Abstract
Objective
Studies of the human cochlea are particularly challenging due its exceptional vulnerability and surrounding hard bone. Swift fixation and mild decalcification are necessary to maintain its structural integrity and preserve antigenicity. Such procedures may allow immunohistochemistry, gene analyses, and molecular imaging using super resolution illumination microscopy (SR-SIM) with nanometer resolution.
Design
This presentation updates recent studies of the human cochlear microanatomy and immunohistochemistry by our laboratory, discussed in the context of current and past anatomic findings and the available literature.
Results
Human studies are necessary, and there are intriguing discrepancies compared with experimental animal studies, highlighting that “men are not simply big mice.” The results may improve our understanding of the function of the human hearing organ, the diseases related to it, and how this better understanding can be extended to impact future treatment.
Conclusion
The first human inner ear gene therapy trials are in progress, and the accessibility of human cochlear tissue for future stem cell treatment and gene transfer needs further elucidation.
Keywords:
Introduction
For many years, electron microscopy has been conducted to the study of the inner ear, initially with little emphasis on human anatomy [Citation1]. The first scanning electron microscopy (SEM) studies were published more than 50 years ago [Citation2,Citation3]. Spoendlin and Schrott-Fischer established techniques to obtain perilymph fixed human inner ear tissue suitable for transmission electron microscopy (TEM) [Citation4]. Techniques have also been developed to collect human cell material at surgery after ethical permission and patient consent [Citation5]. Well-preserved tissue can be acquired that is suitable for electron microscopy, immunohistochemistry, and super-resolution structured illumination microscopy (SR-SIM) and stimulated emission depletion microscopy (STED). These novel techniques offer the potential to image molecular organization with nanometre resolution and can provide information about the localization of ion channels, transporters, gene expression, and the constituents of the human cochlea. Tissue from individuals with preserved cochlear function (petroclival conditions) offers unique possibilities to detail the molecular organization under ‘normal’ settings.
The GJB2 encoding of connexin26 (Cx26) in gap junctions (GJs) plays a dominant role in human deafness, and studies of its molecular organization are relevant for future gene therapy. SR-SIM provides information about the arrangement of GJ plaques in the organ of Corti and lateral wall of the human cochlea. Human fine structure can differ from that of other species, and this has motivated further studies in man, again highlighting that ‘men are not simply big mice.’ This is particularly pertinent to the auditory nerve and endolymphatic duct and sac where we see extensive interspecies differences. For a more detailed description of methods and techniques used, the reader is referred to recent publications [Citation6].
The human organ of corti
Cell contractility
Each human hearing organ contains around 15,000 sensory hair cells (). Approximately 3,400 are inner hair cells (IHCs) that are clearly wired to the brain and 12,500 are outer hair cells (OHCs). Hearing is a mechanically active process and OHCs are endowed with a unique transmembrane protein called prestin [Citation8], which is also expressed selectively in the basolateral plasma membrane of human OHCs [Citation7] (, insets). It is responsible for the electro-motility that is the driving force behind the somatic motor of the cochlear amplifier. It may overcome the impedance of surrounding fluid and improves auditory sensitivity and frequency selectivity particularly at high frequencies.
Figure 1. Field emission scanning electron microscopy (FE-SEM) of a left human hemi-sectioned cochlea with estimated frequency locations. The specimen was coated with gold–palladium with approx. 2 nm resolution. The hearing organ at 0.5–1 kHz is shown in upper right corner. In lower insets, OHCs at low frequency display beaded efferent nerve terminals high up on their shafts (arrows). These neurons express voltage-gated sodium channel NaV1.6 (red staining). Decalcification in Na-EDTA. OHCs: outer hair cells. *Apical end of tectorial membrane. Upper and lower insets photos reproduced with permission for audiological medicine [Citation7].
![Figure 1. Field emission scanning electron microscopy (FE-SEM) of a left human hemi-sectioned cochlea with estimated frequency locations. The specimen was coated with gold–palladium with approx. 2 nm resolution. The hearing organ at 0.5–1 kHz is shown in upper right corner. In lower insets, OHCs at low frequency display beaded efferent nerve terminals high up on their shafts (arrows). These neurons express voltage-gated sodium channel NaV1.6 (red staining). Decalcification in Na-EDTA. OHCs: outer hair cells. *Apical end of tectorial membrane. Upper and lower insets photos reproduced with permission for audiological medicine [Citation7].](/cms/asset/e2c91f54-c221-40c1-adeb-5fa5cfbb9dac/ihbc_a_1807259_f0001_c.jpg)
Figure 2. FE-SEM of the human organ of Corti at the transition zone between the apical and middle turns of the opposed hemi-section in (area 4). The acoustic ridge is approximately 0.2 mm wide. Insets show coexpression of parvalbumin (green) and prestin (red) in OHCs (red). Cell nuclei are blue. OHC: outer hair cell; IHC: inner hair cell; IPC: inner pillar cell; OPC: outer pillar cell; TCF: tunnel crossing fibres; TSB: tunnel spiral bundle; DC: deiters cell; HS: Hensen cell; BM: basilar membrane. Original photo from Anatomical Record 2012. Published with permission provided by John Wiley and Sons and Copyright Licence Number 4821821134443. Inset photo reproduced with permission for Audiological Medicine.
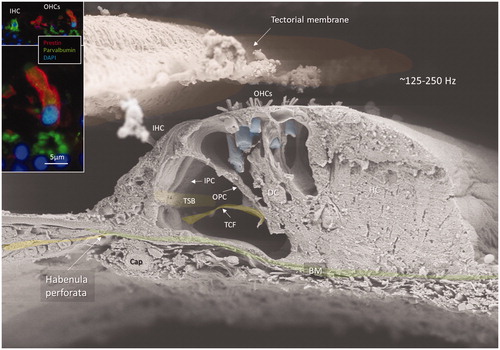
Contractile mechanisms along microtubule bundles seem to be of importance for active vibratory responses during hearing, in animals as well as in humans. Inner and OHCs stereocilia and cuticular plates contain contractile elements such as actin together with an intricate cross-linking molecular machinery whose organization is still poorly understood [Citation9]. Actin filaments and microtubules can crosslink in OHCs [Citation10,Citation11]. Contractile proteins were described in supporting cells in guinea pigs by Flock et al. [Citation12] and were noted to be giving stability to the cells. They contain non-muscle β- and γ actin isoforms and α-actinin [Citation13,Citation14] whereas the cuticular plate contains actin, α-actinin, myosin, tropomyosin, spectrin, profilin and fodrin [Citation10,Citation15]. Non-muscle actin subunits are present in human Deiters cells, IPCs and OPCs and contain a remarkable three-dimensional (3D) interacting skeletal system of actin strands and microtubules anchored to the plasma cell membranes (). Opposing pillars appear to be coupled with functional elements to relay basilar membrane (BM) vibrations to the reticular lamina (RL) and sensory hair cells that have no direct contact with the BM (). In humans, TEM shows a dense meshwork associated with cell junctional complexes at the plasma membrane referred to as surfoskelosomes [Citation13]. These are located in the pillar heads and foot (basal bodies) that contain organized actin and are closely associated with microtubules [Citation16]. Deiters’ cells also express organized actin in basal bodies. Border and phalangeal cells around the IHCs do not express cytoplasmic actin possibly since they are positioned on a rigid fundament operative as principal sensory receptors.
Figure 3. (A) TEM of pillar head surfoskelosomes with actin and associated microtubules. Inset shows framed area and a GJ in higher magnification (GJ width is 120 nm and intercellular distance 10–15 nm). OPS: outer pillar cell and IPC: inner pillar cell. (B) SR-SIM shows actin distribution in the pillar cells. (C) Higher magnification of IHC stereocilia and cuticle (inset) which are positive for actin and transmembrane serine protease 3 (TMPRSS3). (D) TEM of actin conglomerates and associated microtubules in pillars. (E) TEM of OHC rows. (F) Pillar cell connects to the first row OHC.
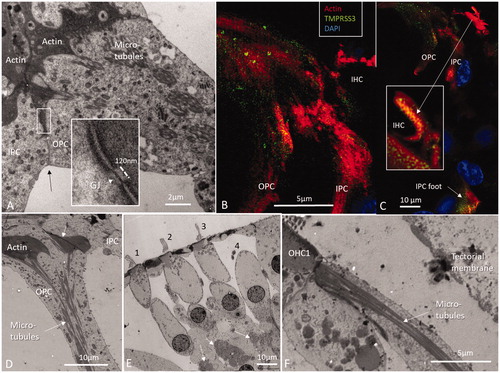
Figure 4. (A) Illustration showing actin and the associated protein TMPRSS3 in the human organ of Corti and their role in cell motility. The proteins co-localize in hair cell stereocilia, cuticles and membrane-associated regions in pillar and Deiters’ cells called surfoskelosomes. In supporting cells, the surfoskelosomes are connected via a system of microtubules. The image on the right shows the putative organization of microtubule–actin at the anchorage to the BM. Here, microtubules also run parallel with the contractile apparatus. They may act as load-bearing spring coils controlling contraction–relaxation of the polarized network during oscillatory vibrations with long-lasting mechanical properties. Microtubules are anchored against the BM through filamentous connections at focal adhesions. Illustration by Karin Lodin.
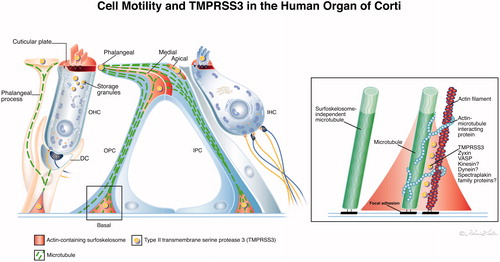
Cx30 for a ‘cochlear symphony’
Both Connexin30 (Gjb6) and Connexin26 (Gjb2)-deficiencies cause severe hearing loss and lack of endo-cochlear potential (EP) [Citation17–19]. Most supporting cells show a large number of GJs in man. They allow passage of small substances (<1.5 kDa) [Citation20,Citation21] and ions but can also electrically couple cells to coordinate activity of larger cell groups. Remarkably, a large number of cx30 expressing GJs are present both alongside adjacent and opposite pillars [Citation5]. GJ plaques are present between human pillar heads as demonstrated with super resolution immunohistochemistry and TEM shown in (inset). This suggests that the pillars are electrically coupled and coordinate motor activity of larger cell groups in the human organ. The dynamic components of supporting cells may modulate mechanical vibrations from the BM via the microtubule–actin system along the cellular shafts to the RL and hair cells. Microtubules are anchored through filamentous connections at focal adhesions (). Microtubules also run independently of the basal surfoskelosome, which is of significance for contraction–relaxation of the polarized network during oscillatory vibrations. According to Schick et al. [Citation22], vasodilator-stimulated phosphoprotein (VASP) is believed to regulate actin dynamics, motility, and cell adhesion, together with zyxin. These authors believed that actin-based dynamics in the pillars control longer-lasting mechanical properties contrary to the faster cilia action.
Actin and TMPRSS3—is the human cochlea also electrically tuned?
An intriguing association was found between actin and the enzyme TMPRSS3, a type II trans-membrane serine protease, [Citation16]. Several mutations in the TMPRSS3 gene on chromosome 21q22.3 cause autosomal recessive non-syndromic deafness (DFNB8/10) with varied phenotypic expression [Citation23,Citation24]. A proposed substrate is the potassium channel Kca1.1, known to be essential for electric tuning in lower animals. In situ hybridization shows that TMPRSS3 mRNA is localized in sensory hair cells in the cochlea, and patch-clamp and proteomic analyses reveal that the outward-rectifying K+ currents are altered in mutant mice. This was confirmed by immunohistochemistry showing that the channels are absent at the neck of IHCs in TMPRSS3-mutant mice [Citation25]. These studies motivated us to analyse if the enzyme is also localized in the human cochlea. Such a finding may improve our understanding of its biological roles, mechanic-electric transduction and connection to ear disease. Variable expression along the cochlear spiral could explain variances in channel conductance and even frequency filtering. We found that actin was strongly expressed in association with TMPRRS3 in stereocilia and cuticular plates of IHCs and OHCs (). TMPRRS3 and actin were jointly expressed in electron-dense regions of the pillar head and foot associated with cell membrane specializations. These findings suggest that the proteolytic enzyme plays a major role in the actin networks for cilia-related hair cell mechanics and support cell contractility (). Our study could not verify with certainty if KCa1.1 channels are the TMPRSS3 substrate related to actin activity. Electric tuning has generally been denied in mammalian cochlear hair cells [Citation26]. More knowledge of the function of this protease and its domains and Cx interaction is necessary in order to obtain a complete representation of its roles in human hearing.
The human tectorial membrane
The human tectorial membrane (TM) is an acellular matrix overlying the cochlear hair cells. The wedge effectuate cilia deflection but recent research indicates that it plays an even more active role in auditory transmission than earlier believed. TM travelling waves have been documented, explaining that the TM’s variable radial stiffness and longitudinal coupling may contribute to the electromotor responses of OHCs [Citation27,Citation28]. The TM was studied in several animal models over the years but there have been relatively few investigations in humans [Citation29–33].
Human TM fibres express collagen type II. Also collagen V, IX and XI are expressed and three unique non-collagenous glycoproteins α-tectorin, β-tectorin and otogelin constitute half of the proteins in the TM [Citation34–36]. TEM shows that fibres are embedded in the jelly-like matrix of α-tectorin and β-tectorin where the latter is believed to shape Hensen´s stripe and the peripheral or marginal net [Citation28]. No β-tectorin protein immunoreactivity could be verified in our study, presumably due to staining failure. We identified α-tectorin secreted by the interdental cells (IDCs) that were incorporated into the TM ().
Figure 5. Artist´s drawing of the human spiral limbus and the proposed secretory pathway and uptake of macromolecular substances into the TM. (A) Secretory vesicles (coated pits) coalesce with the apical membrane of the IDCs (lilac) partly submerged in the spiral prominence (lower left TEM). (B) Its content empties into the sub-tectorial space and is taken up by the TM. The jelly-like matrix is comprised of various glycoproteins such as β- and α-tectorin and otogelin and is incorporated into the limbus zone of the TM in close association with the collagen II fibres [Citation37]. (C) Imprints of OHC stereocilia tips on the undersurface tectorin layer. (D) SEM image of the undersurface of the TM. Our SEM study did not show cilia imprints on Hensen’s stripe where the tips of the IHC stereocilia seem to attach. The drawing was done by Karin Lodin. Inset D is from ‘Molecular Organization and Fine Structure of the Human Tectorial Membrane: is It replenished?’ by Hisamitsu Hayashi et al., Cell and Tissue Research, June 18, 2015 [Citation33]. It is used here with permission from Springer Nature, licence and terms provided by Springer Nature and Copyright Clearance Centre, licence number 4821850025140.
![Figure 5. Artist´s drawing of the human spiral limbus and the proposed secretory pathway and uptake of macromolecular substances into the TM. (A) Secretory vesicles (coated pits) coalesce with the apical membrane of the IDCs (lilac) partly submerged in the spiral prominence (lower left TEM). (B) Its content empties into the sub-tectorial space and is taken up by the TM. The jelly-like matrix is comprised of various glycoproteins such as β- and α-tectorin and otogelin and is incorporated into the limbus zone of the TM in close association with the collagen II fibres [Citation37]. (C) Imprints of OHC stereocilia tips on the undersurface tectorin layer. (D) SEM image of the undersurface of the TM. Our SEM study did not show cilia imprints on Hensen’s stripe where the tips of the IHC stereocilia seem to attach. The drawing was done by Karin Lodin. Inset D is from ‘Molecular Organization and Fine Structure of the Human Tectorial Membrane: is It replenished?’ by Hisamitsu Hayashi et al., Cell and Tissue Research, June 18, 2015 [Citation33]. It is used here with permission from Springer Nature, licence and terms provided by Springer Nature and Copyright Clearance Centre, licence number 4821850025140.](/cms/asset/8e81c8ec-f6ed-4abe-a8d7-a54e96778ec3/ihbc_a_1807259_f0005_c.jpg)
Is the tectorial membrane replenished?
A renewal was earlier proposed in adults [Citation38]and during development [Citation39]. According to Prieto et al., two secretion products could be involved in the turnover of the adult TM and/or secretion of some components. [Citation40] In humans, α-tectorin seems to be translocated along the collagen fibres to reach the surfaces. It was more expressed in the high-frequency region and in the outer circumference of the TM. This may explain why some α-tectorin (TECTA) gene mutations cause more hearing loss at high frequencies [Citation41]. Human α-tectorin underlies two dominantly inherited non-syndromic deafnesses, DFNA8 and DFNA12 [Citation42,Citation43]. Targeted gene disruption shows the intricate roles of α-tectorin and β-tectorin for OHCs to optimally stimulate the IHCs [Citation44,Citation45].
Human tectorial membrane may influence the characteristic frequency of the BM
The human TM consists of a partially smooth-surfaced medial portion overlying the spiral limbus (limbus zone), while a larger lateral part consists of a middle and marginal zone (). They are covered by a radial net with fibrous elements. The medial part overrides an amorphous substance that was not visible in mice in our studies. The TM contains a rich meshwork of thin fibrils that undergoes a morphological change along the cochlear spiral. Its size increases from base to apex. Its width and thickness increase to the upper middle turn. The undersurface shows prominent features, such as Hensen’s stripe and a marginal band, with which IHCs and OHCs are associated. In the base, the marginal band is solid and robust, suggesting a larger mass load. In the middle turn, the marginal band facing the OHCs is delicate and contains fine struts with imprints of the interacting fourth row of the variable OHC bundles. This arrangement suggests an exquisite and gradual sensitivity to mechanical ambiances. The fibril density and diameter of the radial collagen bundles decreased from base to apex. This may influence TM stiffness, which confirms experiments showing that it changes longitudinally from base to apex with graded viscoelasticity and porosity [Citation46–48]. At the hair cells, though, TM stiffness seems to gradually increase in the longitudinal direction [Citation49] and this may be related to differences in collagen arrangement in the marginal zone as viewed with SEM.
How are inner and OHCs coupled?
The undersurface displays radial fibre bundles running from the marginal band to Hensen’s stripe with direct connections. An intriguing observation is that vibrations are conveyed directly from OHCs to IHCs via functional radial fibres that couple the two hair cell systems. Similarly, radial coupling might synchronize equally tuned OHCs to enhance sensitivity and frequency resolution [Citation50]. However, SEM showed no cilia imprints on Hensen’s stripe, similar to earlier reports [Citation31,Citation51] suggesting that IHC bundles are freestanding, although physical contact could occur during BM motion [Citation29]. In another human SEM study, imprints of IHC bundles were found in the basal turn but not in the apical and middle turns [Citation30]. Another option for the IHCs to be activated is through the sub-tectorial fluid. At some frequencies the TM and RL oscillations influence the sub-tectorial fluid space that, in itself, could couple OHCs and IHCs [Citation52]. We also localized the inner ear-specific protein otoancorin expressed on the spiral limbus and believed to be indispensable for TM attachment to the spiral limbus [Citation44,Citation53]. The gene OTOA encoding the protein was found to be mutated in non-syndromic recessive deafness DFNB22.
The lateral cochlear wall—an ion machinery and electric battery
There has now been more focus directed to the cochlear lateral wall since isolated changes can be associated with sensorineural hearing loss (SNHL). Age-related changes may be caused by a “metabolic presbycusis” often characterised by flat auditory thresholds [Citation54]. This tissue contains fibrocytes (types I–V) tightly associated with the stria vascularis (StV). It is responsible for the generation of the high DC potential and endolymph K+ concentration essential for hair cell transduction. Bekesy described the potential already in 1952 [Citation55], and due to its anoxia sensitivity it was suggested it originates in the StV with its rich vascular supply. The essential roles played by the fibrocytes were also shown by their structural and metabolic association with StV atrophy in aged gerbils and in models of hereditary deafness [Citation56,Citation57].
The DC potential is explained by a ‘two-cell model’ as suggested by Salt et al. [Citation58] and by the updated five-compartment model, where the StV is separated by four membranes [Citation59,Citation60]. The basal cells (BCs) form a tight junction (TJ) barrier between the intrastrial space and an electrical syncytium represented by type I fibrocytes, BCs, intermediate cells (ICs) and endothelial cells. They seem to play a crucial role in avoiding a short circuit to perilymph and are heavily connected with GJs (Supplementary Figure 1). In vivo studies suggest that the endo-cochlear potential (EP) stems primarily from two K+ diffusion potentials situated at the apical membrane of the marginal cells (MCs) and the medial surface of the ICs (Kir4.1). K+ seems to be transported from the intrastrial space into MCs via basolateral Na/K-ATPase and NKCC1 (Supplementary Figures 2 and 3) and is secreted into the endolymph via apical KCNQ1/KCNE1 channels [Citation61,Citation62] ( and ). SR-SIM in humans confirms this arrangement of ion channels and transporters, including extensive and selective expressions of Na/K-ATPase, a rich number of intercellular GJs, and a TJ network. SR-SIM shows that a Claudin-11 TJ protein insulates the StV presumably to preserve ion gradients with Cx26 and Cx30 GJs separately expressed in homomeric/homotypic arrangements (Supplementary Figure 1). TJ protein restricts para-cellular ion flow and deficiency may open the intrastrial space, eliminate isolation, and result in a reduced EP and hearing loss. Claudin-11 null (Cld11−/−) mice exhibit severe deafness and a low EP but maintain K+ concentration. TJs disappeared from BCs and the barrier was destroyed without affecting the MC barrier. Findings suggest that the BC barrier is indispensable for hearing through the generation/maintenance of EP [Citation63,Citation64]. Clinically, several conditions could be with barrier disruption, including hereditary factors, inflammation, infections and autoimmunity, inner ear surgery and trauma ( and ).
Figure 6. (A) Principal drawing of the lateral wall of the human cochlea and super-resolution microscopy. The epithelial layer consists of MCs, ICs, and BCs and is associated with spiral ligament fibrocytes classified into five different categories of I–V. Type I forms a syncytium with the BCs and ICs. Cx26 and cx30 are believed to be essential for ion circulation and recirculation via the outer sulcus and root cells. Apical epithelial TJs express occludin. (B) Image of the lateral insertion of the Reissner’s membrane (RM) at the StV and suprastrial region. The basolateral cell membranes of the MCs express Na/K-ATPase and NKCC1. (C) BCs express insulating lamellae (arrow) expressing TJ protein claudin-11. (D) Framed area in B is shown in higher magnification with expression of the ion transporters Na/K-ATPase and NKCC1. Illustration by Karin lodin.
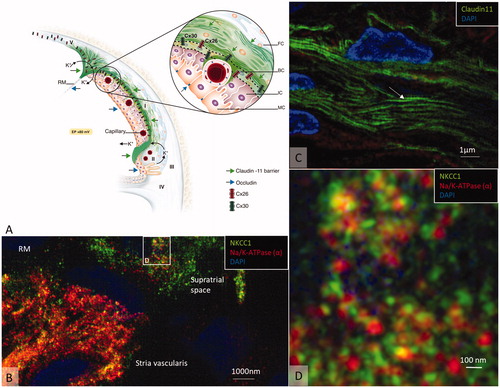
Figure 7. Hypothetical model of the generation of the EP. The green area represents the insulating area expressing claudin-11 TJ. A ‘two-cell model’ was presented by Salt et al. (1987) with ICs, BCs, and fibrocytes (FCs) on one side forming a syncytium and MCs on the other. The syncytium contains ‘gap/tight’ junctions (semiconductors) and the inward rectifying potassium channels Kir4.1 relaying K+ into the intrastrial space. Na/K-ATPase is forcefully expressed in the basolateral MCs, and secretion of K+ into the endolymph is via KCNQ1/KCNE1. Na/K-ATPase could not be detected in type I fibrocytes, but it was in types II and V cells in this study. They are believed to be involved in a recirculation of ions to type I fibrocytes through GJs. Conceivable factors weakening the EP are listed. Illustration by Karin Lodin.
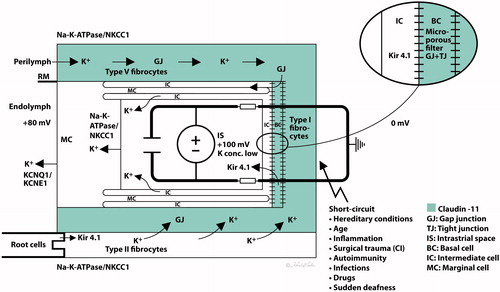
An important role for the EP generation is played by the ICs, expressing the inwardly rectifying K+ channel Kir4.1 [Citation65]. Ba2+-sensitive Kir4.1 channels sets the membrane potential in the ICs [Citation66,Citation67] via regulation of K+ diffusion. Deletion of the gene causes deafness with diminished K+ secretion and loss of the EP [Citation68]. We found Kir4.1 expressed in the intermediate and BC layers often jointly with Cx26 at the BCs (Supplementary Figure 4). If SNHL caused by GJB2 gene disruption depends on a functional linkage between the two channels needs further clarification. In humans, TEM shows the intricate cell labyrinths (BC) expressing both GJs and TJs [Citation69]. It noticeably permits the transcellular flow of ions through Cx26 and Cx30 GJs but equally restricts para-cellular flow through the TJs [Citation59,Citation70,Citation71]. Claudin-11 TJ/GJ cells may act as dual conductor-isolators (semi-conductors) to selectively mediate movement of charged units analogous to a conventional electrochemical ‘battery’. GJs may act like wires in an electric circuit, accomplishing the movement of charged units and membrane capacitance of the basal surface [Citation72]. Thus, our findings may add further understanding of the generation of EP and the role played by claudin-11 in the human cochlea.
The human auditory nerve
Well-preserved nerve tissue for TEM is difficult to obtain but may give us critical information about the function of the human auditory nerve. Unusually, spiral ganglion cell bodies in humans remain after dendrite degeneration. It is momentous for patients receiving cochlear implants (CI). In patients with progressive adult-onset hearing loss, such as presbyacusis, neurons may be conserved even after long duration of deafness. The reason for the robustness may be inherent biological differences, pattern of myelination, vascular supply and/or particular trans-cellular signaling [Citation73,Citation74]. Human ganglion cells consist of large type 1 cells innervating the IHCs and fewer small type 2 cells innervating the OHCs. Axo-somatic synaptic contacts exist on both cell bodies related to the olivocochlear efferents [Citation75].
Human spiral ganglion cells are extraordinarily shaped
More than a century ago, researchers found that even the bodies of the vestibular and cochlear ganglia were surrounded by a myelin sheath. However, this does not apply to humans and shows the difference in myelin expression in human and pig. In humans there is even membrane zones between type I cell bodies with no separating glia layer, with both symmetric and asymmetric soma-somatic membrane connections [Citation76,Citation77]. Such physical interaction seems to be unique to humans, but the physiological significance remains unknown.
Figure 8. Expression of myelin basic protein (MBP) in the spiral ganglion cell soma in human (A) and pig (B). The axonal initial segments also frequently lack MBP expression in man. Cell perikarya in the pig are completely surrounded by compact myelin (arrow).
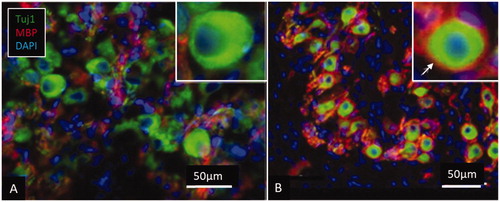
Cx43-expressing GJs are found between satellite glial cells that may play a role in cell preservation following dendrite loss [Citation74]. They have the capacity to transfer miRNA between cells and can regulate gene activity through genetic communication and thereby synchronise cell proliferation and differentiation [Citation78]. They can be responsible for glial cell reorganization and dynamics by regulating gene expression in neighbouring cells to re-establish surrounding protecting cell coat and arrest further retrograde degeneration. shows clustered human spiral ganglion cells fixed directly in glutaraldehyde where some cells lie ‘tete-a-tete.’ displays a lack of myelin basic protein (MBP) around cell soma and axonal initial segments. This represents normal human inner ear tissue, and the morphology does not seem to be explicated by any ear pathology or age-related changes. Lack of myelin reduces the speed of electric signals across the ganglion but may, on the other hand, allow nerve signals to be processed and influence nerve acuity. demonstrates expression of Na/K-ATPase-beta1 in the cell coat of the neuronal bodies and axons that re-establishes membrane potential after depolarisation. A similar expression is found in other mammals.
Figure 9. (A) Semi-thin section of a human spiral ganglion from the first turn of the cochlea. Some cell bodies have no separating satellite cell (*). (B) RNA-scope verified gene localization of both β1 and β3 Na/K-ATPase isoforms in the cell soma. These cells also express α3 isoform [Citation6]. (C) Cell bodies and axons express Na/K-ATPase β1 isoform. (D) SR-SIM shows staining of the β1 Na/K-ATPase in cell membranes of opposing cells.
![Figure 9. (A) Semi-thin section of a human spiral ganglion from the first turn of the cochlea. Some cell bodies have no separating satellite cell (*). (B) RNA-scope verified gene localization of both β1 and β3 Na/K-ATPase isoforms in the cell soma. These cells also express α3 isoform [Citation6]. (C) Cell bodies and axons express Na/K-ATPase β1 isoform. (D) SR-SIM shows staining of the β1 Na/K-ATPase in cell membranes of opposing cells.](/cms/asset/642e7a36-0cd2-476e-b3b0-57a7553802d9/ihbc_a_1807259_f0009_c.jpg)
Where are spike generators located?
Before a more comprehensive model of human auditory nerve excitation can be made, more information is needed about the distribution of gated ion channels, Ranvier nodes and spike generators. Current observations raise the possibilities that unique local electrical circuits may alter response characteristics among ganglion cells to code information through synchrony of their APs. This could be implemented to filter, sensitize and broaden signal transfer in the human auditory nerve. Nevertheless, significant species differences in microanatomy remain unexplained and captivating. SR-SIM showed surprisingly a double staining of the β1 and β3 subunits of Na/K-ATPase in the same cell (). Its significance remains obscure but the findings were verified with high sensitivity in situ hybridization RNA scope to localize both genes encoding β1 and β3 Na/K-ATPase isoforms (). Co-expression of the β1 isoform with both α2 and β2 isoforms in the same cells was earlier noted in human brain sections [Citation79]. Notably, β-subunits may function independently of Na/K-ATPase activity and may be involved in cell–cell adhesiveness [Citation80]. Obviously, more information of pump/channel/gene expressions and their sub-cellular distribution may further widen our understanding of the nerve transmission. More information could further empower the development of CI, one of the major advancements in modern medicine.
Ethical approval
The study conforms to The Declaration of Helsinki and was approved by the Medical Ethics Committee at the Uppsala University Hospital (No. C254/4, C45/7 2007, No. 99398, 22/9 1999, cont., 2003, Dnr. 2013/190). Patients’ consent for their participation was obtained.
ihbc_a_1807259_sm4293.docx
Download MS Word (996.6 KB)ihbc_a_1807259_sm0885.tif
Download TIFF Image (2.2 MB)ihbc_a_1807259_sm0864.tif
Download TIFF Image (3.8 MB)ihbc_a_1807259_sm0880.tif
Download TIFF Image (1.4 MB)ihbc_a_1807259_sm0883.tif
Download TIFF Image (3.7 MB)Acknowledgements
We also acknowledge the kind donations of private funds by Arne Sundström, Sweden. We are grateful to SciLife Laboratories and the BioVis Platform at the Uppsala University for providing SR-SIM microscope equipment and personal support throughout the study. The project was supported by Medel Inc. Austria under the agreement and contract with the Uppsala University. This research has been financially supported by the funding programs of the ‘K-regio project eVITA’ (electrical Vestibular Implant Tirol Austria) sponsored by EFRE (Dieses Projekt wird aus Mitteln des Europäischen Fonds für regionale Entwicklung kofinanziert, www.efre.gv.at). The Austrian Science Fund FWF Austria, project I 4147-B (Modelling electrical stimulation of the human cochlear nerve), also supported this research. We gratefully thank the Austrian Science Fund (FWF) for project funding Gapless Man: Machine Interface (Project No. I-3154-B27). We thank Karin Lodin for skillful artwork.
Disclosure statement
The authors declare that the research was conducted in the absence of any commercial or financial relationships that could be construed as a potential conflict of interest.
Additional information
Funding
References
- Engström H, Sjöstrand FS. The structure and innervation of the cochlear hair cells. Acta Otolaryngol. 1954;44(5–6):490–501.
- Lim DJ. Three dimensional observation of the inner ear with the scanning electron microscope. Acta Otolaryngol Suppl. 1969;255:1–38.
- Bredberg G, Lindeman HH, Ades HW, et al. Scanning electron microscopy of the organ of corti. Science. 1970;170(3960):861–863.
- Spoendlin H, Schrott A. The spiral ganglion and the innervation of the human organ of corti. Acta Otolaryngol. 1988;105(5–6):403–410.
- Liu W, Li H, Edin F, et al. Molecular composition and distribution of gap junctions in the sensory epithelium of the human cochlea—a super-resolution structured illumination microscopy (SR-SIM) study. Ups J Med Sci. 2017;122(3):160–170.
- Liu W, Luque M, Glueckert R, et al. Expression of Na/K-ATPase subunits in the human cochlea: a confocal and super-resolution microscopy study with special reference to auditory nerve excitation and cochlear implantation. Ups J Med Sci. 2019;124(3):168–179.
- Rask-Andersen H, Liu W, Boström M, et al. Audiological Medicine Immunolocalization of prestin in the human cochlea Immunolocalization of prestin in the human cochlea. Audiol Med. 2010;8(2):56–62.
- Zheng J, Shen W, He DZ, et al. Prestin is the motor protein of cochlear outer hair cells. Nature. 2000;405(6783):149–155.
- Pollock LM, Gupta N, Chen X, et al. Supervillin is a component of the hair cell’s cuticular plate and the head plates of organ of Corti supporting cells. PLoS One. 2016;11(7):e0158349.
- Slepecky NB, Ulfendahl M. Actin-binding and microtubule-associated proteins in the organ of Corti. Hear Res. 1992;57(2):201–215.
- Griffith LM, Pollard TD. Evidence for actin filament-microtubule interaction mediated by microtubule-associated proteins. J Cell Biol. 1978;78(3):958–965.
- Flock Å, Bretscher A, Weber K. Immunohistochemical localization of several cytoskeletal proteins in inner ear sensory and supporting cells. Hear Res. 1982;7:75–89.
- Henderson CG, Tucker JB, Mogensen MM, et al. Three microtubule-organizing centres collaborate in a mouse cochlear epithelial cell during supracellularly coordinated control of microtubule positioning. 1995:108(Pt 1):37–50.
- Slepecky NB, Savage JE. Expression of actin isoforms in the guinea pig organ of Corti: muscle isoforms are not detected. Hear Res. 1994;73(1):16–26.
- Mahendrasingam S, Furness D, Hackney C. Ultrastructural localisation of spectrin in sensory and supporting cells of guinea-pig organ of Corti. Hear Res. 1998;126(1–2):151–160.
- Liu W, Löwenheim H, Santi PA, et al. Expression of trans-membrane serine protease 3 (TMPRSS3) in the human organ of Corti. Cell Tissue Res. 2018;372(3):445–456.
- Kelsell DP, Dunlop J, Stevens HP, et al. Connexin 26 mutations in hereditary non-syndromic sensorineural deafness. Nature. 1997;387(6628):80–83.
- del Castillo I, Villamar M, Moreno-Pelayo MA, et al. A deletion involving the connexin 30 gene in nonsyndromic hearing impairment. N Engl J Med. 2002;346(4):243–249.
- Teubner B, Michel V, Pesch J, et al. Connexin30 (Gjb6)-deficiency causes severe hearing impairment and lack of endocochlear potential. Hum Mol Genet. 2003;12(1):13–21.
- Harris LA. Emerging issues of connexin channels: biophysics fills the gap. Q Rev Biophys. 2001;34(3):325–472.
- Jagger DJ, Forge A. Compartmentalized and signal-selective gap junctional coupling in the hearing cochlea. J Neurosci. 2006;26(4):1260–1268.
- Schick B, Praetorius M, Eigenthaler M, et al. Expression of VASP and zyxin in cochlear pillar cells: indication for actin-based dynamics?. Cell Tissue Res. 2003;311(3):315–323.
- Guipponi M, Vuagniaux G, Wattenhofer M, et al. The transmembrane serine protease (TMPRSS3) mutated in deafness DFNB8/10 activates the epithelial sodium channel (ENaC) in vitro. Hum Mol Genet. 2002;11(23):2829–2836.
- Fasquelle L, Scott HS, Lenoir M, et al. Tmprss3, a transmembrane serine protease deficient in human DFNB8/10 deafness, is critical for cochlear hair cell survival at the onset of hearing. J Biol Chem. 2011;286(19):17383–17397.
- Molina L, Fasquelle L, Nouvian R, et al. Tmprss3 loss of function impairs cochlear inner hair cell Kcnma1 channel membrane expression. Hum Mol Genet. 2013;22(7):1289–1299.
- Mammano F, Ashmore JF. Differential expression of outer hair cell potassium currents in the isolated cochlea of the guinea-pig. J Physiol. 1996;496(3):639–646.
- Ghaffari R, Aranyosi AJ, Freeman DM. Longitudinally propagating traveling waves of the mammalian tectorial membrane. Proc Natl Acad Sci USA. 2007;104(42):16510–16515.
- Richardson GP, Lukashkin AN, Russell IJ. The tectorial membrane: one slice of a complex cochlear sandwich. Curr Opin Otolaryngol Head Neck Surg. 2008;16(5):458–464.
- Lim DJ. Fine morphology of the tectorial membrane. Its relationship to the organ of corti. Arch Otolaryngol. 1972;96(3):199–215.
- Hoshino T. Contact between the tectorial membrane and the cochlear sensory hairs in the human and the monkey. Arch Otorhinolaryngol. 1977;217(1):53–60.
- Kawabata I, Nomura Y. Extra internal hair cells. A scanning electron microscopic study. Acta Otolaryngol. 1978;85(5–6):342–348.
- Rask-Andersen H, Liu W, Erixon E, et al. Human cochlea: anatomical characteristics and their relevance for cochlear implantation. Anat Rec. 2012;295(11):1791–1811.
- Hayashi H, Schrott-Fischer A, Glueckert R, et al. Molecular organization and fine structure of the human tectorial membrane: is it replenished? Cell Tissue Res. 2015;362(3):513–527.
- Richardson GP, Russell IJ, Duance VC, et al. Polypeptide composition of the mammalian tectorial membrane. Hear Res. 1987;25(1):45–60.
- Thalmann I, Thallinger G, Comegys TH, et al. Composition and supramolecular organization of the tectorial membrane. Laryngoscope. 1987;97(3 Pt 1):357–367.
- Slepecky NB, Savage JE, Cefaratti LK, et al. Electron-microscopic localization of type II, IX, and V collagen in the organ of Corti of the gerbil. Cell Tissue Res. 1992;267(3):413–418.
- Goodyear RJ, Richardson GP. Structure, function, and development of the tectorial membrane: an extracellular matrix essential for hearing. Curr Top Dev Biol. 2018;130:217–244.
- Iurato S. Submicroscopic structure of the membranous labyrinth. 1. The tectorial membrane. Z Zellforsch Mikrosk Anat. 1960;52(1):105–128.
- Thorn L, Arnold W, Schinko I, et al. Light and electron microscopic studies of the greater epithelial ridge and its relationship to the developing tectorial membrane in the cochlear duct of the guinea pig (author's transl). Arch Otorhinolaryngol. 1978;221(2):123–133.
- Prieto JJ, Rueda J, Merchan JA. Two different secretion mechanisms in the inner ear’s interdental cells. Hear Res. 1990;45(1–2):51–61.
- Pfister M, Thiele H, Van Camp G, et al. A genotype-phenotype correlation with gender-effect for hearing impairment caused by TECTA mutations. Cell Physiol Biochem. 2004;14(4–6):369–376.
- Verhoeven K, Laer L, Van Kirschhofer K, et al. Mutations in the human alpha-tectorin gene cause autosomal dominant non-syndromic hearing impairment. Nat Genet. 1998;19(1):60–62.
- Alasti F, Sanati MH, Behrouzifard AH, et al. A novel TECTA mutation confirms the recognizable phenotype among autosomal recessive hearing impairment families. Int J Pediatr Otorhinolaryngol. 2008;72(2):249–255.
- Lukashkin AN, Legan PK, Weddell TD, et al. A mouse model for human deafness DFNB22 reveals that hearing impairment is due to a loss of inner hair cell stimulation. Proc Natl Acad Sci USA. 2012;109(47):19351–19356.
- Legan PK, Lukashkina VA, Goodyear RJ, et al. A targeted deletion in alpha-tectorin reveals that the tectorial membrane is required for the gain and timing of cochlear feedback. Neuron. 2000;28(1):273–285.
- Sellon JB, Ghaffari R, Farrahi S, et al. Porosity controls spread of excitation in tectorial membrane traveling waves. Biophys J. 2014;106(6):1406–1413.
- Meaud J, Grosh K. The effect of tectorial membrane and basilar membrane longitudinal coupling in cochlear mechanics. J Acoust Soc Am. 2010;127(3):1411–1421.
- Teudt IU, Richter CP. Basilar membrane and tectorial membrane stiffness in the CBA/CaJ mouse. J Assoc Res Otolaryngol. 2014;15(5):675–694.
- Gueta R, Barlam D, Shneck RZ, et al. Measurement of the mechanical properties of isolated tectorial membrane using atomic force microscopy. Proc Natl Acad Sci USA. 2006;103(40):14790–14795.
- Gavara N, Chadwick RS, Gribble PL. Collagen-based mechanical anisotropy of the tectorial membrane: implications for inter-row coupling of outer hair cell bundles. PLoS One. 2009;4(3):e4877.
- Kimura RS. Hairs of the cochlear sensory cells and their attachment to the tectorial membrane. Acta Otolaryngol. 1966;61(1):55–72.
- Nowotny M, Gummer AW. Nanomechanics of the subtectorial space caused by electromechanics of cochlear outer hair cells. Proc Natl Acad Sci USA. 2006;103(7):2120–2125.
- Zwaenepoel I, Mustapha M, Leibovici M, et al. Otoancorin, an inner ear protein restricted to the interface between the apical surface of sensory epithelia and their overlying acellular gels, is defective in autosomal recessive deafness DFNB22. Proc Natl Acad Sci USA. 2002;99(9):6240–6245.
- Schuknecht HF, Gacek MR. Cochlear pathology in presbycusis. Ann Otol Rhinol Laryngol. 1993;102(1 Pt 2):1–16.
- Békésy GV, Sy GVB. DC resting potentials inside the cochlear partition exploration of cochlear potentials in guinea pig with a microelectrode DC resting potentials inside the cochlear partition. Cit J Acoust Soc Am. 1952;24(1):72.
- Schulte BA, Schmiedt RA. Lateral wall Na, K-ATPase and endocochlear potentials decline with age in quiet-reared gerbils. Hear Res. 1992;61(1–2):35–46.
- Iizuka T, Kamiya K, Gotoh S, et al. Perinatal Gjb2 gene transfer rescues hearing in a mouse model of hereditary deafness. Hum Mol Genet. 2015;24(13):3651–3661.
- Salt AN, Melichar I, Thalmann R. Mechanisms of endocochlear potential generation by stria vascularis. Laryngoscope. 1987;97(8 Pt 1):984–991.
- Takeuchi S, Ando M, Kakigi A. Mechanism generating endocochlear potential: role played by intermediate cells in stria vascularis. Biophys J. 2000;79(5):2572–2582.
- Nin F, Hibino H, Doi K, et al. Y. The endocochlear potential depends on two K + diffusion potentials and an electrical barrier in the stria vascularis of the inner ear. Proc Natl Acad Sci USA. 2008;105(5):1751–1756.
- Wangemann P, Liu J, Marcus DC. Ion transport mechanisms responsible for K + secretion and the transepithelial voltage across marginal cells of stria vascularis in vitro. Hear Res. 1995;84(1–2):19–29.
- Shen Z, Marcus DC, Sunose H, et al. I(sK) channel in strial marginal cells. Voltage-dependence, ion-selectivity, inhibition by 293B and sensitivity to clofilium. Aud Neurosci. 1997;3(3):215–230.
- Gow A, Davies C, Southwood CM, et al. Deafness in Claudin 11-null mice reveals the critical contribution of basal cell tight junctions to stria vascularis function. J Neurosci. 2004;24(32):7051–7062.
- Kitajiri SI, Miyamoto T, Mineharu A, et al. Compartmentalization established by claudin-11-based tight junctions in stria vascularis is required for hearing through generation of endocochlear potential. J Cell Sci. 2004;117(Pt 21):5087–5096.
- Hibino H, Horio Y, Inanobe A, et al. An ATP-dependent inwardly rectifying potassium channel, KAB-2 (Kir4. 1), in cochlear stria vascularis of inner ear: its specific subcellular localization and correlation with the formation of endocochlear potential. J Neurosci. 1997;17(12):4711–4721.
- Hibino H, Nin F, Tsuzuki C, et al. How is the highly positive endocochlear potential formed? the specific architecture of the stria vascularis and the roles of the ion-transport apparatus. Pflugers Arch. 2010;459(4):521–533.
- Nin F, Yoshida T, Sawamura S, et al. The unique electrical properties in an extracellular fluid of the mammalian cochlea; their functional roles, homeostatic processes, and pathological significance. Pflugers Arch Eur J Physiol. 2016;4(10):1637–1649.
- Marcus DC, Wu T, Wangemann P, et al. KCNJ10 (kir4.1) potassium channel knockout abolishes endocochlear potential. Am J Physiol Cell Physiol. 2002;282(2):C403–C407.
- Liu W, Schrott-Fischer A, Glueckert R, et al. The human “Cochlear Battery” - claudin-11 barrier and ion transport proteins in the lateral wall of the cochlea. Front Mol Neurosci. 2017;10:239.
- Kikuchi T, Adams JC, Miyabe Y, et al. Potassium ion recycling pathway via gap junction systems in the mammalian cochlea and its interruption in hereditary nonsyndromic deafness. Med Electron Microsc. 2000;33(2):51–56.
- Forge A, Becker D, Casalotti S, et al. Connexins and gap junctions in the inner ear. Audiol Neurootol. 2002;7(3):141–145.
- Zhang Y, Tang W, Ahmad S, et al. Gap junction-mediated intercellular biochemical coupling in cochlear supporting cells is required for normal cochlear functions. Proc Natl Acad Sci USA. 2005;102(42):15201–15206.
- Linthicum FH, Fayad JN. Spiral ganglion cell loss is unrelated to segmental cochlear sensory system degeneration in humans. Otol Neurotol. 2009;30(3):418–422.
- Liu W, Glueckert R, Linthicum FH, et al. Possible role of gap junction intercellular channels and connexin 43 in satellite glial cells (SGCs) for preservation of human spiral ganglion neurons: a comparative study with clinical implications. Cell Tissue Res. 2014;355(2):267–278.
- Rask-Andersen H, Tylstedt S, Kinnefors A, et al. Synapses on human spiral ganglion cells: A transmission electron microscopy and immunohistochemical study. Hear Res. 2000;141(1–2):1–2.
- Tylstedt S, Rask-Andersen H. A 3-D model of membrane specializations between human auditory spiral ganglion cells. J Neurocytol. 2001;30(6):465–473.
- Rask-Andersen H, Tylstedt S, Kinnefors A, et al. Nerve fibre interaction with large ganglion cells in the human spiral ganglion: a TEM study. Auris Nasus Larynx. 1997;24(1):1–11.
- Zong L, Zhu Y, Liang R, et al. Gap junction mediated miRNA intercellular transfer and gene regulation: a novel mechanism for intercellular genetic communication. Sci Rep. 2016;6:19884.
- Tokhtaeva E, Clifford RJ, Kaplan JH, et al. Subunit isoform selectivity in assembly of Na,K. ATPase α-β Heterodimers. J Biol Chem. 2012;287(31):26115–26125.
- Geering K. The functional role of the β-subunit in the maturation and intracellular transport of Na,K-ATPase. FEBS Lett. 1991;285:189–193.