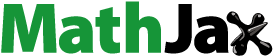
Abstract
Injectable hydrogels attract more attention to hard tissue engineering for the fulfilment of the defects with irregular shapes. Therefore, the researchers investigated the biocompatibility and immune response to the injectable PCL-PEG-PCL-Col/nHA hydrogels in a mouse model. The histological examination was done via H&E. The activation of the immune cells was evaluated by using antibodies against the CD68, CD4, and CD8 markers. The expression of CCL-2, BCL-2, IL-10, and CD31 genes was measured. Moreover, serum levels of the ALT, ALP, AST, and Urea were detected. The results of the chemical analysis showed that the collagen and Nano-hydroxyapatite were successfully integrated into the PCL-PEG-PCL hydrogels. The histological examination revealed a delayed biodegradation rate after the addition of the collagen and Nano-hydroxyapatite. No prominent pro-inflammatory response was found at the site of the injection. There are no significant differences in the levels of the CD68 and CD8/CD4 lymphocyte ratio among groups (p > .05). The expression of the CD31, IL-10 was significantly increased in the PCL-PEG-PCL-Col/nHA hydrogel (p < .05). ALT, ALP, AST, and Urea levels were not altered pre- and post-transplantation of the hydrogels (p > .05). These in vivo results demonstrated that the injectable PCL-PEG-PCL-Col/nHA hydrogels are biocompatible and suitable for further research in hard tissue regeneration.
Introduction
Regeneration strategies in the craniofacial region for repairing and reconstructing the damaged hard tissues including bones, teeth, and cartilage should mimic or promote the oral developmental processes by using the biomaterials to induce the tissue formation via stimulation of the specific cellular function for regaining the function and aesthetic in this area [Citation1]. In general, hard tissue engineering has revolutionized the treatment of injuries by overcoming the conventional drawbacks and has become a promising approach for healing injured tissues. Bone, tooth, and cartilage are complex bio-mineralized hierarchical structures containing the Nano-hydroxyapatite (nHA) and collagen [Citation2–4]. Hence, the ideal alternative to the conventional reconstruction methods should mimic the distinctive properties of the natural host tissue and promote regeneration. Tissue engineering scaffolds should be biocompatible, biodegradable, and have similar composition natural bone extracellular matrix (ECM). This similarity between ECM and scaffold structure could provide a distinct niche for cell migration, adhesion, and proliferation similar to the natural microenvironment, which is described for the tissue [Citation5,Citation6]. It should be noted that the fabricated scaffolds should be biocompatible without the induction of the immune responses to avoid producing inflammatory responses, acute immunogenicity, or cytotoxicity for the transplant cells, tissues, and organs [Citation7].
As 3D scaffolds, the hydrogels consisting of natural and synthetic polymers have many advantages similar to in vivo ECM [Citation5]. Collagen is one of the most common natural polymers in regenerative medicine due to its excellent biological features [Citation8]. However, natural hydrogels are mechanically weak due to a high amount of water absorption. To overcome this limitation, the synthesized polymers and nanoparticles are usually added to the natural polymers to improve the physicochemical properties of the hydrogels [Citation9,Citation10]. Among the synthetic polymers used in tissue engineering, poly (ɛ-caprolactone) (PCL) is biodegradable and biocompatible polyester with superior mechanical strength [Citation11]. However, this polymer is insoluble and has a low degradation rate [Citation12]. Therefore, efforts have been made to circumvent these pitfalls by copolymerization with the polyethylene glycol [Citation13]. Moreover, it has been shown that the nHA particles have the potential to enhance the osteogenesis capacity of the scaffolds. Degradation of these particles releases the calcium and phosphate ions, which could dictate certain mineralization signalling pathways [Citation14–16].
The injectable form of the hydrogels is more appropriate for the clinical application in the oral and maxillofacial regions as they can be implanted with minimal aggressive surgery [Citation17]. The injectable hydrogels could be used to the fulfilment of defects in bone, cartilage, and tooth with irregular contours and shapes which leads to the occupying spaces of the defects and preventing the invagination of the fibrotic tissues [Citation14]. Biocompatibility is the first and important criterion for the scaffolds and demonstrates the ability of the scaffold to perform its application without immune system response [Citation18]. Subcutaneous implantation of the scaffold is considered as the first step in evaluating the biocompatibility [Citation18,Citation19]. In the current study, the researchers aimed to develop a novel combination of the injectable PCL-PEG-PCL-Col/nHA hydrogels and evaluating some features such as biocompatibility, non-toxicity, and biodegradability in vivo.
Material and methods
Materials
-Caprolactone (Mw = 114.14 g/mol), poly (ethylene glycol) 1000 (Mw = 950–1050 g/mol), glutaraldehyde, (25%), and stannous octoate (Mw = 1.251 g/mL) were purchased from Sigma-Aldrich Co. (Steinem, Germany). Diammonium hydrogen phosphate ((NH4)2HPO4) (Mw = 132.06 g/mL), calcium nitrate tetrahydrate (Ca(NO3)2.4H2O) (Mw = 236.15 g/mL), sodium hydroxide (NaOH) (Mw = 40 g/mL), dimethyl sulfoxide (Mw = 78.13 g/mL), methylene chloride (Mw = 84.93 g/mL), Formalin, and ethanol (
Mw = 46.07 g/mol) were supplied from Merck Chemical Co. DMEM high glucose, foetal bovine serum, penicillin/streptomycin, trypsin-EDTA, and Phosphate buffered saline (PBS) were obtained from Gibco, Singapore. RNA extraction kit (Cat No: FABRK001), cDNA synthesis kit, and SYBR Green PCR Master Mix (Cat No: YT4500) were provided by Yekta Tajhiz Azma Company (Tehran, Iran). Collagen (type I, from bovine) was purchased from SBPE Company (Mw116 kDa, Iran).
Synthesis of PCL-PEG-PCL polymer
PCL/PEG/PCL copolymer was prepared by ring-opening polymerization of -caprolactone (2:1 w/w) in the presence of poly (ethylene glycol) 1000, using Sn(Oct)2 (0.5 wt% of monomers) as a catalyst. In summary, the suspension was heated to 140 °C under stirring and in a nitrogen atmosphere for 6 h. Then, the synthesized polymer was dissolved in methylene chloride and re-precipitated in an excess of cold diethyl ether and dried in a vacuum oven at 40 °C for 24 h.
Synthesis of nHA
Nano-hydroxyapatite powders were fabricated by wet chemical precipitation. Briefly, after dissolving of Ca(NO3)2.4H2O and (NH4)2HPO4 in distilled water, the pH of obtained 0.5 M Ca(NO3)2 and 0.3 M (NH4)2HPO4 solutions was adjusted by NaOH. After that, (NH4)2HPO4 solution was added into the Ca(NO3)2 solution dropwise, and stirred for 24 h. Finally, the obtained powders were dried and sintered at 120 and 850 °C, respectively.
Preparation of hybrid scaffold
The PCL-PEG-PCL-Col and PCL-PEG-PCL-Col/nHA scaffolds were prepared using the in situ precipitation method. The 350 mg of the collagen and 350 mg of PCL-PEG-PCL were solved in 10 mL acetic acid 1% and combined with a circulating 25,000 round-trip homogenizer at 4 °C for 2 h. Then, 1% PVA was added to the mixture and circulating 25,000 round-trip homogenizer until homogenous solution produces. In nano-hydroxyapatite group methods like the previous one but 35 mg nHA powder was added when salt and PVA were added. These solutions were kept at −20 °C for three days and −80 °C for one day followed by placing in freeze-dryer for 48 h.
Scanning electron microscope and energy dispersive X-ray (EDX)
Morphology and structure of the synthesised nanocrystals and scaffolds were determined by SEM (MIRA3 FEG-SEM-Tescan, Czech). The pore sizes of porous scaffolds and the size of nano hydroxyapatite particles were determined from SEM micrographs using Image J software (NIH, USA, version 1.52n) as described previously [Citation20]. Moreover, The elemental composition of the synthesized nano-hydroxyapatite particles and PCL-PEG-PCL-Col/nHA hydrogels were analyzed using energy-dispersive X-ray (EDX) attached to the field emission scanning electron microscope (FE-SEM) (MIRA3 FEG-SEM – Tescan, Czech).
X-ray diffraction (XRD)
The phase constitution of the fabricated nano-hydroxyapatite particles and phase structure of this nanoparticle in the synthesized PCL-PEG-PCL-Col/nHA hydrogels were characterized using the XRD test (Bruker Discover 8 X-ray diffractometer; Germany) in the 2θ range 30–80°.
Fourier-transform infrared spectroscopy (FTIR)
The Fourier transform infrared spectroscopy (FTIR) of specimens was recorded by Tensor27 (Bruker-Germany) in the frequency range between 400 and 4000 cm–1. The specimens were firstly poured into a uniform powder with a ratio of one to one hundred pure KBr, and then the powder was made to clear tablet then was placed in special tubes, and spectra were recorded.
Nuclear magnetic resonance spectroscopy (NMR)
The chemical structures of PCEC-Alg and PCEC-Alg/nHA scaffolds were evaluated by NMR 400 MHz Ultrashield (Avance 400 MHZ, Bruker, Germany). 1HNMR and 13CNMR spectra of scaffolds were evaluated after dissolving of scaffolds in Deuterium dimethyl sulfoxide.
Measurement of the sol-gel transition temperature
The sol-gel transition study was carried out using the test tube inverting method. The synthesized copolymer (PCL-PEG-PCL-Col/nHA) was dispersed in PBS in 10 mL vials with 20 wt%. Then NaCl with a concentration of 1.5 wt% was added to the mixture. The prepared samples were examined to determine the phase transition temperature by heating the vials from room temperature to 40 °C in a shaker incubator. The vials were kept at each temperature for 3 min. The samples with no flow within 30 s were considered gel [Citation21].
Alizarin red S staining
Bone marrow mesenchymal stem cells (MSCs) were purchased from the Pasteur Institute (Tehran, Iran). These cells were seeded on sterilized PCL-PEG-PCL-Col and PCL-PEG-PCL-Col/nHA hydrogels. After 14 days, MSCs were fixed in 4% glutaraldehyde for 30 min and stained with 2% Alizarin Red S for 1 h at room temperature to stain calcium deposits. After that, supernatants were transferred to a new plate, and absorbance was measured at 450 nm.
Preparation and subcutaneous transplantation of hydrogels
To yield a homogenous mixture, hydrogels were diluted in sterile phosphate-buffered saline (1:10 Scaffold: PBS) followed gentle stirring at 40 °C. To avoid bacterial contamination, Gentamicin (Alborz Darou Co, Iran) was added to the mixture and aspirated into 3 mL syringes. The biodegradability and biocompatibility of scaffolds were assessed by an in vivo subcutaneous injection of 500 μL from each hydrogel into the supra-flank region of thirty mature 8-week-old male Swiss CD-1 mice, weighing approximately 50 g. Before injection, the region was shaved and sterilely prepared. Then, mice were anaesthetised by IM injection of Ketamine HCL (50 mg/kg) and Xylazine HCL (5 mg/kg). Mice were kept in standard cages under 12:12 light/dark cycle at 20 °C. Postoperative analgesia and antibiotic were provided by IM administration of Tramadol (10 mg/kg) twice a day for three days and Gentamicin (10 mg/kg) daily for five days, respectively. Animals were euthanized following anaesthesia with a mixture of Ketamine and Xylazine 2 weeks after the implantation and tissues were subjected to different analyses. All phases of our study were affirmed by the published guideline of The Care and Use of Laboratory Animals (NIH Publication No. 85-23, revised 1996) and approved by the Ethics committee of Tabriz University of Medical Sciences (IR.TBZMED.REC.1196.512).
Histopathological examination
The subcutaneous specimens were harvested from the location of injections for histopathological examinations. Samples were fixed in 10% neutral-buffered formalin, dehydrated in graded ethanol, and embedded in paraffin (Tissue-processor, Leica, Jung histokinette 2000, Germany). The 6 µm-sized sections (Sliding microtome, Leica, Jung histocuts, Germany) were stained with Haematoxylin and Eosin (H&E) solution. One veterinary pathologist analyzed the histological changes.
Immunofluorescence analysis (IF) of CD68 cells
The number of CD68 (monocyte-macrophage marker) positive cells was examined at the site of injection using IF imaging. Samples from Control, PCL-PEG-PCL-Col, and PCL-PEG-PCL-Col/nHA group were cut into 5 μm thick section. Then, 0.3% Triton-X100 was used for the permeabilization of tissues. Samples were incubated with the primary anti-CD68 antibody (Cat No. M0876; Dako; Denmark) at 4 °C overnight. After twice PBS wash, samples were incubated with FITC-conjugated secondary antibody (Dako, Denmark) for 1 h at room temperature. The 1 µg/mL DAPI solution was used for nuclear staining. CD68+ cells were counted and observed by a fluorescence microscope and analysis with Image J software.
Calculating CD8/CD4 lymphocytes ratio
The CD8/CD4 lymphocytes ratio was determined in peripheral blood by using IF imaging. To this end, blood samples were collected by cardiac puncture into heparinized tubes. Then, samples were smeared on glass slides. Thereafter, slides were air-dried and fixed in pre-chilled methanol (4%) solution for 10 min. Then, the cells were immunophenotyped using anti-CD4-FITC (BD Pharmingen) and anti-CD8-PerCP (BD Pharmingen) according to the manufacturer’s recommendation. We counted the number of CD4 and CD8 in 4 random high-power-fields.
Gene expression analysis
Real-time PCR analysis was performed to evaluate the immune response 14 days after hydrogel transplantation. Total RNA was extracted from control, PCL-PEG-PCL-Col, and PCL-PEG-PCL-Col/nHA groups by using an RNA extraction kit. Then, reverse transcripted into cDNA was used to cDNA synthesis by cDNA kit (Cat No. YT9065). The list of primers, including BCL2, CCL2, IL10, and CD31 was outlined in . The PCR reaction was done using the Rotor-Gene 3000 (Corbett Life Sciences, Australia) system and SYBR Green PCR master mix (Cat No. YT2551). Relative Levels of gene expression were evaluated and the actin gene was used as the housekeeping gene for normalization.
Table 1. Primer list.
Measuring levels of factors correlated with function of renal and hepatic tissues
For this purpose, the blood samples were collected into anticoagulant-free test tubes and allowed to coagulate at room temperature. Then, the samples were centrifuged at 4000 rpm for 10 min to isolate the serum. In this study, we measure the levels of urea, alkaline phosphatase (ALP), alanine transaminase, and aspartate transaminase (AST) using commercial kits by an automatic chemistry analyzer (BS400, Mindray).
Statistical analysis
All experiments were repeated in triplicates and data are presented as mean ± SD and evaluated by One-way ANOVA and Tukey post hoc test. p-value <.05 was considered statistically significant.
Results
Scanning electron microscope and energy dispersive X-ray (EDX)
The SEM evaluations of the Nano-hydroxyapatite particles, PCL-PEG-PCL-Col, and PCL-PEG-PCL-Col/nHA were shown in . The scaffolds demonstrated the uniform structure with homogenous interconnected porous structures with pore size in the range of 50–150 µm ()). Also, in the SEM picture of the nano-hydroxyapatite particles with higher magnification, these particles with semi-oval shape morphology and particle size in the range of 100–200 nm were observed (). After the addition of the nHA, the porosity of the PCL-PEG-PCL-Col/nHA scaffold increased in comparison with the PCL-PEG-PCL-Col scaffold.
Figure 1. FE-SEM image of synthesized nanoparticles, scaffolds, and the corresponding diameter distributions (A) PCL-PEG-PCL-Col; (B) PCL-PEG-PCL-Col/nHA; (C) Nano hydroxyapatite particles. EDX; (D) synthesized nano-hydroxyapatite particles; (E) PCL-PEG-PCL-Col/nHA.
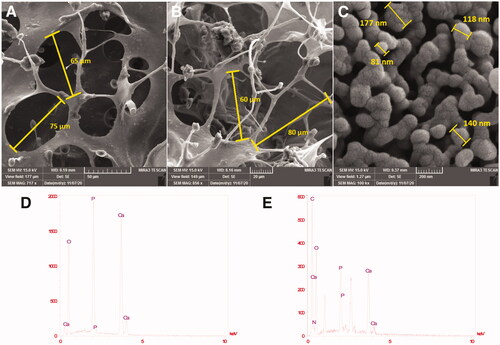
EDX analysis of three batches of synthesized nano-hydroxyapatite particles showed calcium and phosphorus exhibiting a ratio (Ca/P) of 1.73 0.08, which is in proximity to the desired value of 1.67 (). The presence of calcium and phosphorus proved the successful synthesis of the hydroxyapatite. In the EDX pattern of the PCL-PEG-PCL-Col/nHA scaffold, the presence of calcium and phosphorous proved the incorporation of HA in the scaffold ().
X-ray diffraction (XRD)
The XRD analysis of the PCL-PEG-PCL-Col/nHA scaffold is represented in . XRD analysis confirmed that the synthesis process applied to achieve the PCL-PEG-PCL-Col/nHA was successful. From XRD analysis, the peaks of n-HA were detected at 2θ = 32°, 38, 46°, 56°, and 67°. Moreover, the collagen characteristic peak appeared at 44°.
Fourier-transform infrared spectroscopy (FTIR)
FTIR spectra of the PCL-PEG-PCL-Col and PCL-PEG-PCL-Col/nHA scaffolds are demonstrated in . In spectra of the PCL-PEG-PCL-Col, the characteristic bands at 1731 and 1297 cm−1 are related to the C=O, C–C, and C–N groups of PCL. The absorption bands at 1187 cm−1 are attributed to the C–O–C stretching vibrations of the repeated OCH2CH2 units of PEG. Collagen revealed the characteristic bands at 1651 and 1548 cm−1 corresponding to the stretching vibrations of the amide I and amide II groups 1244 cm−1 due to the C–N bond. The intense peak that appeared at 3200–3500 peak at cm−1 was related to N–H and COOH groups of the collagen. The peaks of carboxyl group ν(C=O) of collagen were overlapped with (C=O) of the esteric groups of the PCL at 1731 cm−1. In the spectra of the PCL-PEG-PCL-Col/nHA (), the stretching vibration peaks of the phosphate groups related to nHA appeared at 565 cm−1.
Nuclear magnetic resonance spectroscopy (NMR)
The H-NMR spectra of the PCL-PEG-PCL-Col/nHA are shown in . The peaks that appeared at 1.2–1.3, 1.5, 2.2, 2.5, and 3.9 ppm were related to the PCL section of the copolymer. The peak at 3.3 ppm was due to the presence of the PEG section of the copolymer. Characteristic peaks of the Col appeared at 1.6 ppm and 4–4.5 ppm. Collagen amide peak appeared at 8 ppm. The C-NMR spectrum of the PCL-PEG-PCL-Col/nHA is shown in . The peaks that appeared 24–40 ppm and 63.49 were attributed to the C–H and C-O of the PCL section of the copolymer. The peak that appeared at 69.788 ppm corresponded to the C-O of the PEG and collagen section of the hydrogel. The peak at 172 ppm was related to the carboxylate (O–C = O) section of PCL and collagen.
Sol-gel transition studies
The synthesized PCL-PEG-PCL-Collagen/nHA copolymer displayed a temperature-dependent reversible sol-to-gel transition in PBS (). The polymer solution (20 wt%) was in a free-flowing clear sol state at the ambient temperature. Increasing the temperature to 37 °C made the solution convert to a clear gel (lower transition temperature).
Alizarin red S staining
The mineralization of MSCs in the synthetized PCL-PEG-PCL-Collagen, PCL-PEG-PCL-Collagen/nHA, and the control group is demonstrated in . Compared to the control and PCL-PEG-PCL-Collagen groups, cells on PCL-PEG-PCL-Collagen/nHA showed higher ARS activity (p < .05).
Macroscopic and histological examinations
Before the animal euthanization, no systemic complications such a shock, septicaemia, toxaemia, and speared inflammatory reactions in all studied groups were found. On gross examination, it was shown that significant amounts of the injected hydrogels were absorbed especially in the PCL-PEG-PCL group compared to the PCL-PEG-PCL-Col and PCL-PEG-PCL-Col/nHA groups ()). The hydrogels enriched with nHA were identified as the opaque white nodules while the PCL-PEG-PCL-Col hydrogel exhibited a swollen and transparent appearance 14 days after transplantation. Compared to PCL-PEG-PCL-Col/nHA hydrogel, it seems that the PCL-PEG-PCL-Col group had a high rate of water adsorption capacity indicated by a soft consistency ()). According to our observations, the PCL-PEG-PCL-Col/nHA nodules had more consistency and firmness compared to the PCL-PEG-PCL-Col group. Through the present study, the average diameter of the PCL-PEG-PCL-Col and PCL-PEG-PCL-Col/nHA reached between 2 and 8 mm.
Figure 7. Gross and histological examination of the transplantation site after 14 days (A–C). In group PCL-PEG-PCL, the transplant mass was considerably biodegraded while PCL-PEG-PCL-Col and PCL-PEG-PCL-Col/nHA exhibited a slow biodegradation rate. Bright-field imaging showed the lack of PCL-PEG-PCL substrate beneath the epidermis while remnants of PCL-PEG-PCL-Col and PCL-PEG-PCL-Col/nHA were surrounded by fibrotic tissue. These data showed the stability of PCL-PEG-PCL-Col and PCL-PEG-PCL-Col/nHA hydrogels in in vivo conditions after 14 days.
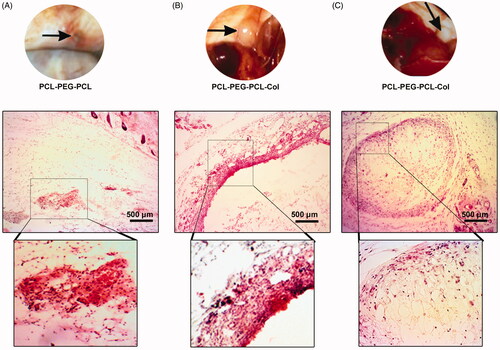
Histological examination revealed the lack of acute inflammatory response at the site of the injection after 14 days ()). In group PCL-PEG-PCL, a small number of immune cells at the site of injection scavenging the remnants of the hydrogel has been found. These features showed the prominent quick biodegradability of the PCL-PEG-PCL hydrogel in in vivo. In groups PCL-PEG-PCL-Col and PCL-PEG-PCL-Col/nHA, a marked fibrotic layer surrounded the remained hydrogel after 2 weeks, which possibly correlates with the prolonged biodegradation time ()). No superficial and deep dermatitis and panniculitis were shown in the PCL-PEG-PCL-Col and PCL-PEG-PCL-Col/nHA groups. Taken together, these data showed that the addition of the Col and nHA to the PCL-PEG-PCL backbone increased the biodegradation time and postponed hydrogel resorption in in vivo conditions.
Addition of nHA and col to PCL-PEG-PCL did not change CD68 recruitment and alter CD8/CD4 lymphocyte ratio
Data from IF imaging revealed that the non-significant differences in the number of CD68-positive cells at the site of the injection among all groups (p > .05; ). Despite the high average number of CD68 positive cells in the PCL-PEG-PCL, these values were not statistically significant in comparison with the groups PCL-PEG-PCL-Col and PCL-PEG-PCL-Col/nHA. The frequencies of the CD4 and CD8 lymphocytes were also counted in the blood smear prepared from all groups (). Similar to the number of the CD68 positive cells at the site of the injection, we found statistically nonsignificant differences in blood CD8/CD4 lymphocyte ratio among the groups (p > .0; ). These data possibly show that the subcutaneous transplantation of PCL-PEG-PCL derivatives enriched with nHA and Col could not provoke the severe pro-inflammatory response and alter the systemic CD8/CD4 lymphocyte ratio, indicating the biocompatibility of PCL-PEG-PCL-Col and PCL-PEG-PCL-Col/nHA in in vivo condition.
Figure 8. (A) Measuring the levels of local CD68 positive cells and (B) blood CD8/CD4 lymphocyte ratio using IF staining. Data showed the lack of statistically significant differences in the levels of CD68 cells and CD8/CD4 lymphocyte ratio between the groups. These data showed that PCL-PEG-PCL-Col and PCL-PEG-PCL-Col/nHA hydrogels well-tolerated in vivo without a magnificent immune system reaction.
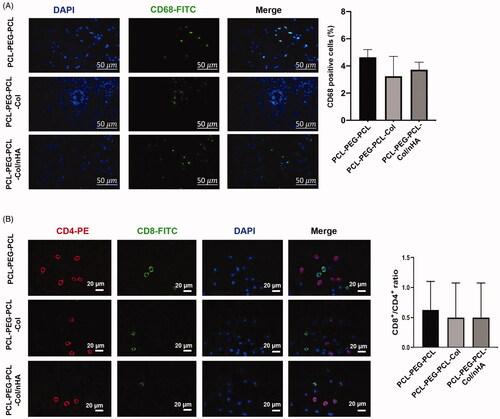
An elevated angiogenesis rate and T regulatory function was determined using real-time PCR analysis
Here, the researchers monitored the expression of the different genes related to the immune system function and vascularization (). The expression of the BCl-2 and CCL-2, macrophage activation factors, was investigated and data showed that the addition of nHA and Col to the PCL-PEG-PCL backbone increased the activation of the genes related to the macrophage lineage (p < 0.05; ). The maximum levels of the BCL-2 expression were found in mice that received the PCL-PEG-PCL-Col compared to the PCL-PEG-PCL and PCL-PEG-PCL-Col/nHA groups. According to data, the PCL-PEG-PCL-Col substrate has a superior effect to induce the expression of the BCL-2 in comparison with the PCL-PEG-PCL-Col/nHA group (p < .01; ). Despite the induction of the CCL-2 in PCL-PEG-PCL-Col, the differences were statistically non-significant. Real-time PCR analysis confirmed the activation of a gene, IL-10, related to the function of T regulatory lymphocytes. Data showed that the injection of the PCL-PEG-PCL-Col and especially PCL-PEG-PCL-Col/nHA hydrogels up-regulated the expression of IL-10 at the site of the injection compared to the PCL-PEG-PCL substrate. Like the dynamic expression of IL-10, the researchers found an enhanced angiogenesis rate at the site of the injection containing PCL-PEG-PCL-Col and PCL-PEG-PCL-Col/nHA hydrogels compared to the control PCL-PEG-PCL group (p < .05; ). To this end, the researchers monitored the expression of CD31, an endothelial marker, 14 days after subcutaneous transplantation. The accessed data showed that both PCL-PEG-PCL-Col and PCL-PEG-PCL-Col/nHA promoted significantly the expression of CD31 (p < .05; ).
Figure 9. (A) Measuring the expression of BCL2, CCl-2, IL10, and CD31 in subcutaneous transplants after 14 days using real-time PCR assay (n = 6). (B) Serum levels of AST, ALT, ALP, and Urea in PCL-PEG-PCL, PCL-PEG-PCL-Col, and PCL-PEG-PCL-Col/nHA groups after 14 days (n = 6). *p < .05, **p < .01, ***p < .001, and ****p < .0001.
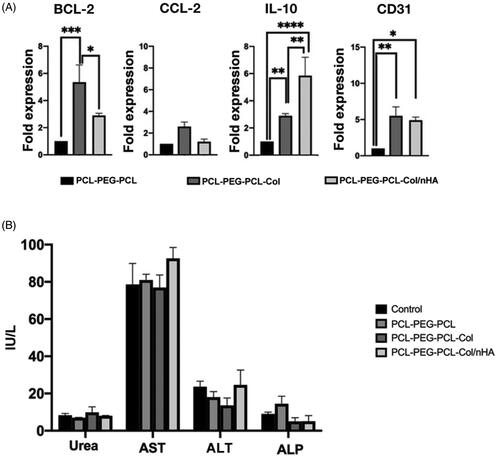
Subcutaneous injection of PCL-PEG-PCL derivatives did not alter the function of hepatic and renal tissues
Serum levels of different factors such as ALT, ALP, AST, and Urea were measured by using the biochemical kits to monitor the function of the renal and hepatic tissues (). The results showed non-significant differences in the levels of ALT, ALP, AST, and Urea in all studied groups after 14 days. These data showed that the injection of the PCL-PEG-PCL, PCL-PEG-PCL-Col, and PCL-PEG-PCL-Col/nHA did not produce byproducts, which could exert toxic effects on the liver and kidneys.
Discussion
The initial step for determining its biocompatibility of the synthesized scaffolds is the subcutaneous or intramuscular implantation. In a study by Sumayya and Kurup, the biocompatibility of the cross-linked hydroxyapatite, alginate, chitosan, and fucoidan scaffolds were evaluated in the same manner and the results of the histopathological analysis proved their scaffolds biocompatibility [Citation19]. Another study which was conducted by Gui-Bo et al. reported the in vivo biocompatibility of electrospun PLA/silk fibroin-gelatin scaffold by the subcutaneous implantation in the animal model of the rat [Citation22].
Through the current study, the injectable PCL-PEG-PCL-Col/nHA hydrogels were developed and investigated in vivo biocompatibility after the subcutaneous injection in the model of the mouse. In tissue engineering, the PCL has been widely used as a raw material for the synthesis of the scaffolds due to the gradual degradation in in vivo conditions, good mechanical strength, and appropriate biocompatibility [Citation13,Citation23]. Despite these advantages, the absence of molecular motifs leads to inappropriate cell attachment and growth [Citation24]. Thus, many attempts have been gathered to improve the PCL surface interaction by the addition of natural products [Citation13,Citation23].
Type 1 Col possesses cell-binding sites consisted of RGD and GFOGER sequences. These motifs provide the suitable binding sites that made the Col choice component for the synthesis of the engineered scaffolds [Citation23,Citation25]. Therefore, the Col is combined with different polymers and biomaterials for hard tissue engineering as well as cartilage, bone, and tooth [Citation26,Citation27]. The collagen-PCL binding occurs through COOH and improves cell binding and growth, which could be explained by the fact that the bioactive molecules improve the collagen characteristics [Citation23]. In the present research, both combined scaffolds PCL-PEG-PCL-Col and PCL-PEG-PCL-Col/nHA were soluble in aqueous solvents and provided the suitable structures as shown in SEM images. Moreover, the sol-gel transition occurred in the body temperature after subcutaneous injection. Other studies evaluated in situ gel-forming injectable scaffolds with the collagen and chitosan polymers for bone tissue engineering [Citation6,Citation28,Citation29]. Also, in the current study, the researchers designed and fabricated the injectable hydrogels by combining the synthesized PCL/PEG/PCL polymer with natural collagen polymer for the hard tissue regeneration in the craniofacial region. The addition of the nano-hydroxyapatite particles to this composition as well as shown in FTIR and XRD analysis was considered to improve the mineralization in the host tissue. Although, the EDX analysis confirmed the Ca, P, and O ions in the structure.
Biocompatibility is the ability of the biomaterials to accomplish their desired functions in the implanted tissues, without causing any undesirable local or systemic reaction in the host but inducing the most appropriate beneficial cellular or tissue response in the recipient and optimizing the clinically relevant performance of the treatment [Citation28]. To evaluate body reaction and hydrogel biocompatibility, the researchers used a mouse model of the subcutaneous transplantation as well as the other studies [Citation18,Citation19]. Based on the histological examination, the researchers found that the PCL-PEG-PCL backbone biodegraded faster than the PCL-PEG-PCL-Col and PCL-PEG-PCL-Col/nHA substrates. In groups that received the PCL-PEG-PCL-Col and PCL-PEG-PCL-Col/nHA, a mild to moderate immune reaction was initiated to digest the subcutaneous masses by generating a granulation tissue due to the delayed biodegradation rate. Those features showed that a combination of the Col and nHA with PCL-PEG-PCL backbone produced a mixture that was stable to in vivo degradation mechanisms in the short-term period. The modulation of the hydrogel biocompatibility and degradation kinetics was in close association with the tissue type and function. The reduction of the degradation rate seemed strategic policy in the tissues such as bone with slow healing time compared to the soft tissues [Citation30].
The possible immunological effects of the hydrogels PCL-PEG-PCL-Col and PCL-PEG-PCL-Col/nHA were evaluated by monitoring the local CD68 macrophages and systemic CD8/CD4 lymphocyte ratio. The results showed a statistically non-significant difference in the levels of the immune cells in all groups. The obtained data showed that all the PCL-PEG-PCL derivate and the existence of the crosslink among the components were well-tolerated in vivo without any changes in the percentage of the immune cells. Additionally, monitoring the levels of the BCL-2 and CCL-2 showed a superior effect of the PCL-PEG-PCL-Col substrate to recruit the macrophages into the site of the injection. The minimum effect of the PCL-PEG-PCL in the recruitment of the CD68 cells into the site of the injection may relate to the rapid degradation rate at the earlier time points while the PCL-PEG-PCL-Col and PCL-PEG-PCL-Col/nHA were more resistant to the degradation mechanisms.
Another interesting data to the present study correlated with the increase of the IL-10 in the mice that received the PCL-PEG-PCL-Col and PCL-PEG-PCL-Col/nHA, showing activation of the anti-inflammatory responses. It has been shown that the IL-10 prohibited the signalling pathways stimulating the MHC class II molecules in the antigen-presenting cells [Citation31]. The increase of the IL-10 coincided with the elevation of the CD31 in PCL-PEG-PCL-Col and PCL-PEG-PCL-Col/nHA groups, showing the enhanced angiogenesis and vascularization rate, which was important in hard tissue engineering. The increase of the CD31 was primarily associated with the proliferation and recruitment of the endothelial cells to the site of the injection to vascularize the transplant mass to accelerate the biodegradation [Citation32]. Besides, these data demonstrated that the PCL-PEG-PCL-Col and PCL-PEG-PCL-Col/nHA were both suitable to be vascularized of the bone and tooth tissue after transplantation, and blood nourishment was vital to transplant the cells inside these hydrogels [Citation33].
When the hepatic cells and their membrane were lysed and damaged, the amounts of AST, ALT, ALP enzymes increased in the peripheral blood and these elevated levels were the sign of liver damage [Citation19]. Monitoring the systemic levels of the AST, ALT, ALP, and Urea showed the lack of hepatotoxicity and renal injury in the groups that received the PCL-PEG-PCL-Col and PCL-PEG-PCL-Col/nHA.
Conclusion
Overall, the results demonstrated the lack of the adverse reaction in the host animal model, which showed that the PCL-PEG-PCL-Col and PCL-PEG-PCL-Col/nHA hydrogels were biocompatible and biodegradable and their degradation products were not toxic to the kidney and liver of the mouse model. Moreover, the addition of the nano-hydroxyapatite significantly induced the mineralization in vitro. Hence, the composite, biodegradable, and biocompatible PCL-PEG-PCL-Col/nHA could be used as an injectable biomaterial for the regeneration of the hard tissues. However, more in vivo induction of the mineralization in bone, cartilage, and tooth should be established in large animal models for better insights on the application of the injectable PCL-PEG-PCL-Col/nHA in the clinical practice.
Disclosure statement
No potential conflict of interest was reported by the author(s).
Data availability statement
The data that support the findings of this study are available from the corresponding author, [Dr. Aghazadeh and Dr. Alipour], upon reasonable request.
Additional information
Funding
References
- Obregon F, Vaquette C, Ivanovski S, et al. Three-dimensional bioprinting for regenerative dentistry and craniofacial tissue engineering. J Dent Res. 2015;94(9 Suppl):143S–152S.
- Utreja A, Dyment NA, Yadav S, et al. Cell and matrix response of temporomandibular cartilage to mechanical loading. Osteoarthr Cartil. 2016;24(2):335–344.
- Bessa PC, Casal M, Reis R. Bone morphogenetic proteins in tissue engineering: the road from laboratory to clinic, part II (BMP delivery). J Tissue Eng Regen Med. 2008;2(2–3):81–96.
- Polini A, Wang J, Bai H, et al. Stable biofunctionalization of hydroxyapatite (HA) surfaces by HA-binding/osteogenic modular peptides for inducing osteogenic differentiation of mesenchymal stem cells. Biomater Sci. 2014;2:1779–1786.
- Bendtsen ST, Wei M. Synthesis and characterization of a novel injectable alginate-collagen-hydroxyapatite hydrogel for bone tissue regeneration. J Mater Chem B. 2015;3(15):3081–3090.
- Huang Z, Feng Q, Yu B, et al. Biomimetic properties of an injectable chitosan/nano-hydroxyapatite/collagen composite. Mater Sci Eng: C. 2011;31(3):683–687.
- Cheung H-Y, Lau K-T, Lu T-P, et al. A critical review on polymer-based bio-engineered materials for scaffold development. Compos Part B: Eng. 2007;38(3):291–300.
- Ferreira AM, Gentile P, Chiono V, et al. Collagen for bone tissue regeneration. Acta Biomater. 2012;8(9):3191–3200.
- Xavier JR, Thakur T, Desai P, et al. Bioactive nanoengineered hydrogels for bone tissue engineering: a growth-factor-free approach. ACS Nano. 2015;9(3):3109–3118.
- Balazs AC, Emrick T, Russell TP. Nanoparticle polymer composites: where two small worlds meet. Science. 2006;314(5802):1107–1110.
- Samadian H, Ehterami A, Sarrafzadeh A, et al. Sophisticated polycaprolactone/gelatin nanofibrous nerve guided conduit containing platelet-rich plasma and citicoline for peripheral nerve regeneration: in vitro and in vivo study. Int J Biol Macromol. 2020;150:380–388.
- Samadian H, Farzamfar S, Vaez A, et al. A tailored polylactic acid/polycaprolactone biodegradable and bioactive 3D porous scaffold containing gelatin nanofibers and Taurine for bone regeneration. Sci Rep. 2020;10(1):12.
- Hokmabad VR, Davaran S, Aghazadeh M, et al. Effect of incorporating Elaeagnus angustifolia extract in PCL-PEG-PCL nanofibers for bone tissue engineering. Front Chem Sci Eng. 2019;13(1):108–119.
- Barros J, Ferraz MP, Azeredo J, et al. Alginate-nanohydroxyapatite hydrogel system: Optimizing the formulation for enhanced bone regeneration. Mater Sci Eng C: Mater Biol Appl. 2019;105:109985.
- Samadian H, Maleki H, Allahyari Z, et al. Natural polymers-based light-induced hydrogels: promising biomaterials for biomedical applications. Coord Chem Rev. 2020;420:213432.
- Samadian H, Mobasheri H, Azami M, et al. Osteoconductive and electroactive carbon nanofibers/hydroxyapatite nanocomposite tailored for bone tissue engineering: in vitro and in vivo studies. Sci Rep. 2020;10(1):14.
- Ruel-Gariepy E, Leroux J-C. In situ-forming hydrogels-review of temperature-sensitive systems. Eur J Pharm Biopharm. 2004;58(2):409–426.
- Jaiswal AK, Dhumal RV, Bellare JR, et al. In vivo biocompatibility evaluation of electrospun composite scaffolds by subcutaneous implantation in rat. Drug Deliv Transl Res. 2013;3(6):504–517.
- Sumayya A, Muraleedhara Kurup G. Biocompatibility of subcutaneously implanted marine macromolecules cross-linked bio-composite scaffold for cartilage tissue engineering applications. J Biomater Sci Polym Ed. 2018;29(3):257–276.
- Hokmabad VR, Davaran S, Aghazadeh M, et al. Fabrication and characterization of novel ethyl cellulose-grafted-poly (ɛ-caprolactone)/alginate nanofibrous/macroporous scaffolds incorporated with nano-hydroxyapatite for bone tissue engineering. J Biomater Appl. 2019;33(8):1128–1144.
- Alami-Milani M, Zakeri-Milani P, Valizadeh H, et al. Preparation and evaluation of PCL-PEG-PCL micelles as potential nanocarriers for ocular delivery of dexamethasone. Iran J Basic Med Sci. 2018;21(2):153–164.
- Gui-Bo Y, You-Zhu Z, Shu-Dong W, et al. Study of the electrospun PLA/silk fibroin‐gelatin composite nanofibrous scaffold for tissue engineering. J Biomed Mater Res Part A 2010;93:158–163.
- Kim MS, Kim G. Three-dimensional electrospun polycaprolactone (PCL)/alginate hybrid composite scaffolds. Carbohydr Polym. 2014;114:213–221.
- Polo-Corrales L, Latorre-Esteves M, Ramirez-Vick JE. Scaffold design for bone regeneration. J Nanosci Nanotechnol. 2014;14(1):15–56.
- Samadian H, Maleki H, Fathollahi A, et al. Naturally occurring biological macromolecules-based hydrogels: Potential biomaterials for peripheral nerve regeneration. Int J Biol Macromol. 2020;154:795–817.
- Eftekhari A, Maleki Dizaj S, Sharifi S, et al. The use of nanomaterials in tissue engineering for cartilage regeneration; current approaches and future perspectives. IJMS. 2020;21(2):536.
- Ghavimi MA, Bani Shahabadi A, Jarolmasjed S, et al. Nanofibrous asymmetric collagen/curcumin membrane containing aspirin-loaded PLGA nanoparticles for guided bone regeneration. Sci Rep. 2020;10(1):18200.
- Chen Y, Li S, Li X, et al. Noninvasive evaluation of injectable chitosan/nano-hydroxyapatite/collagen scaffold via ultrasound. J Nanomater. 2012;2012:1–7.https://doi.org/http://dx.doi.org/10.1155/2013/728130
- Huang Z, Tian J, Yu B, et al. A bone-like nano-hydroxyapatite/collagen loaded injectable scaffold. Biomed Mater. 2009;4(5):055005.
- Rezwan K, Chen Q, Blaker JJ, et al. Biodegradable and bioactive porous polymer/inorganic composite scaffolds for bone tissue engineering. Biomaterials. 2006;27(18):3413–3431.
- Alhamdi J. Modulating aged macrophages and osteoprogenitors with a calcium phosphate drug delivery system [Doctoral dissertation]. Connecticut: University of Connecticut; 2018.
- Rücker M, Laschke MW, Junker D, et al. Angiogenic and inflammatory response to biodegradable scaffolds in dorsal skinfold chambers of mice. Biomaterials. 2006;27(29):5027–5038.
- Sun G, Shen Y-I, Kusuma S, et al. Functional neovascularization of biodegradable dextran hydrogels with multiple angiogenic growth factors. Biomaterials. 2011;32(1):95–106.