months, illustrating that new-born bone tissue became matured. Finally, the blue staining disappeared in PEGDA/TCS/T-HNTs/BMP2 group at 3 months, indicating that the Masson staining achieved a complete cycle (blue to red) and bone defect achieved completely recovery. The blue staining was also observed in control, PEGDA/TCS, PEGDA/TCS/T-HNTs groups, indicating the un-recovered bone defect. The HE and Masson staining results indicated that PEGDA/TCS/T-HNTs/BMP-2 hydrogels system could accelerate the bone defect area reconstruction efficiency and completed repair at 3 months.
Immunohistochemical examinations of BMP-2, OCN and CD31
To detect the BMP-2-released performance behaviour in the PEGDA/TCS/T-HNTs/BMP-2 group, immunohistochemical staining of BMP-2 was performed. shows that the content of BMP-2 in the PEGDA/TCS/T-HNTs/BMP-2 group was higher than in the other groups at 3 months (dotted with red ovals), illustrating the effective release of BMP-2 from the hydrogel. The results strong demonstrated that BMP-2 facilitated the formation of new bone.
Figure 9. The immunohistochemical BMP-2, OCN and CD31 examinations of the osteogenic potential of the hydrogels were performed at 3 month for control, PEGDA/TCS, PEGDA/TCS/T-HNTs and PEGDA/TCS/T-HNTs/BMP-2 groups, respectively. The scale bar is 100 μm. The red ovals represent BMP2 proteins, green arrows represent OCN proteins, blue arrows represent CD31 proteins.
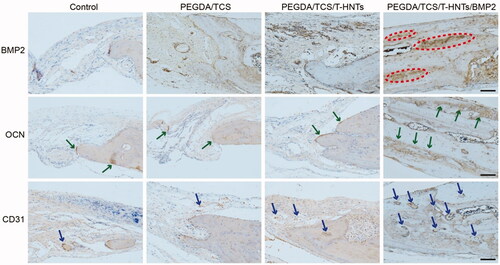
To confirm osteogenesis in the implantation area, we performed an OCN analysis. Osteocalcin is the most important noncollagen protein in the bone matrix and accounts for approximately 1% of the total protein in bone. Osteocalcin is widely accepted as a marker of bone osteoblastic activity [Citation39]. The results obtained for OCN immunohistochemical staining revealed moderate expression of OCN at the edges of the new-born bone in the control, PEGDA/TCS, PEGDA/TCS/T-HNTs groups (dotted with green arrows), while OCN expressed more strongly in the PEGDA/TCS/T-HNTs/BMP-2 group and therefore exhibited better bone regeneration ability.
In the bone regeneration process, angiogenesis could create a capillary network to deliver sufficient nutrients to cells which is crucial for the survival of cells and the healing efficacy of the PEGDA/TCS/T-HNTs/BMP-2 scaffold after implantation [Citation40]. CD31 is known as platelet endothelial cell adhesion molecule-1 which could reflect the formation of blood vessels and served as an endothelial marker of vessels [Citation41]. As shown in immunohistochemical images, many CD31-positive vessels were scattered in the PEGDA/TCS/T-HNTs/BMP-2 group than in the other groups (dotted with blue arrows). The result kept accordance with the previous study. Lvov et al. prepared highly porous chitosan/gelatine/agarose scaffolds (doped with 3–6 wt% halloysite) stimulated neo-vascularization (formation of new blood vessels) in absence of any growth factors or introduced cells [Citation42]. This is due to the composite scaffold doped with HNTs supported cytoskeleton formation, and viability, further guarantee the endothelial cells or smooth muscle cells could penetrate and migrate within the scaffolds to form blood vessel.
Conclusion
PEGDA/TCS/T-HNTs hydrogels were prepared by PEGDA, CS and HNTs via a two-step method. Tensile tests, compressive stress–strain measurements, rheological test, scanning electron microscope, swelling study results confirmed the excellent mechanical property. The favourable cytocompatibility of PEGDA/TCS/T-HNTs hydrogels were confirmed by CCK-8 and CLSM. The BMP2 loading capacity and drug encapsulation and release behaviour of PEGDA/TCS/T-HNTs hydrogels were investigated, which showing no significant burst release and could maintain a sustained release for nearly 24 days due to the hydrogel structure, the molecule interaction of thiolated polysaccharide chains and the BMP2 proteins, T-HNTs and BMP2 proteins. The histomorphometric and immunohistochemical (BMP-2, OCN and CD31) analyses confirmed the enhanced osteogenic potential of composite hydogels. This work develops a dual network hydrogels which shows promising application as novel bone regeneration biomaterial as BMP-2 delivery systems with enhanced osteoinductive properties.
Supplementary_material.docx
Download MS Word (155.8 KB)Disclosure statement
No potential conflict of interest was reported by the author(s).
Additional information
Funding
References
- Li JJ, Akey A, Dunstan CR, et al. Effects of material–tissue interactions on bone regeneration outcomes using Baghdadite implants in a large animal model. Adv Healthcare Mater. 2018;7(15):1800218.
- BaoLin G, Ma PX. Synthetic biodegradable functional polymers for tissue engineering: a brief review. Sci China Chem. 2014;57(4):490–500.
- Liang Y, Chen B, Li M, et al. Injectable antimicrobial conductive hydrogels for wound disinfection and infectious wound healing. Biomacromolecules. 2020;21(5):1841–1852.
- Guo B, Qu J, Zhao X, et al. Degradable conductive self-healing hydrogels based on dextran-graft-tetraaniline and N-carboxyethyl chitosan as injectable carriers for myoblast cell therapy and muscle regeneration. Acta Biomater. 2019;84:180–193.
- Qu J, Zhao X, Ma PX, et al. Injectable antibacterial conductive hydrogels with dual response to an electric field and pH for localized "smart" drug release. Acta Biomater. 2018;72:55–69.
- Cui W, Zhang Z-J, Li H, et al. Robust dual physically cross-linked hydrogels with unique self-reinforcing behavior and improved dye adsorption capacity. RSC Adv. 2015;5(65):52966–52977.
- Yu HC, Li CY, Du M, et al. Improved toughness and stability of κ-Carrageenan/polyacrylamide double-network hydrogels by dual cross-linking of the first network. Macromolecules. 2019;52(2):629–638.
- Xu C, Dai G, Hong Y. Recent advances in high-strength and elastic hydrogels for 3D printing in biomedical applications. Acta Biomater. 2019;95:50–59.
- Huang L, Zhu Z, Wu D, et al. Antibacterial poly (ethylene glycol) diacrylate/chitosan hydrogels enhance mechanical adhesiveness and promote skin regeneration. Carbohydr Polym. 2019;225:115110.
- Mredha MTI, Kitamura N, Nonoyama T, et al. Anisotropic tough double network hydrogel from fish collagen and its spontaneous in vivo bonding to bone. Biomaterials. 2017;132:85–95.
- Wang Q, Hou R, Cheng Y, et al. Super-tough double-network hydrogels reinforced by covalently compositing with silica-nanoparticles. Soft Matter. 2012;8(22):6048–6056.
- Yang X, Bakaic E, Hoare T, et al. Injectable polysaccharide hydrogels reinforced with cellulose nanocrystals: morphology, rheology, degradation, and cytotoxicity. Biomacromolecules. 2013;14(12):4447–4455.
- Li Q, Barrett DG, Messersmith PB, et al. Controlling hydrogel mechanics via bio-inspired polymer-nanoparticle bond dynamics. ACS Nano. 2016;10(1):1317–1324.
- Feng K, Hung G-Y, Yang X, et al. High-strength and physical cross-linked nanocomposite hydrogel with clay nanotubes for strain sensor and dye adsorption application. Compos Sci Technol . 2019;181:107701.
- Xu SA, Gao Y, Guo, L, et al. A hybrid injectable hydrogel from hyperbranched PEG macromer as a stem cell delivery and retention platform for diabetic wound healing. Acta Biomater. 2018;75:63–74.
- Lvov YM, Shchukin DG, Mohwald H, et al. Halloysite clay nanotubes for controlled release of protective agents. ACS Nano. 2008;2(5):814–820.
- Tully J, Yendluri R, Lvov Y. Halloysite clay nanotubes for enzyme immobilization. Biomacromolecules. 2016;17(2):615–621.
- Prashantha K, Schmitt H, Lacrampe M, et al. Mechanical behaviour and essential work of fracture of halloysite nanotubes filled polyamide 6 nanocomposites. Compos Sci Technol. 2011;71(16):1859–1866.
- Hunziker EB, Enggist L, Küffer A, et al. Osseointegration: the slow delivery of BMP-2 enhances osteoinductivity. Bone. 2012;51(1):98–106.
- Jana S, Das S. Development of novel inorganic–organic hybrid nanocomposites as a recyclable adsorbent and catalyst. RSC Adv. 2014;4(65):34435.
- Niu X, Feng Q, Wang M, et al. Porous nano-HA/collagen/PLLA scaffold containing chitosan microspheres for controlled delivery of synthetic peptide derived from BMP-2. J Control Release. 2009;134(2):111–117.
- Feng K, Hung G-Y, Liu J, et al. Fabrication of high performance superhydrophobic coatings by spray-coating of polysiloxane modified halloysite nanotubes. Chem Eng J. 2018;331:744–754.
- Wu Y-P, Yang J, Gao H-Y, et al. Folate-conjugated halloysite nanotubes, an efficient drug carrier, deliver doxorubicin for targeted therapy of breast cancer. ACS Appl Nano Mater. 2018;1(2):595–608.
- Massaro M, Riela S, Lo Meo P, et al. Functionalized halloysite multivalent glycocluster as a new drug delivery system. J Mater Chem B. 2014;2(44):7732–7738.
- Long Z, Wu Y-P, Gao H-Y, et al. Functionalization of halloysite nanotubes via grafting of dendrimer for efficient intracellular delivery of siRNA. Bioconjug Chem. 2018;29(8):2606–2618.
- Deng Z, Guo Y, Zhao X, et al. Multifunctional stimuli-responsive hydrogels with self-healing, high conductivity, and rapid recovery through host–guest interactions. Chem Mater. 2018;30(5):1729–1742.
- Bartoszewski R, Serocki M, Janaszak-Jasiecka A, et al. Collawn, miR-200b downregulates Kruppel like factor 2 (KLF2) during acute hypoxia in human endothelial cells. Eur J Cell Biol. 2017;96(8):758–766.
- Pan C, Qian J, Zhao C, et al. Study on the relationship between crosslinking degree and properties of TPP crosslinked chitosan nanoparticles. Carbohydr Polym. 2020;241:116349.
- Athanasiou KA, Zhu C, Lanctot DR, et al. Fundamentals of biomechanics in tissue engineering of bone. Tissue Eng. 2000;6(4):361–381.
- Wang K, Nune K, Misra R. The functional response of alginate-gelatin-nanocrystalline cellulose injectable hydrogels toward delivery of cells and bioactive molecules. Acta Biomater. 2016;36:143–151.
- Wu D-Q, Wu J, Chu C-C. A novel family of biodegradable hybrid hydrogels from arginine-based poly(ester amide) and hyaluronic acid precursors. Soft Matter. 2013;9(15):3965–3975.
- Wu F, Zheng J, Li Z, et al. Halloysite nanotubes coated 3D printed PLA pattern for guiding human mesenchymal stem cells (hMSCs) orientation. Chem Eng J. 2019;359:672–683.
- Masuko T, Minami A, Iwasaki N, et al. Thiolation of chitosan. Attachment of proteins via thioether formation. Biomacromolecules. 2005;6(2):880–884.
- Rosenberg N, Mor-Cohen R, Sheptovitsky VH, et al. Integrin-mediated cell adhesion requires extracellular disulfide exchange regulated by protein disulfide isomerase. Exp Cell Res. 2019;381(1):77–85.
- Sezlev Bilecen D, Uludag H, Hasirci V. Development of PEI-RANK siRNA complex loaded PLGA nanocapsules for the treatment of osteoporosis. Tissue Eng Part A. 2019;25(1–2):34–43.
- Wang Y, Ma M, Wang J, et al. Development of a photo-crosslinking, biodegradable GelMA/PEGDA hydrogel for guided bone regeneration materials. Materials. 2018;11(8):1345.
- Wang Y, Ma M, Zhang L, et al. Fabrication of bi-layer photocrosslinked GelMA/PEGDA fibrous membrane for guided bone regeneration materials. Mater Lett. 2019;249:112–115.
- Stillman Z, Jarai BM, Raman N, et al. Degradation profiles of Poly(ethylene glycol) diacrylate (PEGDA)-based hydrogel nanoparticles . Polym Chem. 2020;11(2):568–580.
- Johari B, Kadivar M, Lak S, et al. Osteoblast-seeded bioglass/gelatin nanocomposite: a promising bone substitute in critical-size calvarial defect repair in rat. Int J Artif Organs. 2016;39(10):524–533.
- Wang L, Fan H, Zhang Z-Y, et al. Osteogenesis and angiogenesis of tissue-engineered bone constructed by prevascularized β-tricalcium phosphate scaffold and mesenchymal stem cells. Biomaterials. 2010;31(36):9452–9461.
- Mutin M, Dignat-George F, Sampol J. Immunologic phenotype of cultured endothelial cells: quantitative analysis of cell surface molecules. Tissue Antigens. 1997;50(5):449–458.
- Naumenko EA, Guryanov ID, Yendluri R, et al. Clay nanotube-biopolymer composite scaffolds for tissue engineering. Nanoscale. 2016;8(13):7257–7271.