ABSTRACT
Coxsackievirus A16 (CV-A16) is a major causative pathogen of hand, foot, and mouth diseases (HFMDs). The licensed HFMD vaccine targets EV-A71 without cross-protection against CV-A16. Thus, a CV-A16 vaccine is needed. In this study, the immunogenicity and protective efficacy of a live attenuated CV-A16 candidate, K168-8Ac, were evaluated in a rhesus monkey model. Four passages of this strain (P35, P50, P60, and P70) were administered to monkeys, and its protective effect was identified. The immunized monkeys were clinically asymptomatic, except for slight fever. Weak viraemia was observed, and two doses of vaccination were found to significantly reduce virus shedding. High levels of antibody responses were observed (1:1024–1:2048), along with a significant increase in plasma IL-8. The I.M. group showed a much stronger humoural immunity. Pathological damage was detected mainly in lung tissues, although thalamus, spinal cord, lymph nodes, and livers were involved. After the viral challenge, it was found that two doses of vaccine reduced virus shedding, and the degree of lung damage and the number of organs involved decreased as the passage number increased. Overall, a robust immune response and partial protection against CV-A16, triggered by the K168-8Ac strain, were demonstrated. This study provides valuable data for CV-A16 vaccine development.
Introduction
Hand, foot, and mouth disease (HFMD) has received considerable attention in the past decade in China [Citation1–3]. The first HFMD outbreak dates back to as early as 1957 in New Zealand [Citation4]. The 2008 epidemic of HFMD in mainland China is by far the largest outbreak of this disease [Citation5]. As of June 2019, over 21 million HFMD cases were reported in China [Citation6]. HFMD is a common contagious disease among children aged <5 years and occurs sporadically in adults [Citation1,Citation7,Citation8]. Clinical manifestations of HFMD include fever, vesicles on hands, feet, mouth, anus, and central nervous system diseases such as aseptic meningitis, encephalitis, and neurogenic pulmonary oedema.
To date, more than 20 pathogens have been associated with HFMD. Amongst these, enterovirus 71 (EV-A71) and coxsackievirus A16 (CV-A16) are the two most common pathogens. Although several inactivated EV-A71 vaccines have been marketed successfully in China [Citation9,Citation10], their cross-protection efficacy against other HFMD pathogens, including CV-A16, have not been recognized [Citation11]. Therefore, the CV-A16 vaccine development is of great significance to control HFMD.
Live attenuated vaccine represents an attractive vaccine development strategy. In our previous study, we isolated ∼30 CV-A16 strains from clinical faecal samples. These CV-A16 isolates were adapted to a human lung diploid embryonic cell line, KMB17, by serial passage. According to several evaluation metrics, such as virus proliferation and immunogenicity, two strains (K154 and K168-8Ac) were selected as the vaccine candidate strains in our previous studies [Citation12]. Subsequently, we found that 17th passage (P17) of K168-8Ac strain on KMB17 displayed an attenuated-virulence phenotype in mice; however, histopathological examination showed mild inflammatory infiltration in the myocardial interstitium [Citation13]. Therefore, in this study, higher passages of K168-8Ac strain (P35, P50, P60, and P70) were chosen for evaluation of their safety and efficacy in a non-human primate model.
Material and methods
Viruses, cells, and serial passages
Two CV-A16 strains, K168-8Ac (GenBank: KY088084.1) and K154, were isolated and identified by our group from HFMD patients in Kunming Children’s Hospital, Yunnan, China. K168-8Ac strain was used as the parental strain for the live attenuated vaccine, and K154 strain was used in the in vivo challenge experiments. KMB17 and Vero cells are commonly used as cell matrix for vaccine production in China [Citation14–17]. These two cell lines were provided by the Department of Quality Control, the Institute of Medical Biology, Chinese Academic of Medical Sciences (CAMS). KMB17 cells and Vero cells were grown in Dulbecco’s modified Eagle’s medium (Gibco, Indianapolis, OH, USA), supplemented with 10% foetal bovine serum. K168-8Ac strain was serially passaged in KMB17 cells. Each passage was titrated using Vero cells as described in our previous studies [Citation18,Citation19]. Mycoplasma contamination in the passages was determined using a commercial kit (Yise, Shanghai, China). The list of viruses used in this study is shown in .
Table 1. Assays on the CV-A16 viruses for use in in vivo experiments.
Sequencing of the whole genome of the four passages of K168-8Ac strain
To determine the genetic stability of K168-8Ac strain, the whole genomes of four viruses and earlier passages in KMB17 cells along with their parental virus propagated in Vero cells were sequenced. Seven pairs of primers (Table S1) were designed to amplify the genomes using a PrimeScript One-Step RT–PCR kit (Takara, Japan) according to the manufacturer’s instruction. DNA products were sequenced using a commercial corporation (TSINGKE). The editing and alignments of the sequences were performed using DNAstar version 7.1.
Rhesus monkeys
Male rhesus monkeys aged 5–10 months at immunization were used in this study. All experimental procedures and animal care protocols were approved by the Yunnan Provincial Experimental Animal Management Association (approval number: SYXK (Dian) K2015-0006) and the Experimental Animal Ethics Committee of the Institute of Medical Biology, CAMS (approval number: [2016] 59). Before beginning the study, all monkeys were confirmed to be negative for CV-A16 antibodies.
Immunization and challenge
A total of 26 monkeys were randomly assigned to four experiment groups (with six monkeys per group), and to one non-immunization control group, respectively. The intramuscular, intranasal, and oral routes of vaccine administration were used within each experiment group. The vaccination schedule is shown in . Considering that intranasal or oral immunization routes could not induce high production of the CV-A16 neutralizing antibody, we performed the subsequent challenge experiment on the four monkeys vaccinated via the intramuscular route on day 14 after the secondary immunization. In this experiment, two additional monkeys were used as a challenge control without immunization. K154 strain was administered as a challenge virus, at a dose of 7.37 CCID50 (50% cell culture infective dose) per monkey ().
Table 2. Experiment scheme for evaluating the safety and protection of the CV-A16 candidate attenuated strain in rhesus monkeys.
Viral quantification by RT-qPCR
Supernatants obtained from the faeces or plasma samples were used to extract viral RNA for virus quantification, using a commercial kit (Axygen, NY, USA). One-step RT-qPCR (Takara, Dalian, China) was performed using a fluorescence quantitative PCR instrument (Bio-Rad Laboratories, Hercules, CA, USA), according to the manufacturer’s instructions. Primer sequences were as follows: Forward: acactccattaccctgagggtgta; Reverse: agggttgatttctcagaggtcttg. Probe: 5′FAM-atgagaatcaaacacgtcagggcatggat-3′TAMRA.
Neutralization assays
Serum samples were inactivated at 56°C for 20 min before use. The neutralizing activity of serum was measured in 96-well culture plates using the micro-cytopathic method using Vero cells, as described previously [Citation19].
Cytokine and chemokine detection
Interferon-γ (IFN-γ), interleukin-4 (IL-4), IL-6, IL-8, and tumour necrosis factor-α (TNF-α) were quantified as representative cytokines and chemokines, using the Luminex-200 System (Luminex, Austin, TX, USA) with a customized Milliplex Kit (Millipore, MA, USA), according to the manufacturer’s instruction. Data analysis was done using the Milliplex Analyst 5.1 software.
Statistical analysis
The IBM SPSS 22.0 statistical software was used for all statistical analyses. The independent samples t-test or one-way analysis of variance – was used for comparisons between two groups or more than two groups, respectively. For non-normal distribution data, the non-parametric Mann–Whitney U-test or Kruskal–Wallis test was used. A value of P < .05 was considered statistically significant.
Results
Five nucleotide variations in whole genome among the four passages of K168-8Ac strain
We identified five nucleotide variations between these viruses. These were located in two structural regions, VP2 and VP3, and in a non-structural 2C region (). Four nucleotide changes within VP2 and VP3 region were missense mutations, while the one within 2C region was synonymous mutation. When the virus culture matrix was switched to KMB17, only one amino acid substitution was observed. Compared to the earlier passage of K168-8Ac strain, propagated in KMB17 cells, higher passages showed three amino acid changes in the 141st and 226th positions in the VP2 region and in the 29th position in the VP3 region.
Table 3. Comparison of whole-genome sequences of these different passages of the K168-8Ac strain.
Rhesus monkeys inoculated with all K168-8Ac passages were clinically asymptomatic except for slight fever
None of the immunized monkeys showed typical clinical signs of HFMD. The rectal temperature of monkeys inoculated with P35 increased significantly by 0.36°C at days 2–4 post-first immunization (t = 4.824, P < .01) compared with that at the baseline, and no obvious change was seen after second immunization ((A)). The rectal temperature of monkeys inoculated with P50 was mildly elevated at 4–6 days post-first immunization (t = 2.933, P < .05) ((A)). However, the monkeys inoculated with P60 or P70 did not have fever ((A)). Monkeys immunized via the intramuscular or oral routes showed a higher rectal temperature at the indicated time points than the baseline. In contrast, monkeys those immunized via nasal route did not have fever ((A)).
Figure 1. Immunization with four passages of K168-8Ac strain to elicit immune response of CV-A16. (A) The rectal temperature of the rhesus macaques immunized with four passages. Each monkey was daily monitored after primary immunization (n = 6) and after secondary immunization (n = 3) (*P < .05, **P < .01). (B) Plasma viral load in the immunized rhesus macaques with four passages by qPCR. Within 14 days, post-primary immunization (n = 6) and at other time points (n = 3) were shown. (C) CV-A16-specific neutralization antibody levels of the immunized rhesus macaques with four passages administered through intramuscular injection. (D) Viral shedding in stools collected from the immunized rhesus macaques administered with four passages. Viral shedding within 14 days post-primary immunization (n = 6) and at other indicated time points (n = 2) is shown. (E) Quantification analysis of five cytokines or chemokines in plasma of the immunized rhesus macaques administered with four passages. Data were collected during 5–27 days after primary immunization (n = 6) and during 3–15 days after secondary immunization (n = 3).
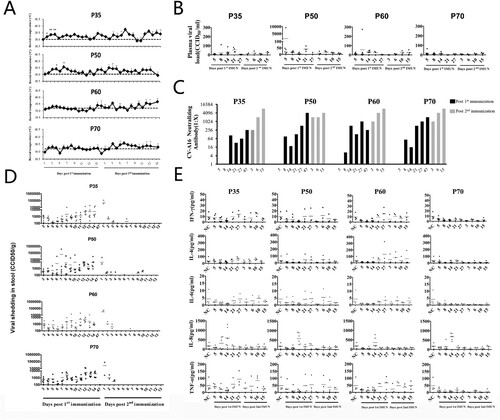
Figure 2. Immunization with K168-8Ac strain via three routes to elicit immune response of CV-A16. (A) The rectal temperature of rhesus macaques immunized via three routes. Each monkey was daily monitored after primary immunization (n = 4) and after secondary immunization (n = 2) (*P < .05). (B) Plasma viral load in immunized rhesus macaques via three routes. Viral load within 14 days post- primary immunization (n = 8) and other time points (n = 4) is shown. (C) CV-A16-specific neutralization antibody levels of the rhesus macaques immunized via three routes after primary immunization (n = 8) and after secondary immunization (n = 4). (D) Viral shedding in stool collected from rhesus macaques via three routes. Viral shedding within 14 days post-primary immunization (n = 8) and at other indicated time points (n = 4) is shown. (E) Quantification analysis of five cytokines or chemokines in plasma from the rhesus macaques immunized via three routes. Data were collected during 5–27 days after primary immunization (n = 8) and during 3–15 days after secondary immunization (n = 4).
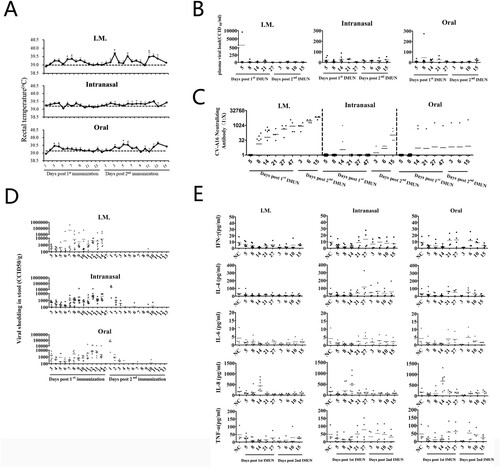
Weak viraemia in the inoculated rhesus monkeys
Among the four passages, the viral load in the plasma of monkeys inoculated with P35 showed a detectable viraemia at days 8–21 after the initial immunization. A transient significant viraemia was observed in ∼16.7% of the animals (1/6) immunized with P50 or P60 on day 5 or day 8 after initial immunization, while the levels of viral load in monkeys immunized with P70 were undetectable ((B)). After secondary immunization, the viral loads in all monkeys were less than 100 CCID50/ml ((B)).
Amongst the eight monkeys immunized via the intramuscular or oral route, one monkey developed transient plasma viraemia of more than 100 CCID50/ml after the initial immunization ((B)). In contrast, all immunized monkeys via the intranasal route showed a lower plasma viraemia (less than 100 CCID50/ml) ((B)).
Shortened faecal viral shedding in monkeys after secondary immunization with the P35, P50, P60, or P70 passage
Continuous faecal virus shedding occurred within 14 days after the initial immunization in all the groups. There were two peaks post-first immunization on days 7–8 and days 12–14 post-first immunization, respectively ((D) and (D)). Interestingly, the duration of virus shedding post-second immunization was shortened to 3 days in all passage groups; however, the virus shedding, after secondary immunization, was undetectable in the intramuscular group. No significant difference was observed in the peak value of virus shedding in these groups (P > .05).
Significantly enhanced plasma IL-8 levels in monkeys after initial immunization with the P35, P50, P60, or P70 passage
Except for IL-8, no statistically significant difference was observed between the levels of IFN-γ, IL-4, IL-6, and TNF-α before and after vaccination ((E) and (E)).
In the four passages, plasma levels of IL-8 were up-regulated by 4.9–10.6 times in 50% (3/6) of the monkeys immunized with P35 on day 8 after initial immunization compared with those at day 5 (P = .017), and this increased by 8.4–15.6 times on day 14 after initial immunization ((E)). The IL-8 levels on day 14 after initial immunization with P50, P60, or P70 were up-regulated in 100% (6/6) of the monkeys by 2.9–24.1, 2.8–40.0, and 3.8–66.8 times, respectively ((E)). Furthermore, the IL-8 levels in the P35 group were significantly lower than that in the P60 group (P = .019), whereas no statistical difference was observed among the P50, P60, and P70 groups.
The levels of IL-8 increased by 2.8–66.9 times in 75% (6/8) of the monkeys inoculated via intramuscular injection on day 8 after the initial immunization compared with those on day 5 (t = −3.424, P = .014) ((E)). Approximately 85% (6/7) of the monkeys that received intranasal inoculation exhibited increase in the IL-8 levels by 3.8–24.1 times, while oral administration resulted in up-regulation of the IL-8 levels by 3.5–40.0 times ((E)). There was no significant difference in plasma IL-8 levels of the monkeys that were inoculated via the three routes.
Varying degree of histopathological changes on thalamus, spinal cord, lymph nodes, lung, and liver tissues in monkeys inoculated with P35, P50, P60, or P70 passage
On day 14 post-first immunization, one monkey in each group was sacrificed for histopathological examination. As shown in , vascular engorgement in lateral ventricular choroid plexus and few glial nodules or lymphocytic cuffs were observed in the thalami of monkeys inoculated with P35, P50, or P60 via three various routes ((A)). Glial nodules were also observed both in lumbar and cervical spinal cord in the monkeys immunized with P35 or P70 via intramuscular or intranasal routes. Phagocytosis or vacuolation of individual neurons was observed in individual sections of spinal cord ((B)). Congestion around individual lymph follicles of alveolar, axilla, or submaxillary lymph nodes was observed in monkeys vaccinated with P35 virus by intramuscular or intranasal route, or with P60 virus by oral or with P70 virus by intranasal ((E)). Of note, the pathological images indicated obvious lung injury, including alveolar wall thickening, haemorrhage, and pulmonary oedema in most groups ((C)). In the liver, lymphocytic infiltrates were identified in the portal areas or parenchyma ((D)).
Figure 3. Histopathological changes in the thalamus, spinal cord, lung, liver, and lymph nodes of rhesus macaques immunized with K168-8Ac passages via three routes (200×). (A) Thalamus: vascular congestion in the choroid plexus of the thalamus following P35 inoculation in the intramuscular or oral route group and perivascular lymphocyte cuffing in the local thalamic following P50 inoculation in the intramuscular or intranasal group and following P60 inoculation in the intramuscular group. (B) Spinal cord: gliosis, individual phagocytosed neurons by microglia and perivascular lymphocyte cuffing in the lumbar section of the spinal cord in the P35 in the intramuscular group; multiple glial nodules in the lumbar section of the spinal cord following P50 inoculation in the intramuscular group and a vacuolated neuron in the lumbar section of the spinal cord following P70 inoculation in the intranasal group. (C) Lungs: Thickening of the alveolar walls and very few inflammatory cells in the lungs following P35 inoculation in the intramuscular group; enlargement of individual lymphoid follicles in the interstitium of the lungs following P35 inoculation in the oral group and P50 inoculation in the intramuscular group; mild or moderate alveolar congestion following P50 inoculation in the oral or intranasal group; and consolidation of individual alveolar cavity following P60 inoculation in the intranasal group and congestion and oedema in local alveolar wall following P60 inoculation in the oral group and P70 in the intranasal group.(D) Liver: A small amount of inflammatory cell infiltration in liver parenchyma following P35, P60 or P70 inoculation in the oral group and infiltration of few inflammatory cells in liver parenchyma or hepatic portal area following P50 or P70 inoculation in the intramuscular group and P60 inoculation in the intranasal group. (E) Lymph nodes: Haemorrhagic perifollicular in pulmonary lymph nodes following P35 inoculation in the intramuscular or intranasal group, haemorrhagic perifollicular in axilla lymph nodes following P35 or P70 inoculation in the intranasal group and P60 inoculation in the oral group, and haemorrhagic perifollicular in submandibular lymph nodes following P35 inoculation in the intranasal group or P60 inoculation in the oral group. Scale bars, 100 µm.
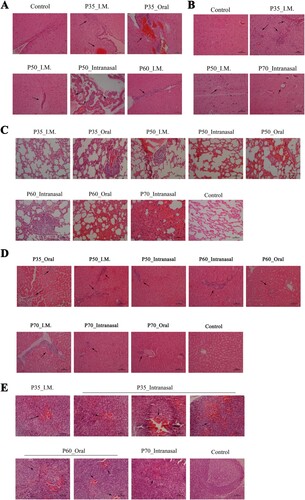
Stronger neutralizing antibody responses induced by P35, P50, P60, or P70 passage intramuscular route than through intranasal or oral route
Immunization with only one dose of P35, P50, P60, or P70 by intramuscular route induced high levels of neutralizing antibody after two doses ((C)). However, intranasal or oral immunization was found to be far less potent in inducing a neutralizing antibody response ((C), P<0.5). Considering the lower limit of neutralizing assay detection (1:8) as the positive threshold for the antibody response, we found the positive rate of antibody in the intramuscular group on day 14 after first immunization was 75% (6/8), and the rates in the intranasal group and in the oral group were 25% (2/8) each. By day 14 after secondary immunization, the intramuscular or intranasal group displayed a significantly higher positive rate than the oral group (χ2 = 7.526, P = .01). No statistically significant difference in antibody titres was observed among P35, P50, P60, and P70 groups.
Partial protective effect induced by P35, P50, P60, and P70 passage
Hands and feet of the non-vaccinated monkeys sporadically developed herpes after the challenge infection. Swab samples of the blisters were found to be negative for CV-A16 nucleic acid. No clinical signs of diseases were observed in the vaccinated animals after challenge.
Modest fever was observed in all the monkeys ((A)). One potential concern when using a protective vaccine is the effective inhibition of virus replication. In the current study, low plasma viraemia (<2 Log CCID50/ml) was detected, as determined by RT-qPCR, in both vaccinated and mock monkeys ((B)); hence, it was difficult to determine any inhibitory effect.
Figure 4. Protective responses induced by K168-8Ac passages. (A) Changes in rectal temperature of immunized or unimmunized rhesus macaques after being challenged. (B) Plasma viral load was quantified by qPCR in the immunized or unimmunized rhesus macaques. (C) Faecal virus shedding was determined by qPCR in immunized or unimmunized rhesus macaques. (D) Levels of neutralizing antibody after being challenged in immunized or unimmunized rhesus macaques. (E) Pathological changes of lung after being challenged in immunized or unimmunized rhesus macaques (scale bars, 100 µm).
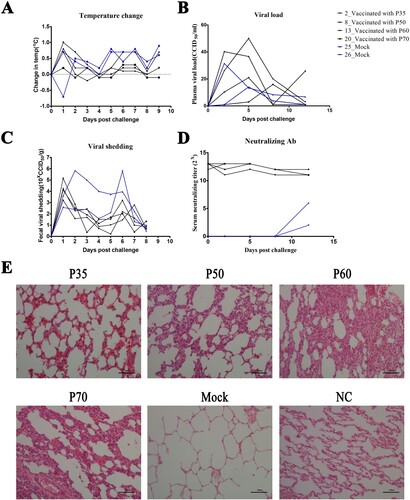
Faecal viral shedding was daily monitored for 14 days after the challenge. It was first detected on day 1 after challenge in vaccinated monkeys, but was not detectable at other time points ((C)). In contrast, of these two controls, one (No. 25) showed the prolonged duration of viral shedding, which continued for 8 days. The viral shedding amount in the vaccinated group ranged from 1.6 × 103 CCID50/g to 1.42 × 105 CCID50/g, while it peaked higher than 6.0× 105 CCID50/g in the mock monkeys. On day 12 after challenge, the animals were sacrificed for pathological examination. The vaccinated group showed clear and intact lung tissue structures, showing oedema or thickening of the alveolar wall after challenge ((E)), while pulmonary injury was exacerbated in the mock monkeys, as indicated by pulmonary oedema, congestion of alveolar, dilated alveolar spaces, and alveolar wall rupture ((E)). The CV-A16 neutralizing antibody levels were monitored within 1–12 days after challenge. It was observed that the neutralizing antibodies were maintained at a high level in the vaccinated group ((D)), which were similar to the levels before challenge. In contrast, a low-level antibody response against CV-A16 was detected ((D)), as expected, in the mock group because of the use of nasal and oral routes with a single dose in the challenge experiments.
Discussion
Currently, no CV-A16 vaccine candidates have entered clinical trials. Although the earlier studies have shown that the formalin-inactivated CV-A16 vaccine exerts protective effects in rodents [Citation20], this vaccine did not confer protection from infection in rhesus macaques [Citation21]. In addition, while studies on other types of CV-A16 vaccines including VLP vaccines [Citation22,Citation23], multipeptide vaccines [Citation24], and multivalent vaccines [Citation25,Citation26] have been carried out in rodents, there is no study conducted in non-human primates. To the best of our knowledge, this study was the first attempt to explore the safety and efficacy of a live attenuated CV-A16 vaccine candidate developed by our group in a non-human primate.
Our results showed that the K168-8Ac strain elicited a specific high-titre antibody response in the immunized monkeys ((C)). Interestingly, it did not demonstrate highly potent protection against the viral challenge ((D)). Several studies have reported that specific antibody response is significantly effective in repelling small RNA viral infections [Citation27]. We thus speculated the CV-A16-specific antibodies, induced by this vaccine candidate, may not be able to adequately perform their primary function in vivo. Two observations supported this speculation. First, vaccine-induced specific antibodies have a strong neutralizing ability in vitro, whereas this neutralization capacity wanes in vivo (E), thus suggesting that these antibodies display a narrow spectrum of neutralizing activity. Second, although the serum antibodies are usually in direct contact with the “naked” virus in the in vitro neutralizing assay, in vivo experiments, the viruses may not be “naked”. It is reported that some specific non-enveloped viruses, such as hepatitis A virus [Citation28] and coxsackievirus B [Citation29,Citation30], can achieve cell-to-cell transmission via a different non-lytic mechanism by integrating into the host cell membrane to protect viruses from antibody-mediated neutralization. Thus, we could detect only few viruses in the plasma samples (B and B).
Besides specific antibody responses, we also studied cytokine and chemokine production profiles. We found that plasma levels of IFN-γ, IL-4, IL-6, and TNF-α showed no significant difference before and after vaccination (E and E). This observation was consistent with the findings of Wang [Citation31] in young rhesus monkeys, using live CV-A16 viruses. Interestingly, a markedly elevated serum level of IL-8 at days 5–14 was observed, after first vaccination with K168-8Ac (E and E). Whole-genome expression profiling analysis in rhesus monkeys showed that IL-8 might be one of the key genes involved in regulating immune defence and inflammation in response to CV-A16 [Citation32]. In other viral infections, IL-8, an important chemokine, has been shown to augment viral replication by counteracting interferon-mediated antiviral activity [Citation28,Citation29]. It is noteworthy that in our study, lung inflammation or damage (C and E) was accompanied by increased IL-8 secretion, thus, suggesting a potential correlation between them. Similar correlation has been also found in other viral infections, such as porcine respiratory syndrome virus [Citation21]. The up-regulation of IL-8 may be induced by certain viral proteins via endoplasmic reticulum stress [Citation33]. In addition, it has been reported that transcription factors such as nuclear factor kappa B and AP-1, or TAK-1/JNK/AP-1 pathway may also be involved in the up-regulation of IL-8 [Citation34]. It would be interesting to investigate the biological functions of IL-8 in CV-A16 infection in future studies.
In this study, we identified four amino acid changes among five passages of K168-8Ac strain prepared in KMB17 cells and its parental strain prepared in Vero cells. One of these variations was at the 141st position within VP2 region converted Asn in P35 or P50 passage to Asp in P60 or P70 passage. Xu et al. demonstrated that VP2 epitopes (aa141–155) were immunodominant between EV-A71 and CV-A16 [Citation17]. In this study, we further determined that a single mutation at aa141 (a change from N to D) may not result in a differential specific antibody response in vivo (C), and did not affect virus replication in a sensitive cell line (). However, greater number of organs showed pathological changes in monkeys immunized with P35 or P50 viruses than with P60 or P70 viruses, and more severe pathological damages were observed in earlier passages. These observations suggest that the candidate CV-A16 strain attenuates pathological alterations, especially lung injuries, and thereby improves the condition of the infected animals. However, further investigations are required to determine whether the substitution is responsible for the observed change in the virulence phenotype.
In summary, a robust immune response and partial protection against CV-A16, induced by a previous isolated live attenuated strain, K168-8Ac, were demonstrated in this study. These findings provide additional evidence that live attenuated vaccine could be a promising effective prophylactic strategy against CV-A16. Certainly, several limitations to this pilot study need to be acknowledged. First, the current investigation could not determine the safe and most effective passage of K168-8Ac strain owing to the limited number of monkeys, although the attenuation of virulence in higher passages was observed. Second, some important issues in the live attenuated vaccine were not addressed in this study, for example, whether this strain can induce protective mucosal immunity or whether the excreted CV-A16 virus can be horizontally transmitted.
Supplementary_table.doc
Download ()Acknowledgements
We are grateful to Yun Li, Lipeng Zhou, Qingling Wang, and Yunguang Hu in our institute for their extensive assistance. This work was supported by the CAMS Innovation Fund for Medical Sciences (2016-I2M-1-019), Fundamental Research Funds for the Central Universities (3332019110), and Yunnan Applied Basic Research Projects (2015BC008).
Disclosure statement
No potential conflict of interest was reported by the author(s).
Additional information
Funding
References
- Xing W, Liao Q, Viboud C, et al. Hand, foot, and mouth disease in China, 2008-12: an epidemiological study. Lancet Infect Dis. 2014;14(4):308–318.
- Baggen J, Thibaut HJ, Strating J, et al. The life cycle of non-polio enteroviruses and how to target it. Nat Rev Microbiol. 2018;16(6):368–381.
- Gonzalez G, Carr MJ, Kobayashi M, et al. Enterovirus-associated hand-foot and mouth disease and neurological complications in Japan and the rest of the world. Int J Mol Sci. 2019;20:5201.
- Seddon JH, Duff MF. Hand-foot-and-mouth disease: Coxsackie virus types A 5, A 10, and A 16 infections. N Z Med J. 1971;74(475):368–373.
- Zhang Y, Zhu Z, Yang W, et al. An emerging recombinant human enterovirus 71 responsible for the 2008 outbreak of hand foot and mouth disease in Fuyang city of China. Virol J. 2010;7:94.
- Zhang Y, Liu H, Wang L, et al. Comparative study of the cytokine/chemokine response in children with differing disease severity in enterovirus 71-induced hand, foot, and mouth disease. PLoS One. 2013;8(6):e67430.
- Murase C, Akiyama M. Hand, foot, and mouth disease in an adult. N Engl J Med. 2018;378(14):e20.
- Reich M, Cakir B, Cvetkoski S, et al. Acute unilateral maculopathy associated with adult onset of hand, foot and mouth disease: a case report. BMC Ophthalmol. 2019;19(1):104.
- Li R, Liu L, Mo Z, et al. An inactivated enterovirus 71 vaccine in healthy children. N Engl J Med. 2014;370(9):829–837.
- Wang W, Li W, Yang X, et al. Interleukin-8 is elevated in severe hand, foot, and mouth disease. J Infect Dev Ctries. 2014;8(1):94–100.
- Yang L, Liu Y, Li S, et al. A novel inactivated enterovirus 71 vaccine can elicit cross-protective immunity against coxsackievirus A16 in mice. Vaccine. 2016;34(48):5938–5945.
- Jiang GJ. The selection of candidate strain of attenuated live coxsackievirus A16 vaccine [master's degree]. Beijing: Chinese Academy of Medical Sciences & Peking Union Medical College; 2014.
- Yang SZ. Attenuated characteristics and bioprocess assessment of CA16 vaccine candidates [master's degree]. Beijing: Chinese Academy of Medical Sciences & Peking Union Medical College; 2015.
- Guo R, Cao YY, Dai ZZ, et al. [Characteristics of a human diploid cell line, KMB-17]. Zhongguo yi xue ke xue yuan xue bao Acta Academiae Medicinae Sinicae. 1981;3(4):226–230.
- Liang Y, Che Y, Yang B, et al. Immunogenicity and safety of an F-genotype attenuated mumps vaccine in healthy 8- to 24-month-old children. J Infect Dis. 2019;219(1):50–58.
- Mao JS, Dong DX, Zhang HY, et al. Primary study of attenuated live hepatitis A vaccine (H2 strain) in humans. J Infect Dis. 1989;159(4):621–624.
- Xu L, He D, Yang L, et al. A broadly cross-protective vaccine presenting the neighboring epitopes within the VP1 GH loop and VP2 EF loop of enterovirus 71. Sci Rep. 2015;5:12973.
- Zhongping X, Hua L, Ting Y, et al. Biological characteristics of different epidemic enterovirus 71 strains and their pathogeneses in neonatal mice and rhesus monkeys. Virus Res. 2016;213:82–89.
- Yang T, Li H, Yue L, et al. A comparative study of multiple clinical enterovirus 71 isolates and evaluation of cross protection of inactivated vaccine strain FY-23 K-B in vitro. Virol J. 2017;14(1):206.
- Yang E, Cheng C, Zhang Y, et al. Comparative study of the immunogenicity in mice and monkeys of an inactivated CA16 vaccine made from a human diploid cell line. Hum Vaccin Immunother. 2014;10(5):1266–1273.
- Wang J, Zhang Y, Zhang X, et al. Pathologic and immunologic characteristics of coxsackievirus A16 infection in rhesus macaques. Virology. 2017;500:198–208.
- Gong M, Zhu H, Zhou J, et al. Cryo-electron microscopy study of insect cell-expressed enterovirus 71 and coxsackievirus a16 virus-like particles provides a structural basis for vaccine development. J Virol. 2014;88(11):6444–6452.
- Feng Q, He Y, Lu J. Virus-like particles produced in Pichia pastoris induce protective immune responses against Coxsackievirus A16 in mice. Med Sci Monit: Int Med J Exp Clin Res. 2016;22:3370–3382.
- Shi J, Huang X, Liu Q, et al. Identification of conserved neutralizing linear epitopes within the VP1 protein of coxsackievirus A16. Vaccine. 2013;31(17):2130–2136.
- Huo C, Yang J, Lei L, et al. Hepatitis B virus core particles containing multiple epitopes confer protection against enterovirus 71 and coxsackievirus A16 infection in mice. Vaccine. 2017;35(52):7322–7330.
- Lim H, In HJ, Lee JA, et al. The immunogenicity and protection effect of an inactivated coxsackievirus A6, A10, and A16 vaccine against hand, foot, and mouth disease. Vaccine. 2018;36(24):3445–3452.
- Anastasina M, Domanska A, Palm K, et al. Human picornaviruses associated with neurological diseases and their neutralization by antibodies. J Gen Virol. 2017;98(6):1145–1158.
- Feng Z, Hensley L, McKnight KL, et al. A pathogenic picornavirus acquires an envelope by hijacking cellular membranes. Nature. 2013;496(7445):367–371.
- Robinson SM, Tsueng G, Sin J, et al. Coxsackievirus B exits the host cell in shed microvesicles displaying autophagosomal markers. PLoS Pathog. 2014;10(4):e1004045.
- Sin J, McIntyre L, Stotland A, et al. Coxsackievirus B escapes the infected cell in ejected mitophagosomes. J Virol. 2017;91(24):e01347–17.
- Wang J. Study of coxsackievirus A16-infected rhesus monkeys macaques model and immunologic characteristics [dissertation]. Beijing: Chinese Academy of Medical Sciences & Peking Union Medical College; 2017.
- Song J. Global gene expression analysis of rhesus monkey infected by CA16 and the study on autophagy elicited by CA16 infection supresses IFN-I production [dissertation]. Beijing: Chinese Academy of Medical Sciences & Peking Union Medical College; 2016.
- Tsuge M, Hiraga N, Zhang Y, et al. Endoplasmic reticulum-mediated induction of interleukin-8 occurs by hepatitis B virus infection and contributes to suppression of interferon responsiveness in human hepatocytes. Virology. 2018;525:48–61.
- Liu Y, Du Y, Wang H, et al. Porcine reproductive and respiratory syndrome virus (PRRSV) up-regulates IL-8 expression through TAK-1/JNK/AP-1 pathways. Virology. 2017;506:64–72.