Abstract
Plants can produce cellular metal nanoparticles (NPs) from the uptake of metal ions, but the mechanism remains unclear. This work reported the new insight into different fates of iron (Fe) and nickel (Ni) ions to transform into the metal NPs in Azolla pinnata roots. After exposing to ferric nitrate, nickel nitrate, and a combination of both for 12 h, the energy dispersive X-ray fluorescence analysis indicated the efficient uptakes of both metal ions in the roots and their transports into the shoots. Transmission electron microscope images revealed the accumulation of spherical FeNPs, but not NiNPs, near the cell wall and cell membrane, and inside vacuoles and multivesicular bodies in cortical and vascular cells at the root tips. The energy dispersive X-ray analysis suggested that the formation of metal NPs depended on the sufficient concentration of metal ions localized in the roots. FeNPs were identified to ɑ-Fe2O3 and Fe3O4 by selected area electron diffraction analysis. The formation of FeNPs might involve the increase of superoxide dismutase activity. This work is the first report about the cellular biogenesis of metal NPs in plant roots that likely depends on cellular metal content and involves the reducing activity of antioxidant enzymes.
Graphical Abstract
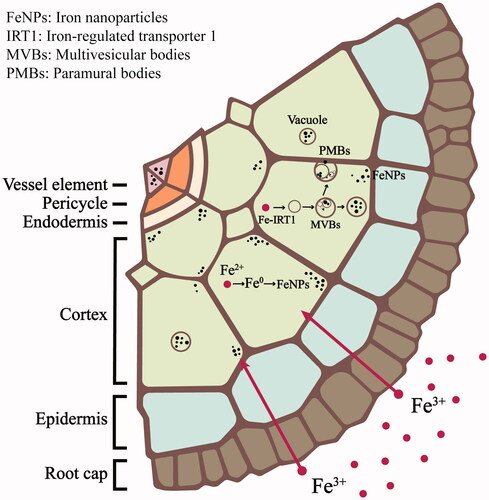
The uptake of Fe3+ ions, but not Ni2+ ions, could transform into NPs inside the root cells of A. pinnata.
FeNPs were detected near the cell wall and cell membrane and inside vacuoles and multivesicular bodies in the root cells of A. pinnata.
The cellular metal concentration was significant for regulating the formation of metal nanoparticles in the plant cells.
Cellular FeNPs were identified to ɑ-Fe2O3 (hematite) and Fe3O4 (magnetite).
Highlights
Introduction
Metal nanoparticles (NPs) have received many research interests due to their outstanding chemical and physical properties at the nanoscale size, which were suitable for diverse applications such as catalysts, optics, biomedical, and environmental applications [Citation1–8]. According to the high demand for these NPs, various approaches for their synthesis have been proposed, mainly via physical and chemical methods. In the physical methods, metal NPs are produced through the reduction of a bulk metal to nanosized metal particles by cutting, milling, or laser-irradiating [Citation9,Citation10]. In contrast, chemical methods are less expensive and simpler than physical methods, making them suitable for an industrial mass-production of metal NPs. Chemical synthesis is based on a reduction of metal ions to zero-valent metal and eventually assembled to NPs. This synthesis requires reducing and stabilizing agents, in which chemical reagents and polymers are generally used [Citation11]. Alternatively, there were reports of biogenesis of metal NPs in living organisms. Some bacteria could produce intracellular metal NPs. For example, Bacillus sp. reduced Ag+ ions to Ag0 and formed the crystalline structure of silver NPs in the periplasmic space after exposure to the aqueous AgNO3 solution. Some bacteria could produce intracellular metal NPs. For example, Bacillus sp. reduced Ag+ ions to Ag0 and formed crystalline structure of silver NPs in the periplasmic space after exposing to the aqueous AgNO3 solution. Magnetotactic bacteria could produce membrane-bound iron NPs accumulated in specific intracellular organelles, the magnetosomes, from the uptake of iron ions [Citation12]. In principle, these bacteria synthesize iron NPs via the binding of the uptake Fe3+ with iron-binding molecules, chelation them to Fe2+, and controlled nucleation and growth of FeNPs in magnetosomes by specific genes and proteins [Citation13]. Nevertheless, the intracellular formation of MNPs in the higher eukaryotes, particularly macrophytes, remains inexplicit.
Some plant species are known as metal-hyperaccumulator plants because they can absorb and store metal ions at high levels inside the cells [Citation14]. Therefore, many studies focus on the molecular actions of these plants under metal stress. The metal-hyperaccumulator plants can cope with a high metal environment via several mechanisms. In roots, the uptake metals are bound to some complex molecules of the cell wall components, thus reducing their translocation to other parts of the plant or direct them into the storage vacuoles [Citation15]. Although the process of metal storage in vacuoles is well characterized, the transformation of cellular metal ions into NPs remains unclear. As plant extracts were reported to mediate the in vitro synthesis of several metal NPs [Citation16–18], we hypothesized that a cellular formation of metal NPs might be potential by natural reducing and stabilizing molecules inside the plant cells. Another question in this work to elucidate is whether the biogenesis of metal NPs depends on metal type.
In this work, iron (Fe3+) and nickel (Ni2+) ions were used as the tested ions to study their transformation into metal NPs in the plant cells, which root cells were emphasized since they are the prime sites of high metal concentration. The water velvet (Azolla pinnata), one of the metal-hyperaccumulator plants, was chosen as the model plant because of its capability to uptake both metal ions at high levels [Citation19,Citation20]. Thus, this work aimed to study the potential biogenesis of FeNPs and NiNPs from their uptake ions in the root cells of A. pinnata. Also, the cellular antioxidant activities, elemental compositions, and macromolecular profiles of the metal-treated plants were evaluated to understand the formation process of metal NPs.
Materials and methods
Chemicals
Iron (III) nitrate (Fe(NO3)3) and Nickel (II) nitrate (Ni(NO3)2) were purchased from VWR (Leuven, Belgium) and MERCK (Darmstadt, Germany), respectively. Glutaraldehyde was from Unilab (Auckland, New Zealand). The low viscosity embedding media Spurr’s kit was from Electron Microscope Science (Hatfield, PA, USA). All other chemicals used were of analytical grade.
Toxicity of Fe(NO3)3 and Ni(NO3)2
Water velvets were raised in a cement tank containing tap water and sedimentary soil in the plant nursery at the Suranaree University of Technology, Nakhon Ratchasima, Thailand. Young plants of similar sizes were carefully detached from the main plant without root damage at the abscission layer of a vegetative multiplication. The collected plants were washed and incubated in deionized (DI) water for 24 h to remove any sediment impurities. Then, they were exposed to various concentrations (10−100 mM) of Fe(NO3)3 and Ni(NO3)2 at ambient temperature (29−32 °C) for 12 h. Three beakers (ten plants per beaker) were used for each concentration. The samples were washed with 10 mM ethylenediaminetetraacetic acid (EDTA) and rinsed with deionized (DI) water to remove the excess metal ions. The toxicity was calculated by the percent of damaged leaves (brown or withered leaves). Two independent experiments were performed to confirm the results.
Energy dispersive X-ray fluorescence analysis
The uptakes of iron and nickel ions in A. pinnata treated with Fe(NO3)3 and Ni(NO3)2 were determined by the energy dispersive X-ray fluorescence (EDXRF) spectroscopy (Horiba Scientific, Kyoto, Japan). The plant samples were exposed to 50 mM Fe(NO3)3, 50 mM Ni(NO3)2, and the mixture of both solutions (1:1 molar ratio) at ambient temperature for 12 h. At the end of the experiment, the excess metal ions were removed by washing with 10 mM EDTA and DI water. The metal uptake and changes of other elemental concentrations in the plants were investigated by EDXRF using an X-ray analytical microscope system. The system can be accomplished using the Rh X-ray tube source, X-ray tube voltage of 50 kV, Peltier cooled silicon drift selector, and 100 s frame counts−1. Thirty-one elements were analyzed by the EDXRF spectrometer; 13Al, 14Si, 15P, 16S, 17Cl, 19K, 20Ca, 22Ti, 23V, 24Cr, 25Mn, 26Fe, 27Co, 28Ni, 29Cu, 30Zn, 37Rb, 42Mo, 43Tc, 44Ru, 45Rh, 46Pd, 47Ag, 48Cd, 77Ir, 78Pt, 79Au, 80Hg, 81Ti, 82Pb, and 83 Bi. Each elemental content was detected for seven locations in each frame and reported as the average data of the total detected mass.
Biomolecular profiles of metal-treated plants
The biomolecular profiles of the plant leaves and roots in response to Fe(NO3)3 and Ni(NO3)2 treatments were analyzed by attenuated total reflectance-Fourier transform infra-red spectroscopy analysis (ATR-FTIR; Bruker, Billerica, MA, USA). The plant samples were treated with 50 mM Fe(NO3)3, 50 mM Ni(NO3)2 and the mixture of both solutions (1:1 molar ratio) at ambient temperature for 12 h. The samples were washed with 10 mM EDTA followed by DI water to remove excess residues of solutions. The leaves and roots (0.5 cm from the tips) were cut and dried at 50 °C in an oven. The dried samples were subjected to ATR-FTIR analysis using IR transmission mode with the frequency range of 400−4000 cm−1. The infra-red spectra were finally analyzed using the software system Opus 7.0 (Bruker, Billerica, MA, USA).
Detection of cellular metal NPs
A transmission electron microscope (TEM) was used to study the formation of metal NPs inside the plant roots. The A. pinnata samples were treated with 50 mM Fe(NO3)3, 50 mM Ni(NO3)2, and the mixture of both solutions (1:1 molar ratio) for 12 h. Treated samples were washed with 10 mM EDTA and DI water and then fixed in 2.5% glutaraldehyde in 0.1 M sodium phosphate buffer, pH 7.4. After washing three times with the sodium phosphate buffer and DI water, the samples were dehydrated in acetone series (30, 50, 75, 95, and 100%) and embedded in a resin at 60 °C for 48 h. They were cross-sectioned (100 nm) using a PowerTome-XL ultramicrotome (RMC Boeckeler, Tucson, AZ, USA) using a diamond knife. Ultrathin sections were collected on bare 200 mesh copper grids and then examined by TEM (Tecnai G2 20 S-Twin, FEI, Hillsboro, OR, USA) operating at 120 kV. The images were captured by a Gatan Orius 200 CCD camera (Gatan, Pleasanton, CA, USA). The diameters of NPs were measured from TEM images (randomly picked 100 NPs) using image analysis of Fiji (ImageJ2) software (Madison, WI, USA).
Characterization of cellular metal NPs
The crystalline structure of the formed NPs inside the treated and control roots was analyzed by selected area electron diffraction (SAED) using a Tecnai G2 S-Twin TEM operating at an accelerating electron source of 200 kV with LaB6 filament and using a Gatan Orius 200 CCD camera. The elemental compositions in roots and leaves were analyzed using a field emission scanning electron microscope equipped with energy dispersive X-ray spectroscopy (FE-SEM/EDX; JEOL JSM 7800 F, Tokyo, Japan). The plant samples were preserved in 2.5% glutaraldehyde in 0.1 M sodium phosphate buffer overnight. They were cross-sectioned (100 µm) with a stainless steel blade before dehydrating in the graded series of ethanol (30, 50, 75, 95, and 100%). The plant sections were dried by vacuum drying at room temperature and attached to the stub with carbon tape. The samples were subjected to EDX analysis of FE-SEM operating at 2 kV. Two independent experiments were performed to confirm the results.
Superoxide dismutase activity
The leaf and root samples of A. pinnata (0.2 g fresh weight) were homogenized in a chilled mortar and pestle with liquid nitrogen. They were suspended in ice-cold homogenization buffer (100 mM phosphate buffer, pH 7.4) and centrifuged at 10,000 ×g for 20 min. The superoxide dismutase (SOD) activity was determined by its activity to inhibit the photochemical reduction of nitro blue tetrazolium (NBT). The 2-ml reaction consisted of 50 mM phosphate buffer (pH 7.8), 2 mM EDTA, 9.9 mM L-methionine, 55 μM NBT, and 0.025% Triton-X100. Then, 40 µl of plant extract and 20 μL of 1 mM riboflavin were added to the reaction mixture. The reaction tubes were placed under a 15 W fluorescent tube for 10 min, before measuring the absorbance at 560 nm. One enzyme unit of SOD is defined as the amount of protein (in mg) causing a 50% inhibition of the photoreduction.
Glutathione reductase activity
The glutathione reductase (GR) activities in leaf and root samples of A. pinnata were measured at 412 nm, which the 5,5′-dithiobis (2-nitrobenzoic acid) (DTNB) was reduced to 5-thio-2-nitrobenzoic acid (TNB). In the 1-ml reaction, plant extract (10 µl) was added in the mixture of 0.75 mM DTNB and 0.1 mM NADPH. After oxidized glutathione (GSSG, 1 mM) was added to start the reaction, the absorbance at 412 nm was recorded every 30 s for 2 min. The activity of GR was calculated, using the extinction coefficient of TNB (14.15 M−1 cm−1). The GR activity was expressed as mmole TNB min−1 g−1 (fresh weight).
Statistical analysis
The quantitative data were expressed as mean ± standard deviation. Statistical comparisons were performed using one-way ANOVA, and the difference between treatment means was compared by Tukey’s honestly significant difference test at a p-value of 0.05 with SPSS 17.0 for Windows software (Chicago, IL, USA).
Results and discussion
Toxicity effects of Fe(NO3)3 and Ni(NO3)2 to A. pinnata
Before studying the ability of A. pinnata to uptake and transform iron and nickel ions to NPs, the toxicities of Fe(NO3)3 and Ni(NO3)2 were examined by the physical changes of the plant leaves (brown, withered leaves). The toxicity was represented as the percent of damaged leaves of the plant samples. shows the toxicity of Fe(NO3)3 and Ni(NO3)2 at various concentrations to A. pinnata, in which the toxicity effects of both metals on A. pinnata were in a dose-dependent response. Also, the toxicity of Fe(NO3)3 was significantly higher than Ni(NO3)2, which their 50% toxicity concentrations were 32.61 and 84.33 mM, respectively. shows the toxicity of 50 mM Fe(NO3)3, 50 mM Ni(NO3)2, and the mixture of both solutions, which their toxicities to the plant samples were 94.15 ± 0.97%, 4.02 ± 0.46%, and 97.75 ± 0.65%, respectively. The results clearly showed that A. pinnata was more sensitive to Fe(NO3)3 than Ni(NO3)2. Also, the additive toxicity effect was detected when both solutions were used to treat the plants. In a vascular plant, the leaf discoloration in response to Fe(NO3)3 is a significant symptom of Fe toxicity called “bronzing” in which the excess ions causing the high generation of free radicals and the induction of plant-defending system, leading to the accumulation of polyphenol oxides [Citation21,Citation22]. The accelerated free radicals mediated via the Fenton/Haber-Weiss reaction can cause the impaired functions of protein, lipid, carbohydrate, and DNA, eventually leading to cell death. For nickel effects on plants, nickel is generally considered less toxic (at least 100 times) than other trace elements as evaluated by its toxic concentration [Citation23]. Nevertheless, the exceed level of nickel and exposure time influence multiple toxicity effects in the plants, including induction of oxidative stress, inhibition of photosynthesis, reduction of growth, inhibition of enzymatic activities, and interference of other metal uptakes [Citation24]. Although iron and nickel ions are necessary micronutrients for plant growth, at above optimal concentrations, they become toxic and cause physiological alterations and multiple toxicity indications, including chlorosis and necrosis [Citation25].
Cellular uptake levels of iron and nickel ions
The uptakes of iron and nickel ions were analyzed by EDXRF in the plants exposed to Fe(NO3)3 and Ni(NO3)2. The elemental mapping images of these plants are in . The mapping images show the distribution of 26Fe throughout the plants exposure to Fe(NO3)3 solution. Based on the EDXRF analyses of 31 elements, high contents of 26Fe (91.44 ± 6.00% mass) and 28Ni (83.45 ± 3.43% mass) were detected in the plants treated with Fe(NO3)3 and Ni(NO3)2, respectively. In the condition with both metals (1:1 molar ratio), the 26Fe and 28Ni levels in the plants were 59.45 ± 6.20 and 34.41 ± 6.27% mass, respectively. These results suggested that A. pinnata could uptake iron ions more efficiently than nickel ions. The detection of both ions in the plant leaves indicated the movement of both ions from roots to other parts of the plant. The modulated levels of 17Cl, 19K, and 20Ca were also detected in both roots and leaves, indicating that these metals involved metal-stress responses in the plant cells.
Figure 2. EDXRF mapping images of 26Fe, 28Ni, 16S, 17Cl, 19K, and 20Ca in A. pinnata exposed to Fe(NO3)3 and Ni(NO3)2.
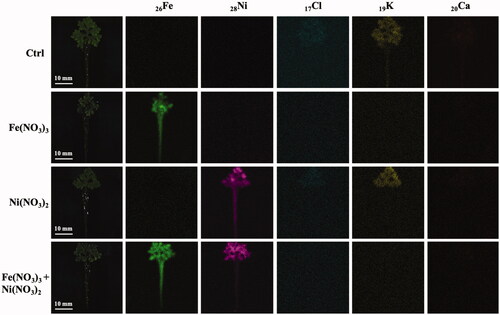
The quantitative data of these metals in roots and leaves are shown in , respectively. In a single metal treatment, the accumulated contents of 26Fe or 28Ni in the plant roots were significantly higher than that in leaves. It was likely due to the translocation distance from the metal uptake site. Exposure to both metals (1:1 molar ratio), the detection of 26Fe content in the plant roots was higher than that of 28Ni. However, in the leaves, the level of 28Ni was significantly higher than 26Fe, suggesting that 28Ni was more efficient to translocate to the leaves. In other words, the primary sink of 28Ni was in leaves, but that of 26Fe was in roots [Citation24, Citation26]. These results also suggested the interference of Ni2+ to Fe3+ accumulation and translocation as they share the same loading and transport processes, including transmembrane proteins for active transport (iron-regulated transporter; IRT) and primary chelators responsible for the mobility (NA and citrate) [Citation27,Citation28]. Moreover, the significant reduction contents of 17Cl, 19K, and 20Ca were detected in both roots and leaves of the plants exposing to Fe(NO3)3 and Ni(NO3)2 as compared with the control plants. It might result from a lower uptake capability of the plant roots and the cell membrane leakage due to the toxicity of excess levels of iron and nickel in the plants [Citation29].
Changes of biomolecular profiles in response to metal treatments
The responses of A. pinnata to the metal treatments could be determined by the changed profiles of functional groups of the plant biomolecules as determined by ATR-FTIR analysis. shows the ATR-FTIR spectra of the roots of A. pinnata exposed to Fe(NO3)3 and Ni(NO3)2. In Fe(NO3)3 and Ni(NO3)2 treated plants, FTIR spectra revealed the changes in peak intensity and new emergent peak as compared with the control plants. Six spectral peaks were modulated in response to these metal treatments, which were in the ranges of 3342−3329, 1632−1616, 1556−1539, 1384−1373, 1057−1053, and 672 cm−1. These spectral peaks corresponded to O–H stretching vibration of alcohol [Citation30], N–H bending vibration of primary amine [Citation30], N–H bending vibration of secondary amide [Citation31], symmetric COO– stretching of carbonyl group [Citation30], C–O stretching vibration of primary alcohol [Citation32], and M–O bond vibration [Citation33], respectively. In the treated plants, two reduction peaks at 3342−3329 and 1057−1053 cm−1 (O–H and C–O of primary alcohol) suggested the possible reduction of some carbohydrates in response to Fe and Ni treatments. The reduction of starch synthesis was reported as one of the metal stress effects in plants. In metal-treated plants, a lower photosynthesis was detected, resulting from metal-induced disruption of light harvesting complex II, the basic pigment-protein complex in photosystem II, and reduction of chlorophyll contents [Citation34]. In contrast, the increased peak intensity at 1384−1373 cm−1 (COO– stretching of carbonyl group) suggested a possible induction of some carbohydrates under a metal stress condition. The inductions of pectin and antioxidant sugars were reported in metal-stress plants. Pectin production was induced to capture and prevent metal translocation into the cells [Citation35]. Increased levels of antioxidant sugars were significant in plants to cope with metal toxicity effects by scavenging reactive oxygen species (ROS) [Citation36]. In addition to carbohydrates, there was a possible reduction of some proteins in metal-treated plants as indicated by the reduced peak intensity at 1632−1616 cm−1 (N–H of primary amine). There was a report of metal stress effects on a protein folding process, resulting in misfolded and non-functioned proteins. These proteins were subsequently degraded through a ubiquitin-proteasome process or autophagy, resulting in reducing levels of some proteins in metal-stress plants [Citation37]. In contrast, there were also possibly newly synthesized proteins in response to metal stress, suggesting new emergent peak at 1556−1539 cm−1 (N–H of secondary amide). The newly synthesized proteins might be related to the metal detoxification processes, such as phytochelatins, metallothionines, and antioxidant enzymes [Citation38]. Another emergent peak was detected at 672 cm−1 (M–O bonding), indicating the formation of metal-oxygen bonding occurring in the metal-treated plants.
Biogenesis of metal NPs in A. pinnata
TEM analysis was employed to study the formation of FeNPs and NiNPs from the uptakes of Fe3+ and Ni2+ by A. pinnata roots. shows the representative TEM images of cross-sections of cortical cells of A. pinnata roots exposed to Fe(NO3)3 and Ni(NO3)2 (single and dual treatments). The FeNPs were detected as the dark particles near the cell wall and cell membrane of cortical cells in the plant samples treated with Fe(NO3)3 and both Fe(NO3)3 and Ni(NO3)2. In the plant samples treated with both metals, FeNPs were additionally found in vacuoles near the cell membrane. Also, the production of multivesicular bodies and the plasmolysis were distinguished in these root-sections. The average diameter of FeNPs in cortical cells was 8.08 ± 2.65 nm as measured from randomly selected 100 particles, which their sizes ranged from 2.40 to 16.44 nm. However, there was no detection of NiNPs in all sections of the plant samples treated with Ni(NO3)2.
Figure 5. TEM images of cortical cells of A. pinnata roots: (A) control, (B) Fe(NO3)3 treatment, (C) Ni(NO3)2 treatment, and (D,E) both metal treatments. Abbreviations: c: cortical cell; v: vacuole; mvbs: multivesicular bodies. Arrows and arrow heads indicated nanoparticles and plasmolysis, respectively.
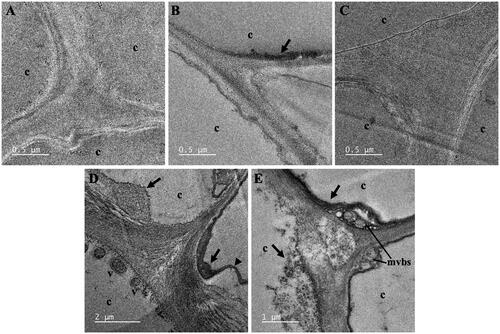
shows the representative TEM images of vascular cells of A. pinnata roots exposed to Fe(NO3)3 and both Fe(NO3)3 and Ni(NO3)2. With Fe(NO3)3 exposure, FeNPs were observed near the cell wall of the metaxylem and in paramural bodies of pericycle cells. With both metal treatments, FeNPs also localized near the cell membrane of pericycle cells. The plasmolysis was also observed. It was likely due to the osmosis of water to the outside of the cell in a hypertonic environment. The average diameter of FeNPs in vascular cells was 18.53 ± 10.24 nm, which their diameters ranged from 4.47 to 52.86 nm.
Figure 6. TEM images of vascular cells of A. pinnata roots: (A,B) Fe(NO3)3 treatment, and (C,D) Fe(NO3)3 and Ni(NO3)2 treatments. Abbreviations: mx: metaxylem; p: pericycle; ph: phloem; pmb: paramural body. Arrows and arrow heads indicated nanoparticles and plasmolysis, respectively.
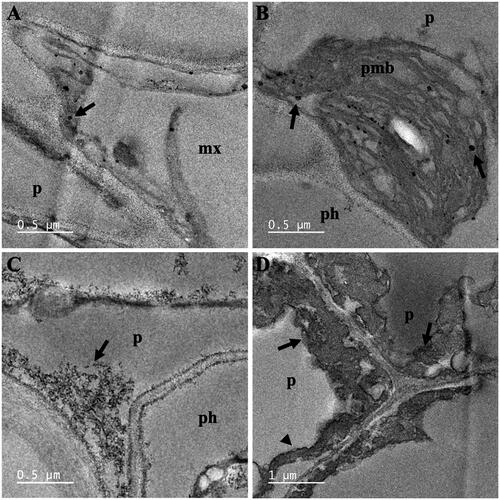
The possible mechanism involved in the formation of metal NPs in the plant cells remains unclear. In this work, we hypothesize that the metal ions were reduced to zero-valent metals by the reducing agents in the cells, such as phenolic compounds, vitamins, reducing sugar, terpenoids, and some metabolites. After the metal seed nuclei occurred, they could grow into metal clusters and eventually metal NPs via the facilitation of cellular stabilizing agents, such as complex phytochemicals and carbohydrates [Citation39]. In this work, the dominant accumulation sites of FeNPs were recognized at the cell wall and cell membrane of root cells, suggesting their significant roles as the first line of defense to cope with excess metals. As the negatively-charged pectin and hemicelluloses are mostly localized at the cell wall, they can absorb positively-charged ions, resulting in the decreased metal toxicity from their translocation to other parts of the plant [Citation40]. The formation of metal NPs in the roots of A. pinnata was hypothesized to involve the suitable concentration of metal ions in the cells. As root cells are the iron sinks, these iron ions might be reduced and formed the seed nuclei. With a high concentration of iron ions, these seed nuclei feasibly grew into FeNPs. It was also speculated that nickel ions were mobile and easily transported to other parts of the plant. As a result, their insufficient concentrations inside the cells caused no formation of NiNPs. In a dual metal treatment, the production of multiple vacuoles inside the vascular cells was hypothesized to involve the mechanism to reduce the metal toxicity when exposed to a very high metal concentration. The accumulation of metal ions in vacuoles was efficient for decreasing the cellular toxicity of these excess metals. Also, as metal ions were very high in vacuoles, the metal NPs could be formed inside the vacuoles. In this work, only FeNPs were detected in the vacuoles as determined by SAED-TEM analysis. It was hypothesized that iron ions were effectively stored in the vacuoles but not for nickel ions. In a dual metal treatment, the multivesicular bodies were detected, which their formation might be induced by the hypertonic environment. As the cell lost into the outside, it caused the induction of the inward bulging of the plasma membrane, thus making a cell wall–plasma membrane interspace filled with vesicles [Citation41]. As metal ions were also captured inside the multivesicular bodies, they could be induced to form the metal NPs.
Identity of cellular metal NPs
Cellular FeNPs in the root cells were characterized by SAED-TEM. SAED is a crystallographic analysis operating with TEM, which can determine the crystal structure of the specimen from the diffraction pattern of the electron beam scattered by the sample lattice. The diffraction pattern appears as an array of “spots,” which corresponds to specific sets of crystal lattice planes in the specimen, denoted by Miller indices (hkl). In the Fe(NO3)3-treated root cells (), the concentric diffraction rings were detected at 3.68, 2.66, 2.23, and 1.67 Å, corresponding to the hlk planar of (012), (104), (113), and (116) of ɑ-Fe2O3 (hematite), according to the standard JCPDS 01-1053 [Citation42,Citation43]. Besides, the detection of the concentric diffraction rings at 4.82, 2.93, and 2.51 Å, attributed by the hlk planar of (111), (220), and (311) of Fe3O4 (magnetite) according to the standard JCPDS No. 01-1111 [Citation44,Citation45]. Similarly, in the samples treated with both Fe(NO3)3 and Ni(NO3)2 (), the concentric diffraction rings of 3.64, 2.23, 1.85, and 1.66 Å were marked, attributing to the hlk planar of (012), (113), (024), and (116) of ɑ-Fe2O3. Also, the concentric diffraction rings of 2.97, 2.54, 2.41, and 1.46 Å corresponded to the hlk planar of (220), (311), (222), and (440) of Fe3O4. In this work, the plant tissue sections of 100-nm thickness were analyzed by SAED-TEM so the crystallinity structures of metal NPs inside the cells could be determined. The analysis results suggested two structural forms of iron oxide NPs in the plant cells; ɑ-Fe2O3 (hematite) and Fe3O4 (magnetite) [Citation43,Citation45]. It was also noted that in the samples treated with Ni(NO3)2, both single and dual metal treatments, there was no detection of the characteristic diffraction rings of NiNPs, indicating no cellular formation of NiNPs.
Figure 7. SAED-TEM analysis of cellular NPs in A. pinnata treated with (A) Fe(NO3)3 and (B) Fe(NO3)3 and Ni(NO3)2.
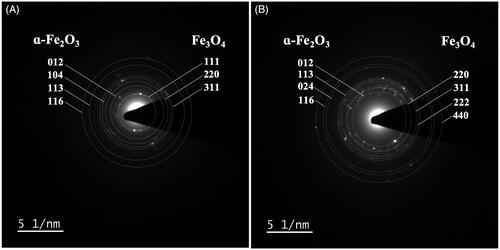
EDX analysis was also employed to elucidate the elemental composition in the root and shoots of A. pinnata (). In the plants treated with Fe(NO3)3, Fe element was highly detected in the plant roots and much less in the shoots. This result supported that root is a Fe basin in the plant. In the plant treated with Ni(NO3)2), the Ni content in the shoots was higher than that in the roots, suggesting the mobility of Ni to other parts of the plants. In the plant treated with both metals, the Fe content was also highly accumulated in the roots, whereas most Ni element was detected in the shoots. These results also supported the hypothesis that the suitable concentration of metal ions was a key factor for the induction of metal NPs. The Fe content in a range of 7.55−8.90% weight in A. pinnata roots was probably sufficient for the formation of FeNPs. In contrast, the Ni content in 0.16−0.20% weight in A. pinnata roots was inadequate for the production of NiNPs. It was noted about the detection of high carbon content in all sample analyses. For samples of <2 µM thickness, this carbon could attribute to the carbon adhesion tape [Citation46,Citation47]. However, in this work, the plant specimens were cross-sectioned into 100-µM thickness, so the detected carbon was probably due to the organic carbon of the plant samples [Citation48]. For Na, its detection was likely due to the trace of sodium phosphate buffer used in a sample preparation step.
SOD and GR activities in response to metal treatments
In this study, the oxidative stress in A. pinnata was determined by the activities of superoxide dismutase (SOD) and glutathione reductase (GR). SOD and GR were the enzymes that play significant roles in the antioxidant defense under an oxidative stress condition. shows the SOD and GR activities in roots and shoots of A. pinnata. The SOD and GR activities in the plants treated with Fe(NO3)3 and Ni(NO3)2, both single or dual metal treatments, were significantly higher than those in the control plants. Both enzyme activities were also higher in the roots than the shoots, which might relate to the different levels of metals in both tissues.
Figure 9. Activities of (A) superoxide dismutase and (B) glutathione reductase in roots and shoots of A. pinnata exposed to Fe(NO3)3 and Ni(NO3)2.
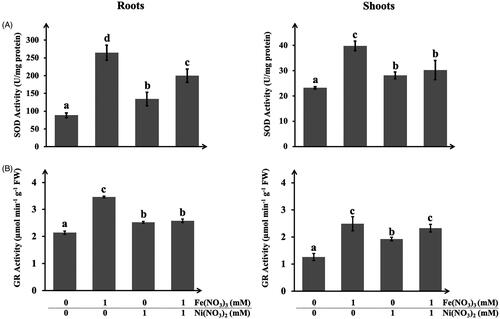
In roots, the SOD activity was the highest in the plant treated with Fe(NO3)3, followed by those treated with Ni(NO3)2 and both metals, respectively. These results well agreed with the previous experiments that roots were the prime sink site of iron ions. As plants are exposed to elevated concentrations of iron ions, they impose oxidative stress due to the high stimulation of free radical production. One of the mechanisms to reduce ROS toxicity is to alleviate the activities of antioxidant enzymes, such as SOD, to control the ROS levels in the cells [Citation49]. In general, SOD is considered the first-line antioxidant defense in plants, which can catalyze superoxide anion to hydrogen peroxide and oxygen. Interestingly, this work showed that plants treated with both Fe(NO3)3 and Ni(NO3)2 had lower SOD activity as compared with only Fe(NO3)3. It was likely due to the lesser content of iron ions causing by the uptake competition with nickel ions. The lowest SOD activity in the plants treated with Ni(NO3)2 was probably due to its lower concentration in the roots as nickel ions were more efficient to transport to other parts of the plants. This assumption was supported by the results of SOD activity in the shoots. The SOD activities in the plants treated with Ni(NO3)2 and both metals were not significantly different. It was noted that SOD activity in shoots was much lower than roots. These results were potentially due to the accumulated level of iron ions in shoots much lesser than roots.
For the GR activity, similar results were detected, in which the GR activity was higher in the metal-treated plants as compared with the controls. Also, the GR activity was the highest in the plants treated with Fe(NO3)3, followed by the plants treated with Ni(NO3)2 and both metals. The profiles of GR activity in both roots and shoots were similar. It was noted that SOD might be the key enzyme in metal-stress defense in the plant roots, as its activity was at least 6-folds higher than that in shoots. As SOD can function as a reducing agent, it was postulated to involve the formation of metal NPs in the plant cells, especially in roots. In contrast, the levels of GR activities in both roots and shoots were not highly different, suggesting its significant functions in both tissues. Nevertheless, the maximal activities of both enzymes in the plant roots were higher than those in the shoots. It was feasible due to the higher metal contents in the plant roots that caused more oxidative stress in these tissues [Citation29].
Conclusions
We report the new insight of different fates of the uptakes of Fe3+ and Ni2+ ions to transform into metal NPs inside the root cells of A. pinnata. The detection of FeNPs was near the cell wall and cell membrane and inside the vacuoles and multivesicular bodies. However, the formation of NiNPs was not detected even though the same molar concentration was used. According to the EDXRF and EDX analyses, the results suggested that the biogenesis of metal NPs depended on the sufficient concentration of metal ions in the cells. As the concentration of nickel ions was low in the roots, it was insufficient to induce the formation of NiNPs. Also, the SAED analysis confirmed the identity of cellular FeNPs as ɑ-Fe2O3 (hematite) and Fe3O4 (magnetite). These results reported the new finding that the formation of intracellular FeNPs in the plant cells might involve the reduction activity of the antioxidant enzymes, which was highly induced in the roots of the metal-treated plants. Also, the biogenesis of FeNPs in the plant roots depended on the sufficient concentration of the accumulated iron ions inside the cell.
Disclosure statement
No potential conflict of interest was reported by the author(s).
Data availability statement
The authors confirm that the data supporting the findings of this study are available within the article [and/or] its supplementary materials.
Additional information
Funding
References
- Raza A, Ikram M, Aqeel M, et al. Enhanced industrial dye degradation using Co doped in chemically exfoliated MoS2 nanosheets. Appl Nanosci. 2020;10(5):1535–1544.
- Ikram M, Khan MI, Raza A, et al. Outstanding performance of silver-decorated MoS2 nanopetals used as nanocatalyst for synthetic dye degradation. Physica E. 2020;124:114246.
- Ikram M, Tabassum R, Qumar U, et al. Promising performance of chemically exfoliated Zr-doped MoS2 nanosheets for catalytic and antibacterial applications. RSC Adv. 2020;10(35):20559–20571.
- Ikram M, Hassan J, Raza A, et al. Photocatalytic and bactericidal properties and molecular docking analysis of TiO2 nanoparticles conjugated with Zr for environmental remediation. RSC Adv. 2020;10(50):30007–30024.
- Ikram M, Umar E, Raza A, et al. Dye degradation performance, bactericidal behavior and molecular docking analysis of Cu-doped TiO2 nanoparticles. RSC Adv. 2020;10(41):24215–24233.
- Ikram M, Raza A, et al. Hydrothermal synthesis of silver decorated reduced graphene oxide (rGO) nanoflakes with effective photocatalytic activity for wastewater treatment. Nanoscale Res Lett. 2020;15(1):95.
- Ikram M, Hassan J, Imran M, et al. 2D chemically exfoliated hexagonal boron nitride (hBN) nanosheets doped with Ni: synthesis, properties and catalytic application for the treatment of industrial wastewater. Appl Nanosci. 2020;10(9):3525–3528.
- Ikram M, Ali S, Aqeel M, et al. Reduced graphene oxide nanosheets doped by Cu with highly efficient visible light photocatalytic behavior. J Alloys Compd. 2020;837:155588.
- Dauthal P, Mukhopadhyay M. Noble metal nanoparticles: Plant-mediated synthesis, mechanistic aspects of synthesis, and applications. Ind Eng Chem Res. 2016;55(36):9557–9577.
- Saravanan A, Kumar PS, et al. A review on biosynthesis of metal nanoparticles and its environmental applications. Chemosphere. 2021;264:128580.
- Jamkhande PG, Ghule NW, Bamer AH, et al. Metal nanoparticles synthesis: An overview on methods of preparation, advantages and disadvantages, and applications. J Drug Deliv Sci Technol. 2019;53:101174.
- Yadav VK, Khan SH, et al. Microbial synthesis of nanoparticles and their applications for wastewater treatment. In: Singh J, Vyas A, et al. editors. Microbial biotechnology: basic research and applications. Singapore: Springer Singapore; 2020. p. 147–187.
- Vargas G, Cypriano J, Correa T, et al. Applications of magnetotactic bacteria, magnetosomes and magnetosome crystals in biotechnology and nanotechnology: Mini-review. Molecules. 2018;23(10):2438.
- Reeves RD, Baker AJM, et al. A global database for plants that hyperaccumulate metal and metalloid trace elements. New Phytol. 2018;218(2):407–411.
- Corso M, García de la Torre VS. Biomolecular approaches to understanding metal tolerance and hyperaccumulation in plants. Metallomics. 2020;12(6):840–859.
- Yadi M, Mostafavi E, et al. Current developments in green synthesis of metallic nanoparticles using plant extracts: A review. Artif Cells Nanomed Biotechnol. 2018;46(sup3):S336–S43.
- Nasrollahzadeh M, Sajjadi M, et al. Pd-based nanoparticles: Plant-assisted biosynthesis, characterization, mechanism, stability, catalytic and antimicrobial activities. Adv Colloid Interface Sci. 2020;276:102103.
- Khodadadi B, Bordbar M, et al. Achillea millefolium L. extract mediated green synthesis of waste peach kernel shell supported silver nanoparticles: application of the nanoparticles for catalytic reduction of a variety of dyes in water. J Colloid Interface Sci. 2017;493:85–93.
- Kumar V, Kumar P, Singh J, et al. Potential of water fern (Azolla pinnata R.Br.) in phytoremediation of integrated industrial effluent of SIIDCUL, Haridwar, India: removal of physicochemical and heavy metal pollutants. Int J Phytoremediation. 2020;22(4):392–403.
- Talebi M, Tabatabaei BES, et al. Hyperaccumulation of Cu, Zn, Ni, and Cd in Azolla species inducing expression of methallothionein and phytochelatin synthase genes. Chemosphere. 2019;230:488–497.
- Sikirou M, Saito K, Dramé KN, et al. Soil-based screening for iron toxicity tolerance in rice using pots. Plant Prod Sci. 2016;19(4):489–496.
- Müller C, Kuki KN, Pinheiro DT, et al. Differential physiological responses in rice upon exposure to excess distinct iron forms. Plant Soil. 2015;391(1–2):123–138.
- Küpper H, Andresen E. Mechanisms of metal toxicity in plants. Metallomics. 2016;8(3):269–285.
- Hassan MU, Chattha MU, et al. Nickel toxicity in plants: reasons, toxic effects, tolerance mechanisms, and remediation possibilities—a review. Environ Sci Pollut Res. 2019;26(13):12673–12688.
- Emamverdian A, Ding Y, Mokhberdoran F, et al. Heavy metal stress and some mechanisms of plant defense response. Sci World J. 2015;2015:1–18.
- Deng T-H-B, van der Ent A, Tang Y-T, et al. Nickel hyperaccumulation mechanisms: a review on the current state of knowledge. Plant Soil. 2018;423(1–2):1–11.
- Nishida S, Kato A, et al. Induction of nickel accumulation in response to zinc deficiency in Arabidopsis thaliana. Int J Mol Sci. 2015;16(5):9420–9430.
- Andresen E, Peiter E, et al. Trace metal metabolism in plants. J Exp Bot. 2018;69(5):909–954.
- Ghori N-H, Ghori T, Hayat MQ, et al. Heavy metal stress and responses in plants. Int J Environ Sci Technol. 2019;16(3):1807–1828.
- Nandiyanto ABD, Oktiani R, Ragadhita R, et al. How to read and interpret FTIR spectroscope of organic material. Indonesian J Sci Technol. 2019;4(1):97–128.
- Khosravi Z, Kotula S, Lippitz A, et al. IR- and NEXAFS-spectroscopic characterization of plasma-nitrogenated polyolefin surfaces. Plasma Process Polym. 2018;15(1):1700066.
- Loupatty VD, Radiena MSY. Properties activity of yeast against seaweed bioethanol fermentation time and its functional group. J Phys Conf Ser. 2020;1463:012018.
- Hamadneh I, Alhayek H, et al. Green synthesis and characterization of yttrium oxide, copper oxide and barium carbonate nanoparticles using Azadirachta indica (the neem tree) fruit aqueous extract. Egypt J Chem. 2019;62(4):573–581.
- Muhammad I, Shalmani A, et al. Mechanisms regulating the dynamics of photosynthesis under abiotic stresses. Front Plant Sci. 2021;11: https://doi.org/https://doi.org/10.3389/fpls.2020.615942.
- Krzesłowska M. The cell wall in plant cell response to trace metals: Polysaccharide remodeling and its role in defense strategy. Acta Physiol Plant. 2011;33(1):35–51.
- Keunen E, Peshev D, et al. Plant sugars are crucial players in the oxidative challenge during abiotic stress: extending the traditional concept. Plant Cell Environ. 2013;36(7):1242–1255.
- Dutta S, Mitra M, et al. Oxidative and genotoxic damages in plants in response to heavy metal stress and maintenance of genome stability. Plant Signal Behav. 2018;13(8):e1460048.
- Kong J, Yu S. Fourier transform infrared spectroscopic analysis of protein secondary structures. Acta Biochim Biophys Sin. 2007;39(8):549–559.
- Khandel P, Yadaw RK, Soni DK, et al. Biogenesis of metal nanoparticles and their pharmacological applications: present status and application prospects. J Nanostruct Chem. 2018;8(3):217–254.
- Li G, Kronzucker HJ, et al. The response of the root apex in plant adaptation to iron heterogeneity in soil. Front Plant Sci. 2016;7(344):1–7.
- Adamakis I-DS, Eleftheriou EP. Structural evidence of programmed cell death induction by tungsten in root tip cells of Pisum sativum. Plants. 2019;8(3):62.
- Muraro PCL, Mortari SR, et al. Iron oxide nanocatalyst with titanium and silver nanoparticles: Synthesis, characterization and photocatalytic activity on the degradation of Rhodamine B dye. Sci Rep. 2020;10(1):3055.
- Sharma AK, Pawar CA, Prasad NR, et al. Antimicrobial efficacy of green synthesized iron oxide nanoparticles. Mater Res Express. 2018;5(7):075402.
- Khaghani S, Ghanbari D, et al. Green synthesis of iron oxide-palladium nanocomposites by pepper extract and its application in removing of colored pollutants from water. J Nanostruct. 2017;7(3):175–182.
- Wan D, Li W, Wang G, et al. Shape-controllable synthesis of peroxidase-like Fe3O4 nanoparticles for catalytic removal of organic pollutants. J Mater Eng Perform. 2016;25(10):4333–4340.
- Hassan J, Ikram M, et al. Application of chemically exfoliated boron nitride nanosheets doped with co to remove organic pollutants rapidly from textile water. Nanoscale Res Lett. 2020;15(1):75.
- Ikram M, Hussain I, Hassan J, et al. Evaluation of antibacterial and catalytic potential of copper-doped chemically exfoliated boron nitride nanosheets. Ceram. 2020;46(13):21073–21083.
- Titus D, James Jebaseelan Samuel E, et al. Chapter 12 - Nanoparticle characterization techniques. In: Shukla AK, Iravani S, editors. Green synthesis, characterization and applications of nanoparticles. : Elsevier; 2019. p. 303–319. Cambridge, MA, USA.
- Bhaduri AM, Fulekar MH. Antioxidant enzyme responses of plants to heavy metal stress. Rev Environ Sci Biotechnol. 2012;11(1):55–69.