ABSTRACT
Ideal methods for detecting pathogens should be sensitive, specific, rapid, cost-effective and instrument-free. Conventional nucleic acid pathogen detection strategies, mostly PCR-based techniques, have various limitations, such as expensive equipment, reagents and skilled performance. Recently, CRISPR/Cas-based methods have burst onto the scene, with the potential to power the pathogen detection field. Here we introduce these unique methods and discuss its hurdles and promises.
Introduction
Infectious diseases are the cause of about 22% of all human deaths, often inflicting public distress and huge economic loss [Citation1]. Rapid molecular detection has become indispensable for diagnosing and monitoring infectious pathogen, for providing update disease information to control spread and timely treatment responses [Citation2]. Point-of-care testing (POCT) will help to promptly optimize decision-making, improve the efficiency of care and decrease costs, especially in the resource-constrained region [Citation3]. According to the World Health Organization ASSURED criteria, an ideal pathogen diagnostic test would be inexpensive, sensitive, specific, easy-to-use, rapid, without large equipment and delivered to the user [Citation4]. A vast array of assays have emerged for detecting nucleic acid signatures of pathogens [Citation5], including PCR/qPCR-based detection methods, isothermal amplification-based detection assays and next-generation sequencing-based pathogen diagnostics [Citation6–8]. However, these techniques are time- and money-consuming, low sensitivity and low specificity, and require specialized and expensive equipment, high-level technical expertise (), thus cannot fully satisfy with the rapid point-of-care tests and prohibit widespread use.
Table 1. Comparisons of CRISPR/Cas-based detection with current pathogen nucleic acid diagnostic methods.
CRISPR-Cas systems consist of an RNA guide(s) for target recognition and a Cas enzyme, which has evolved in bacteria and archaea to defend against foreign viruses by cleaving their nucleic acid [Citation9–12]. CRISPR-Cas system has been harnessed not only for genome and RNA editing [Citation13,Citation14], but lately, also for nucleic acid detection [Citation15–17], which has engendered much excitement. The CRISPR-Cas systems are grouped into two classes (Class 1 and 2), and the most widely used toolbox for nucleic acid detection contains Cas9, Cas12, Cas13 and Cas14 belongs to class 2 system, as detailed below [Citation18,Citation19].
In this review, we first give a brief description of the current knowledge of CRISPR/Cas-based nucleic acid detection system. We also describe the possible technical challenges that should be considered for developing CRISPR/Cas-based diagnostics for outbreak pathogen.
Detection based on specific cleavage or binding of DNA: Cas9
CRISPR/Cas9, a Class 2 protein, is guided by RNA to cleave double-stranded DNA (dsDNA). To detect and genotype Zika virus (ZIKV), Pardee and collaborators first amplified the viral RNA using an isothermal amplification – nucleic acid sequence based amplification (NASBA). CRISPR/Cas9 was then used to cleave the DNA, and the event detected by toehold switch sensors, leading to a colourimetric output on test paper (A). This system is capable of distinguishing American and African strains of ZIKV with single-base resolution [Citation20].
Figure 1. Detection of pathogen nucleic acids with CRISPR-Cas9 based assay. (A) Schematic of NASBACC detection. A synthetic trigger sequence and T7 primer are appended to a NASBA-amplified RNA fragment through reverse transcription. The Cas9 specific cleavage of the sequence with PAM, leading to the production of either truncated or full-length trigger RNA, which differentially activates a toehold switch sensor. (B) Schematic of CAS-EXPAR detection. The Cas9/sgRNA complexed with designed PAMer induces site-specific cleavage of ssDNA substrates producing cleaved fragments. The fragment will be hybridized with the EXPAR template and amplified by DNA polymerase, which can be monitored with a real-time fluoresces. (C) Schematic of ctPCR detection. The CRISPR-typing PCR (ctPCR) integrates Cas9 cutting with PCR assay. By using Cas9 specific cleavage to get a second-round primer then start the amplification with PCR or qPCR, and visualized with gel or fluorescence readout. (D) Schematic of CASLFA detection. The CASLFA system means CRISPR/Cas9-mediated lateral flow nucleic acid assay. The CRISPR/Cas9 is integrated with a lateral flow detection platform, which will show a band on the test line of the strip when there appears the target sequence. (E) Schematic of Paired dCas9 (PC) reporter system detection. The firefly luciferase (NFluc or CFluc) is split and fused to two dCas9s. When the two dCas9s closely binding to the target sequence, will bring the half luciferase into proximity to form integral enzyme which catalyzes the bioluminescent reaction. (F) Schematic of CRISPR-mediated DNA-FISH detection. In the system, a magnetic nano-bead is fused with dCas9, and the target nucleic acid once bind by the dCas9-sgRNA complex will be isolated by magnetic and give the fluorescence signal by SYBR Green staining. (G) Schematic of CRISPR-Chip detection. CRISPR–Chip is composed of a gFET construct, on which the dCas9 complexed with a target-specific sgRNA immobilized on the surface of the graphene. When there is target DNA on the chip, the dCas9 kinetically binds to the target DNA, which will modulate the electrical characteristics of the gFET and result in electrical signal output. ssRNA, single-strand RNA; NASBA, nucleic acid sequence-based amplification; dsDNA, double-stranded DNA; PAM, protospacer-adjacent motif; ssDNA, single-stranded DNA; dCas9, Nuclease-deactivated Cas9; PC, paired dCas9; RCA, rolling circle amplification.
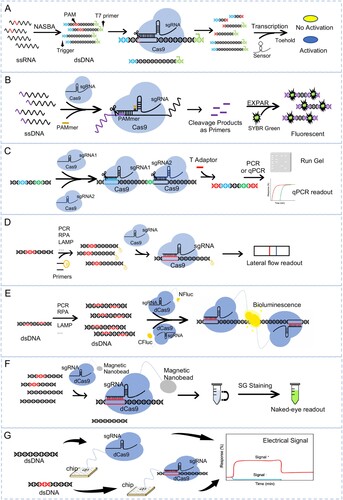
Recently, Huang and colleagues developed a CRISPR/Cas9 triggered isothermal exponential amplification reaction (CAS-EXPAR) strategy, which detects DNA targets with attomolar (aM) sensitivity and single-base specificity [Citation21]. CAS-EXPAR is primed by the target DNA fragment produced by cleavage of CRISPR/Cas9, and the amplification reaction performs cyclically to generate a large number of DNA replicates which are detected using a real-time SYBR Green fluorescence signal (B). The system successfully provides the proof-of-concept of detecting DNA methylation and Listeria monocytogenes RNA [Citation21]. Another group developed two methods, named CRISPR-typing PCR (ctPCR) and CRISPR-associated reverse PCR (CARP) for detecting DNA [Citation22–24]. These assays combine CRISPR specific cleavage and PCR amplification of target DNA, and then the results can be visualized with gel readout or qPCR (C). ctPCR and CARP are verified by detecting HPV16 and HPV18 L1 gene of human papillomavirus (HPV) [Citation22–24]. More recently, Wang et al. combine CRISPR/Cas9 with the lateral flow assay to develop CRISPR/Cas9-mediated lateral flow nucleic acid assay (CASLFA) (D) [Citation25]. The CASLFA is utilized to identify Listeria monocytogenes and African swine fever virus (ASFV) at a detection limit of hundreds of copies of genome samples with high specificity within 1 h [Citation25].
The nuclease-dead Cas9 (dCas9), which binds DNA without cleaving it, has also been repurposed. Zhang et al. split luciferase and fused the two halves each to dCas9; the two halves can heterodimerize to reconstitute intact protein and produce bioluminescent signal if and only if they are targeted to adjacent sites on DNA. To detect Mycobacterium tuberculosis DNA [Citation26], the pathogen DNA was pre-amplified, and the dCas9 fusion proteins added together with the two gRNAs targeting the pathogen DNA, which will produce bioluminescence if the amplified DNA is the expected pathogen DNA (E) [Citation26]. A similar strategy is used for rapidly detecting MicroRNAs, termed as rolling circle amplification (RCA)-CRISPR-split-HRP (RCH) system [Citation27]. More recently, Kyeonghye Guk and colleagues introduce a CRISPR-mediated DNA-FISH method for the simple, rapid and highly sensitive detection using dCas9 for specific targeting and SYBR Green I (SG I) as a fluorescent probe [Citation22](F). This CRISPR-mediated DNA-FISH can detect methicillin-resistant Staphylococcus aureus (MRSA) as low as 10 CFU/ml within 30 min [Citation28].
The methods above all need amplification to improve sensitivity. In contrast, Reza Hajian and colleagues develop a CRISPR–Chip, a label-free nucleic-acid-testing device, to detect a target sequence within intact genomic material [Citation29]. The biosensor uses dCas9-gRNA complex immobilized on the graphene-based field-effect transistor, whose output signal can be measured with a simple handheld reader (G). The CRISPR–Chip detects target DNA with a sensitivity of 1.7 fM in 15 min, and can diagnose DNA mutations in Duchenne muscular dystrophy clinical samples [Citation29]. CRISPR–Chip expands the applications of CRISPR–Cas9 technology to the on-chip electrical detection of nucleic acids.
Detection based on collateral cleavage: other Cas proteins
In multiple Cas family members, including Cas13, Cas12 and Cas14 effector, cutting the target nucleic acid can trigger the cleavage of irrelevant single-strand DNA (ssDNA) or single-strand RNA (ssRNA). This collateral cleavage [Citation30–34] has been exploited for nucleic acid detection [Citation31–33,Citation35–37].
Cas13-based system
CRISPR-Cas13a (formerly C2c2), a Type VI Class 2 CRISPR-Cas effector, is a single-component enzyme targeting single-stranded RNA with a guide RNA [Citation38]. Binding with a complementary ssRNA will activate its targeting and general ssRNase activity (A) [Citation34,Citation38], the latter responsible for collateral ssRNA cleavage. In the specific high-sensitivity enzymatic reporter unlocking (SHERLOCK) platform, quenched fluorophore is added to the collateral substrate, which becomes released and thus emits fluorescence once the substrate is cut, thus enabling target RNA detection () [Citation35]. For nucleic acid detection, a recombinase polymerase amplification (RPA) or reverse transcriptase RPA is used to amplify target DNA, which is then transcribed into RNA for detection. The fluorescence signal is monitored using the spectroscopy reader (). To expedite the detection, a pathogen sample treatment method – HUDSON (heating unextracted diagnostic samples to obliterate nucleases) was introduced, which enables detection directly from bodily fluids without nucleic acid extraction. SHERLOCK enables instrument-free Zika virus (ZIKV) and dengue virus (DENV) detection directly from patient samples (such as serum, urine and saliva) in 2 h, at concentrations as low as 1 copy per microliter [Citation39].
Figure 2. Diagnostic of pathogen nucleic acids with trans-cleavage of active CRISPR-Cas. Schematic of a CRISPR effector nuclease exploited for nucleic acid detection in SHERLOCK (Cas13a), DETECTR (Cas12a) or Cas4-DETECTR (Cas14a). In the absence of its nucleic acid target, the Cas nuclease is inactive. Upon binding of its guide crRNA to a cognate target (RNA for Cas13a, dsDNA for Cas12a, ssDNA for Cas14a), the nuclease is activated, leading to target cleavage and catalytic cleavage of nearby nucleic acids (ssRNA for Cas13a, ssDNA for Cas12a and Cas14a). This “collateral” nuclease activity is turned into an amplified signal by providing reporter probes with a fluorophore (usually FAM) linked to a quencher (or Biotin for later flow assay) by a short oligonucleotide. Upon cleavage of the reporter by the activated nuclease, the reporter nucleotide will break and thus fluoresces bright or band appearing in a strip. In both SHERLOCK and DETECTR, target abundance is enhanced by isothermal pre-amplification using RPA with or without in vitro transcription or reverse transcription.
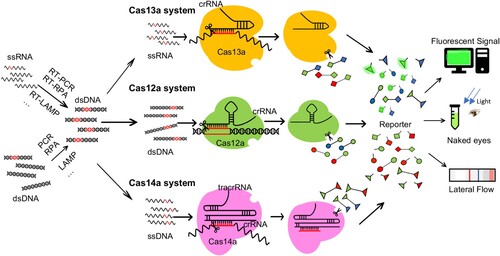
In the enhanced SHERLOCKv2, four nucleic acid sequences are detectable in a single reaction, using a combination of 4 different Cas13 and Cas12 enzymes, and the detection sensitivity is enhanced about 3.5-fold by using Csm6, a CRISPR-associated enzyme [Citation40], to boost Cas13 activity [Citation41]. Finally, a reporter probe for a lateral flow assay is introduced, which allows visual readout on the test strip. SHERLOCKv2 can detect Dengue or Zika virus single-stranded RNA as well as mutations in patient liquid biopsy samples via lateral flow [Citation41].
Cas12-based detection system
Cas12a (also known as Cpf1), a Class V CRISPR-Cas protein, consists of one RuvC endonuclease domain and recognize T rich PAM sequence for target cleavage. Its dsDNA collateral cleavage reflects its general ssDNase activity on a bound dsDNA substrate [Citation30,Citation31] (). In DETECTR (DNA endonuclease targeted CRISPR trans-reporter) and HOLMES (a one-HOur Low-cost Multipurpose highly Efficient System) [Citation31,Citation37]. Target nucleic acid is amplified with isothermal amplification by RPA or RT-RPA, which then bind the Cas12a-sgRNA complex and trigger the cleavage of an ssDNA fluorophore-quencher reporter, generating a fluorescent signal (). DETECTR and HOLMES can detect DNA sequences with attomolar sensitivity and high specificity. DETECTR has been employed to detect and genotype HPV strains in patient samples [Citation31], and HOLMES to detect DNA and RNA viruses, distinguishing between the strains with high specificity [Citation37].
Cas12b also has similar activity as Cas12a and used in HOLMESv2 and CDetection (Cas12b-mediated DNA detection) [Citation32,Citation36]. CDetection can directly detect the HPV16 DNAs in human plasma at the concentration of 1 attomolar [Citation32]. A series of improvements on the Cas12-based nucleic acid detection system have been reported. To date, multiple amplification methods (including PCR, RPA, LAMP) and single readout forms (e.g. fluorescence detector, naked-eye view and later flow assay) are integrated, making the methods sensitive, accurate, portable and easy-to-use () [Citation5,Citation16].
Cas14-based detection system
Cas14, a small Cas protein half the size of other Cas proteins, comprises 24 variants clustered into three subgroups (Cas14a-c), which share a conserved RuvC nuclease domain [Citation33]. Cas14 proteins are capable of targeted single-stranded DNA (ssDNA) cleavage without requiring a PAM for activation. Moreover, target recognition by Cas14 triggers non-specific ssDNA molecule cleavage activity, similar to the RuvC-containing enzyme Cas12a [Citation31,Citation33].
Cas14a is integrated into the DETECTR platform to generate a new ssDNA detection system termed Cas14a-DETECTR (). It enables high-fidelity DNA single nucleotide polymorphisms (SNPs) detection without PAM constraint [Citation33]. Inspired by this study, several researchers propose to detect DNA virus infections with Cas14a-DETECTR [Citation42,Citation43], but no pathogen diagnosis case has been reported.
The strengths of CRISPR/Cas based pathogen detection systems
An accurate, rapid and affordable nucleic acid detection method plays an important role in infectious pathogen diagnostic. The PCR-based diagnostic, the mostly used method, is high sensitivity and specificity, but needs particular instrument and professional skill, which limits its widespread application. Meanwhile, the sequencing is becoming more and more critical in nucleic acid detection; however, the high complexity and cost hamper its application in rapid on-site infectious diagnostic. Isothermal amplification of nucleic acids has emerged as a promising alternative in rapid and portable detection compared over PCR, but its sensitivity and specificity need to be improved [Citation7].
Highly specific and sensitive, fast, cost-effective and easy-to-use, the CRISPR/Cas-based methods hold great promises for detecting pathogens such as SARS-CoV-2, Zika, Ebola, HPV and M. tuberculosis (). The methods are also undergoing further improvements and modifications to make them more versatile. Importantly, the simplicity of the CRISPR/Cas-based detection system allows the rapid development of diagnostic methods for an emergency outbreak of infectious diseases. For example, integrating later flow assay (CORDS, CRISPR/Cas12a-LFD) and naked-eye detection (CRISPR/Cas-based colorimetric platform, fluorescence-based POC system), four groups quickly developed Cas12a-based on-site diagnostic assays for African swine virus (ASFV), an emergence virus for the global swine industry () [Citation44–47]. For the current COVID-19 pandemic [Citation48], several research groups are developing CRISPR/Cas-based diagnostic assay [Citation49–53]. Among which, these diagnostic methods can detect as low as 2 copies of extracted SARS-CoV-2 RNA and readout on later flow strip in an hour without requiring for elaborate instrumentation or visualized by green fluorescent with naked-eye under 485 nm light [Citation49–52]. More recently, Joung and colleagues developed an integrated test called STOPCovid (SHERLOCK Testing in One Pot) for detecting SARS-CoV-2, which can return result on the strip in 70 min, with a detection limit of 100 copies of viral genome input in saliva or nasopharyngeal swabs per reaction [Citation53]. Compared with the RT-qPCR-based diagnostic methods, these CRISPR/Cas-based SARS-CoV-2 tests are featured with high speed, no requirement for expensive instrument and professional technician, which will significantly aid the COVID-19 epidemic control.
Table 2. Diagnostic of infection pathogen with CRISPR/Cas-based detection.
Future perspectives
As mentioned before, an ideal pathogen diagnostic test would be inexpensive, sensitive, specific, easy-to-use, rapid, without large equipment and delivered to the user [Citation4]. We will discuss the pros and cons of CRISPR/Cas-based pathogen diagnostic; moreover, we expect a mobile-powered CRISPR/Cas-based early alarm system.
The sensitivity and specificity of CRISPR/Cas-based pathogen detection methods are outstanding: they have reached the aM (SHERLOCK, DETECTR) and even the zM (HOLMES and SHERLOCKv2) ranges [Citation16], and can distinguish SNP, HPV strains (e.g. DETECTR, crPCR and CARP), Zika virus strains (NASBACC), and can detect point mutations (e.g. SHERLOCK; HOLMES) [Citation54]. Further improvement may be made by identifying new Cas enzyme and engineering existing ones, and by optimizing the signal amplification system (such as combining new engineering materials and synthetic elements).
Regarding the speed, the fastest assays (e.g. SHERLOCKv2, CRISPR-Chip) takes at least 15 min [Citation29,Citation41]. To improve the speed, one may seek to discover novel robust Cas protein and optimize the reaction by e.g. adding new components.
Regarding the ease-of-use, several simplified formats have been developed, including paper-based biosensors with visual readout (NASBACC; SHERLOCK; DETECTR), pathogen detection without nucleic acid extraction (HUDSON) [Citation39], and a single-tube assay combing isothermal amplification and Cas-mediate reaction [Citation41,Citation55]. In future, one step diagnostic should be developed, including pathogen nucleic acid release, pre-amplification, CRISPR-Cas induced reaction and signal readout, which will be user-friendly.
In terms of cost-effectiveness, the cost estimates of the NASBACC and SHERLOCK technologies are under $1 per test [Citation20,Citation56], DETECTR and HOLMES have also been proposed as low-cost technologies [Citation54]. By systematic optimization, the price is expected to be even lower.
On-site pathogen diagnostic is ideally portable without needing complex equipment. Isothermal amplification that does not require instruments has been widely integrated into CRISPR-based detection [Citation7]. For signal readout, more equipment-free methods, such as later flow assay or naked-eye view under light, should be introduced [Citation41,Citation45,Citation46,Citation55]. Currently, the store and delivery of the CRISPR-based detection system still need a cold chain. Keith Pardee and colleagues tested the NASBACC detection system with freeze-dried reagents, allowing for long-term storage and transport [Citation20]. The SHERLOCK system also works well with freeze-drying and paper spotting reagents [Citation35]. More storage and transportation solutions of the CRISPR-based reaction kit at room temperature are to be developed.
Finally, artificial intelligence (AI) can be combined with CRISPR-Cas diagnostic tests to build an early alarm system for rapid, accurate and more intelligent diagnosis of the infectious pathogen (). Specifically, a user-friendly portable CRISPR/Cas-based point-of-care detection kits are delivered to community, hospital, health centre or even individual to detect the specific infectious pathogens rapidly and accurately. The detection readout is sensed with a mobile phone through an APP, and the result data transformed into the cloud for storage and processing through the 5G service. In the era of big data, powered with an AI model, more personalized and refined data interpretation and early warning can be realized. Therefore, at the social group level, accurate updated pathogen diagnostic data and AI-powered machine learning through big data will more accurately alarm the risk and give suggestions for countermeasure. At the individual level, the system will provide early warning of the risk of emerging infectious viruses nearby. For example, such a CRISPR-Cas diagnostic AI-powered alarm system should be beneficial for the timely response and prevention of the spread of COVID-19.
Figure 3. Pathogen diagnostic and alarm with CRISPR/Cas-based cloud detection. Schematic diagram of an early alarm of infection risk system based on CRISPR-Cas rapid diagnostic and artificial intelligence (AI) powered predication. Individual patient from the community, hospital or health centre, can be rapidly and accurately detected with the specific infectious pathogen. The readout data will be transformed into the cloud for store and process through the 5G service of a mobile phone app. The cloud computing will calculate the infectious risk to generate an AI-powered model on update diagnostic cases, then warn the administrators or individuals.
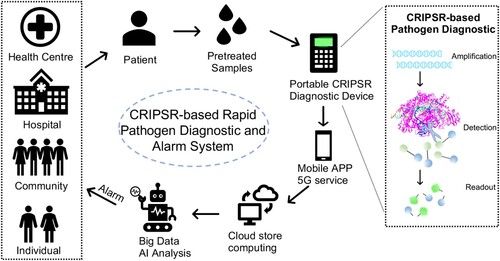
In summary, although there is still much room for improvement, CRISPR/Cas-based systems has emerged as the tool of the future for pathogen diagnosis.
Acknowledgements
We thank members of the Huang lab for helpful discussions. We are grateful to Dr. Tian Chi from ShanghaiTech University for critical reading and excellent language editing.
Disclosure statement
No potential conflict of interest was reported by the authors.
Additional information
Funding
References
- Lozano R, Naghavi M, Foreman K, et al. Global and regional mortality from 235 causes of death for 20 age groups in 1990 and 2010: asystematic analysis for the global burden of disease study 2010. Lancet. 2012 Dec 15;380(9859):2095–2128.
- Broadhurst MJ, Brooks TJ, Pollock NR. Diagnosis of ebola virus disease: past, present, and future. Clin Microbiol Rev. 2016 Oct;29(4):773–793.
- Nayak S, Blumenfeld NR, Laksanasopin T, et al. Point-of-care diagnostics: recent developments in a connected age. Anal Chem. 2017 Jan 3;89(1):102–123.
- Kosack CS, Page AL, Klatser PR. A guide to aid the selection of diagnostic tests. Bull World Health Organ. 2017 Sep 1;95(9):639–645.
- Bhattacharyya RP, Thakku SG, Hung DT. Harnessing crispr effectors for infectious disease diagnostics. ACS Infect Dis. 2018 Sep 14;4(9):1278–1282.
- Scheler O, Glynn B, Kurg A. Nucleic acid detection technologies and marker molecules in bacterial diagnostics. Expert Rev Mol Diagn. 2014 May;14(4):489–500.
- Zhao Y, Chen F, Li Q, et al. Isothermal amplification of nucleic acids. Chem Rev. 2015 Nov 25;115(22):12491–12545.
- Matthijs G, Souche E, Alders M, et al. Guidelines for diagnostic next-generation sequencing. Eur J Hum Genet. 2016 Jan;24(1):2–5.
- Horvath P, Barrangou R. Crispr/cas, the immune system of bacteria and archaea. Science. 2010 Jan 8;327(5962):167–170.
- Garneau JE, Dupuis ME, Villion M, et al. The crispr/cas bacterial immune system cleaves bacteriophage and plasmid DNA. Nature. 2010 Nov 4;468(7320):67–71.
- Brouns SJ, Jore MM, Lundgren M, et al. Small crispr rnas guide antiviral defense in prokaryotes. Science. 2008 Aug 15;321(5891):960–964.
- Barrangou R, Fremaux C, Deveau H, et al. Crispr provides acquired resistance against viruses in prokaryotes. Science. 2007 Mar 23;315(5819):1709–1712.
- Xu X, Qi LS. A crispr-dcas toolbox for genetic engineering and synthetic biology. J Mol Biol. 2019 Jan 4;431(1):34–47.
- Komor AC, Badran AH, Liu DR. Crispr-based technologies for the manipulation of eukaryotic genomes. Cell. 2017 Jan 12;168(1–2):20–36.
- Khambhati K, Bhattacharjee G, Singh V. Current progress in crispr-based diagnostic platforms. J Cell Biochem. 2019 Mar;120(3):2721–2725.
- Li Y, Li S, Wang J, et al. Crispr/cas systems towards next-generation biosensing. Trends Biotechnol. 2019 Jul;37(7):730–743.
- Chertow DS. Next-generation diagnostics with crispr. Science. 2018 Apr 27;360(6387):381–382.
- Makarova KS, Wolf YI, Alkhnbashi OS, et al. An updated evolutionary classification of crispr-cas systems. Nat Rev Microbiol. 2015 Nov;13(11):722–736.
- Mota DS, Marques JM, Guimarães JM, et al. Research article crispr/cas class 2 systems and their applications in biotechnological processes. Genet Mol Res. 2020;19(1):gmr18478, 1–11.
- Pardee K, Green AA, Takahashi MK, et al. Rapid, low-cost detection of zika virus using programmable biomolecular components. Cell. 2016 May 19;165(5):1255–1266.
- Huang M, Zhou X, Wang H, et al. Clustered regularly interspaced short palindromic repeats/cas9 triggered isothermal amplification for site-specific nucleic acid detection. Anal Chem. 2018 Feb 6;90(3):2193–2200.
- Zhang B, Wang Q, Xu X, et al. Detection of target DNA with a novel cas9/sgrnas-associated reverse pcr (carp) technique. Anal Bioanal Chem. 2018 May;410(12):2889–2900.
- Wang Q, Zhang B, Xu X, et al. Crispr-typing pcr (ctpcr), a new cas9-based DNA detection method. Sci Rep. 2018 Sep 20;8(1):14126.
- Zhang B, Xia Q, Wang Q, et al. Detecting and typing target DNA with a novel crispr-typing pcr (ctpcr) technique. Anal Biochem. 2018 Nov 15;561–562:37–46.
- Wang X, Xiong E, Tian T, et al. Clustered regularly interspaced short palindromic repeats/cas9-mediated lateral flow nucleic acid assay. ACS Nano. 2020 Feb 25;14(2):2497–2508.
- Zhang Y, Qian L, Wei W, et al. Paired design of dcas9 as a systematic platform for the detection of featured nucleic acid sequences in pathogenic strains. ACS Synth Biol. 2017 Feb 17;6(2):211–216.
- Qiu XY, Zhu LY, Zhu CS, et al. Highly effective and low-cost microrna detection with crispr-cas9. ACS Synth Biol. 2018 Mar 16;7(3):807–813.
- Guk K, Keem JO, Hwang SG, et al. A facile, rapid and sensitive detection of mrsa using a crispr-mediated DNA fish method, antibody-like dcas9/sgrna complex. Biosens Bioelectron. 2017 Sep 15;95:67–71.
- Hajian R, Balderston S, Tran T, et al. Detection of unamplified target genes via crispr-cas9 immobilized on a graphene field-effect transistor. Nat Biomed Eng. 2019 Jun;3(6):427–437.
- Li SY, Cheng QX, Liu JK, et al. Crispr-cas12a has both cis- and trans-cleavage activities on single-stranded DNA. Cell Res. 2018 Apr;28(4):491–493.
- Chen JS, Ma E, Harrington LB, et al. Crispr-cas12a target binding unleashes indiscriminate single-stranded dnase activity. Science. 2018 Apr 27;360(6387):436–439.
- Teng F, Guo L, Cui T, et al. Cdetection: crispr-cas12b-based DNA detection with sub-attomolar sensitivity and single-base specificity. Genome Biol. 2019 Jul 1;20(1):132.
- Harrington LB, Burstein D, Chen JS, et al. Programmed DNA destruction by miniature crispr-cas14 enzymes. Science. 2018 Nov 16;362(6416):839–842.
- East-Seletsky A, O’Connell MR, Knight SC, et al. Two distinct rnase activities of crispr-c2c2 enable guide-rna processing and rna detection. Nature. 2016 Oct 13;538(7624):270–273.
- Gootenberg JS, Abudayyeh OO, Lee JW, et al. Nucleic acid detection with crispr-cas13a/c2c2. Science. 2017 Apr 28;356(6336):438–442.
- Li L, Li S, Wu N, et al. Holmesv2: acrispr-cas12b-assisted platform for nucleic acid detection and DNA methylation quantitation. ACS Synth Biol. 2019 Oct 18;8(10):2228–2237.
- Li SY, Cheng QX, Wang JM, et al. Crispr-cas12a-assisted nucleic acid detection. Cell Discov. 2018;4:20.
- Abudayyeh OO, Gootenberg JS, Konermann S, et al. C2c2 is a single-component programmable rna-guided rna-targeting crispr effector. Science. 2016 Aug 5;353(6299):aaf5573.
- Myhrvold C, Freije CA, Gootenberg JS, et al. Field-deployable viral diagnostics using crispr-cas13. Science. 2018 Apr 27;360(6387):444–448.
- Kazlauskiene M, Kostiuk G, Venclovas Č, et al. A cyclic oligonucleotide signaling pathway in type iii crispr-cas systems. Science. 2017;357(6351):605–609.
- Gootenberg JS, Abudayyeh OO, Kellner MJ, et al. Multiplexed and portable nucleic acid detection platform with cas13, cas12a, and csm6. Science. 2018 Apr 27;360(6387):439–444.
- Khan MZ, Haider S, Mansoor S, et al. Targeting plant ssdna viruses with engineered miniature crispr-cas14a. Trends Biotechnol. 2019 Aug;37(8):800–804.
- Aquino-Jarquin G. Crispr-cas14 is now part of the artillery for gene editing and molecular diagnostic. Nanomedicine. 2019 Jun;18:428–431.
- He Q, Yu D, Bao M, et al. High-throughput and all-solution phase african swine fever virus (asfv) detection using crispr-cas12a and fluorescence based point-of-care system. Biosens Bioelectron. 2020 Apr 15;154:112068.
- Yuan C, Tian T, Sun J, et al. Universal and naked-eye gene detection platform based on the clustered regularly interspaced short palindromic repeats/cas12a/13a system. Anal Chem. 2020 Mar 3;92(5):4029–4037.
- Wang X, Ji P, Fan H, et al. Crispr/cas12a technology combined with immunochromatographic strips for portable detection of african swine fever virus. Commun Biol. 2020 Feb 11;3(1):62.
- Bai J, Lin H, Li H, et al. Cas12a-based on-site and rapid nucleic acid detection of african swine fever. Front Microbiol. 2019;10:2830.
- Gentile I, Abenavoli L. Covid-19: perspectives on the potential novel global threat. Rev Recent Clin Trials. 2020 Feb 27;15(2):84–86.
- Broughton JP, Deng X, Yu G, et al. Crispr–cas12-based detection of sars-cov-2. Nat Biotechnol. 2020 Jul;38(7):870–874.
- Wang X, Zhong M, Liu Y, et al. Rapid and sensitive detection of covid-19 using crispr/cas12a-based detection with naked eye readout, crispr/cas12a-ner. Sci Bull (Beijing). 2020 May 5.
- Guo L, Sun X, Wang X, et al. Sars-cov-2 detection with crispr diagnostics. Cell Discov. 2020;6:34.
- Huang Z, Tian D, Liu Y, et al. Ultra-sensitive and high-throughput crispr-p owered covid-19 diagnosis. Biosens Bioelectron. 2020 Sep 15;164:112316.
- Joung J, Ladha A, Saito M, et al. Point-of-care testing for covid-19 using sherlock diagnostics. medRxiv. 2020: 2020.05.04.20091231.
- Batista AC, Pacheco LGC. Detecting pathogens with zinc-finger, tale and crispr- based programmable nucleic acid binding proteins. J Microbiol Methods. 2018 Sep;152:98–104.
- Wang B, Wang R, Wang D, et al. Cas12avdet: a crispr/cas12a-based platform for rapid and visual nucleic acid detection. Anal Chem. 2019 Oct 1;91(19):12156–12161.
- Kellner MJ, Koob JG, Gootenberg JS, et al. Sherlock: nucleic acid detection with crispr nucleases. Nat Protoc. 2019 Oct;14(10):2986–3012.
- Khan H, Khan A, Liu YF, et al. Crispr-cas13a mediated nanosystem for attomolar detection of canine parvovirus type 2. Chin Chem Lett. 2019 Dec;30(12):2201–2204.
- Wu Y, Liu SX, Wang F, et al. Room temperature detection of plasma epstein-barr virus DNA with crispr-cas13. Clin Chem. 2019 Apr;65(4):591–592.
- Qin P, Park M, Alfson KJ, et al. Rapid and fully microfluidic ebola virus detection with crispr-cas13a. ACS Sens. 2019 Apr 26;4(4):1048–1054.
- Li Y, Mansour H, Wang T, et al. Naked-eye detection of grapevine red-blotch viral infection using a plasmonic crispr cas12a assay. Anal Chem. 2019 Sep 17;91(18):11510–3.
- Liu Y, Xu H, Liu C, et al. Crispr-cas13a nanomachine based simple technology for avian influenza a (h7n9) virus on-site detection. J Biomed Nanotechnol. 2019 Apr 1;15(4):790–798.
- Freije CA, Myhrvold C, Boehm CK, et al. Programmable inhibition and detection of rna viruses using cas13. Mol Cell. 2019 Dec 5;76(5):826–37 e11.
- Chang Y, Deng Y, Li T, et al. Visual detection of porcine reproductive and respiratory syndrome virus using crispr-cas13a. Transbound Emerg Dis. 2020 Mar;67(2):564–571.
- Sullivan TJ, Dhar AK, Cruz-Flores R, et al. Rapid, crispr-based, field-deployable detection of white spot syndrome virus in shrimp. Sci Rep. 2019 Dec 23;9(1):19702.
- Chaijarasphong T, Thammachai T, Itsathitphaisarn O, et al. Potential application of crispr-cas12a fluorescence assay coupled with rapid nucleic acid amplification for detection of white spot syndrome virus in shrimp. Aquaculture. 2019 Oct 15;512:734340.
- Ai JW, Zhou X, Xu T, et al. Crispr-based rapid and ultra-sensitive diagnostic test for mycobacterium tuberculosis. Emerg Microbes Infect. 2019;8(1):1361–1369.
- Shen J, Zhou X, Shan Y, Yue H, Huang R, Hu J, et al. Sensitive detection of a bacterial pathogen using allosteric probe-initiated catalysis and crispr-cas13a amplification reaction. Nat Commun. 2020 Jan 14;11(1):267.