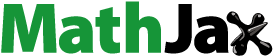
ABSTRACT
The current study explored various antioxidant properties and HPLC-DAD analysis of ethylacetate and aqueous extracts from Talinum triangulare (T. triangulare) as well as their inhibitory potentials on exzymatic activities implicated in type II diabetes. In this study, quantification of total antioxidant abilities revealed that ethylacetate and aqueous extracts of T. triangulare had 11.52 ± 0.78 and 2.46 ± 0.26 mg (AAE/g), respectively. However, both extracts showed metal-chelating ability but aqueous extract had higher inhibition against Fe2+ (IC50 = 0.50 ± 0.01 mg/ml) than ethylacetate extract (IC50 = 0.57 ± 0.01 mg/ml). There was no significant (p > 0.05) difference observed in their inhibitory activities against α-amylase activity, while ethylacetate extract had a strong inhibition (IC50 = 0.17 ± 0.01 mg/ml) on α-glucosidase activity than the aqueous extract (IC50 = 0.85 ± 0.01 mg/ml) in a concentration-dependent manner. Also, higher inhibition was noticed in ethylacetate extract against monoamine oxidase (IC50 = 99.72 ± 0.01 mg/ml) than the aqueous extract (IC50 = 141.08 ± 0.02 mg/ml). In addition, various polyphenolic contents were revealed via HPLC-DAD analysis in the extracts which could have engendered various inhibitions observed. Therefore, it could be suggested that, both extracts if properly annexed, could be promising alternative in the management of diabetes mellitus.
Introduction
Diabetes mellitus (DM) is a chronic impairment in the carbohydrate and lipid metabolism, resulting from a defect in the insulin secretion or biological factors responsible for its activity [Citation1–Citation3]. According to the World health organization (WHO), DM is the main cause of global mortality and a principal cause of cardiovascular diseases, characterized by endothelial dysfunction and accelerated atherosclerosis [Citation4]. However, type 2 diabetes (non-insulin dependent), which accounts for over 95% of the world diabetic population is prevalently common in most parts of the world than type 1 diabetes (insulin-dependent) [Citation5,Citation6].
Novel studies have shown the involvement of oxidative stress in the etiology and pathogenesis of DM via pancreatic tissue damage [Citation7]. Auto-oxidation of glucose in mitochondrial and the catalytic activities of Fe2+ in the generation of free radicals through Fenton’s reaction have been reported as vital possible sources of reactive oxygen species (ROS) that contribute to antioxidant system depletion in the pancreatic tissue [Citation8,Citation9], this in turns, impairs insulin secretion and subsequently abnormality of glucose homeostasis in the adipose tissues [Citation10].
Recently, it has been shown that, the catalytic activities of biologically active monoamine oxidases (MAO) in the deamination of aliphatic and aromatic amines in the parenchymatous tissues of vertebrates also contribute to the generation of free radicals [Citation11], which has directly been connected with mitochondrial, endothelial and contractile dysfunction [Citation12,Citation13]. However, a large amount of ROS are often produced in the mitochondrial as a result of several mutations leading to disturbances in the respiratory chain as well as the loss of control of ROS production at the level of respiratory complexes [Citation14]. Similarly, excessive ROS formation, increased levels of malonylaldehyde (MDA) and protein oxidation in the adipose tissues have hallmarked increased activities of MAO in the mitochondrial of a subject with insulin-specific receptor gene knock-out, signaling mitochondrial dysfunction [Citation15].
Many indigenous medicinal plants have been found successfully effective in the management of DM [Citation16]. The roles of natural polyphenolic compounds have been emphasized in mediating inhibition on carbohydrate-hydrolyzing enzymes activities as well as militating against the formation of/and scavenging the ROS which are key targets in the management of type 2 diabetes [Citation17].
T. triangulare was used in the current study. The plant is popularly known as waterleaf and one of the most locally consumed vegetable in Nigeria [Citation18]. Studies have shown that the plant is naturally rich in contents like flavonoids and polyphenols [Citation19]. Likewise, Afolabi et al. [Citation20], also revealed various antioxidant potentials of solvent-extractable fractions of aeriel leaves of the plant [Citation21]. Therefore, based on the evidence of these previous studies, this work was designed to also appraise the antioxidants and antidiabetic potentials of ethylacetate and aqueous extracts of T. triangulare.
Materials and methods
Chemicals and reagents used
The chemicals and reagent used such as methanol, acetic acid, caffeic acid and chlorogenic acid were purchased from Merck (Darmstadt, Germany). Quercetin, rutin, kaempferol, apigenin, luteolin, Intestinal α-glucosidase, thiobarbituric acid (TBA), pancreatic α-amylase, p-nitrophenyl-α-D-glucopyranose (pNPG), methanol, formic acid, sodium carbonate (Na2CO3), sodium hydroxide (NaOH), aluminum chloride (AlCl3), iron II sulfate (FeSO4) were all of analytical grade acquired from Sigma Chemical Co. (St. Louis, MO, USA).
Preparation of the samples
The fresh aerial leaves of T.triangulare were bought from a popular area called Olorunda in Ado-Ekiti, Ekiti State, Nigeria. A voucher specimen was taken to the Department of Plant Science in Ekiti State University, Ado-Ekiti, Ekiti State, Nigeria for identification, it was identified with a given herbarium number: UHAE 2013/76 after a proper taxonomic investigation from the database.
Preparation of the extracts
Preparation of aqueous extract
The leaves of T.triangulare were air-dried to a constant weight in a ventilated place at an ambient temperature of 30 ± 2°C for 15 days, then pulverized using a laboratory blender and the fine-powdered sample obtained was used. The blended air‑dried sample (50 g) of T.triangulare was soaked in 500 mL distilled water for 48 h. The mixture was filtered and concentrated in water bath at 60°C to dryness.
Preparation of Ethylacetate extract
The powdered sample was weighed and soaked in a solvent combination of 70% ethanol and distilled water for 72 h, the mixture was filtered and the filtrate concentrated at 50°C. About 5 g of the ethanolic extract was reconstituted in distilled water and then defatted with Pet-ether, the fat-free aqueous residue was partitioned repeatedly with ethylacetate in a separating funnel flask until exhaustion.
Preparation of tissue homogenates for the in vitro study
Albino rats of weight ranging from 100 to 115 g were used for this study. The rats were decapitated under mild diethyl-ether anesthesia and the brain tissues were rapidly isolated and placed on ice and weighed. These tissues were subsequently homogenized in Tris-HCl buffer (0.1 M, pH 7.4). The homogenate was centrifuged at 3000 rpm for 10 min to yield a pellet that was discarded and the supernatant was kept for the bioassay.
Antioxidant activity assays
Total antioxidant activity assay
Total antioxidant activity of the extract was determined through the phosphomolybdenum reduction assay according to the method described by Prieto et al. [Citation22]. Briefly, 0.3 mL of sample was mixed with 3 mL of reagent solution (600 mM H2SO4, 28.0 mM sodium phosphate and 4.0 mM ammonium molybdate). The mixture was incubated at 95°C for 90 min and the absorbance of the green phosphomolybdenum complex was measured at 700 nm. Total antioxidant activity was expressed as mg AAE/g sample.
Metal-ion chelating assay
The in vitro Fe2+ chelating ability of extract was determined according to the method of Puntel et al. [Citation23]. Briefly, 0.9 mL of aqueous 0.5 mM FeSO4 and 0.15 mL of the extracts were incubated for 5 min at room temperature. Then, 78 μL of ethanolic solution of 1,10-phenanthroline was added. The absorbance of the orange color solution was read at 510 nm. The in vitro of Fe2+ chelating ability of the samples was calculated by using the following formula:
ControlAbs = The absorbance of the control (Reaction mixture in the absence of extract)
Test sampleAbs = The absorbance of the test sample (Reaction mixture with the extract).
Enzyme inhibitory assays
Determination of α-amylase (EC 3.2.1.1) inhibitory activity
The α-amylase inhibitory activity of the extract was determined according to the method described by Shai et al. [Citation24], with slight modifications based on the spectrophotometric assay. A volume of 250 μL of the extracts used at different concentrations (25–45 µg/mL) was incubated with 500 μL of porcine pancreatic amylase (2 U/mL) in 100 mmol/l phosphate buffer (pH 6.8) at 37°C for 20 min. Two hundred μL of 1% starch dissolved in 100 mmol/l phosphate buffer (pH 6.8) was then added to the reaction mixtures and incubated at 37°C for 1 h. One milliliter of DNS color reagent was then added and boiled for 10 min. The absorbance of the resulting mixtures was measured at 540 nm and the inhibitory activities were expressed as a percentage of a control sample without inhibitors.
where ControlAbs = The absorbance of the control (Reaction mixture without the extract); Test sampleAbs = The absorbance of the control (Reaction mixture with the extract).
Determination of α-glucosidase (EC 3.2.1.20) inhibitory activity
The α-glucosidase inhibitory activity of the extract was determined according to the method described by Ademiluyi and Oboh [Citation25], with slight modifications. Briefly, 0–100 μL of free phenol/bound phenolic extract was incubated with 100 μL of 1.0 U mL−1 α-glucosidase solution in 100 mmol l−1 phosphate buffer (pH 6.8) at 37°C for 15 min. Thereafter, 50 μL of pNPG solution (5 mmol l−1) in 100 mmol l−1 phosphate buffer (pH 6.8) was added and the mixture was further incubated at 37°C for 20 min. The absorbance of the released p-nitrophenol was measured at 405 nm and the inhibitory activity was expressed as a percentage of a control sample without inhibitor.
Monoamine oxidase (EC 1.4.3.4) inhibitory activity
The inhibitory potential of the extract on MAO activity was carried out according to the method of Green and Haughton [Citation26], with slight modification. In brief, the reaction mixture contained 25 mM phosphate buffer (pH 7), 2.5 mM semicarbazide, 10 mM benzylamine (pH adjusted to 7), brain tissue homogenate and appropriate dilutions of the compounds in a total reaction volume of 2 mL. After 30 min, 1 mL of acetic acid was added and boiled for 3 min in boiling water bath followed by centrifugation. The resultant supernatant (1 mL) was mixed with equal volume of 0.05% of 2,4-DNPH and 2.5 mL of benzene was added after 10 min incubation at room temperature. After separating the benzene layer, it was mixed with equal volume of 0.1 N NaOH. Alkaline layer was decanted and heated at 80°C for 10 min. the orange-yellow color developed was measured at 450 nm.
Where Control = Reaction mixture witout the extact;
Test sample = Reaction mixture with the extract.
Determination of IC50 values
The IC50 values in mg/mL (Concentration of the extract that caused 50% inhibition) of the extracts of T. triangulare were calculated from a regression curve of the percentage (%) inhibitions against various concentrations of the extracts.
Protocol for the characterization of ethylacetate and aqueous extracts of T. triangulare by HPLC with diode-array detection (HPLC-DAD)
High-performance liquid chromatography (HPLC-DAD) was performed with a Shimadzu Prominence Auto Sampler (SIL-20A) HPLC system (Shimadzu, Kyoto, Japan), equipped with Shimadzu LC-20AT reciprocating pumps connected to a DGU 20A5 degasser with a CBM 20A integrator, SPD-M20A diode array detector and LC solution 1.22 SP1 software. The T. triangulare extracts at a concentration of 10 mg/mL were injected by means of a model SIL-20A Shimadzu auto sampler. Separations were carried out using Phenomenex C18 column (4.6 mm x 250 mm x 5 μm particle size). The mobile phase was water with 1% formic acid (v/v) (solvent A) and HPLC grade methanol (solvent B) at a flow rate of 0.6 mL/min and injection volume 50 μL.
The composition gradient was: 5% solvent B reaching 15% at 10 min; 30% solvent B at 25 min, 65% solvent B at 40 min and 98% solvent B at 45 min, followed by 50 min at isocratic elution until 55 min. At 60 min the gradient reached the initial conditions again, following the method described by Duarte et al. [Citation27] with slight modifications. The sample and mobile phase were filtered through 0.45 μm membrane filter (Millipore) and then degassed by ultrasonic bath prior to use. Stock solutions of standards references were prepared in the methanol:water (1:1, v/v) at a concentration range of 0.025–0.400 mg/mL. Quantifications were carried out by integration of the peaks using the external standard method, at 325 nm for chlorogenic acid and caffeic acid; and 366 for quercetin, rutin, apigenin, kaempferol and luteolin.
The chromatography peaks were confirmed by comparing its retention time with those of reference standards and by DAD spectra (200 to 600 nm). Chlorogenic acid (Retention time-tR = 21.75 min, peak 1), caffeic acid (tR = 26.11 min, peak 2), rutin (tR = 34.94 min, peak 3), quercetin (tR = 43.62 min, peak 4), kaempferol (tR = 46.15 min, peak 5), luteolin (tR = 56.93 min, peak 6) and apigenin (tR = 60.78 min, peak 7).
Calibration curves used
Caffeic acid: Y = 12409x + 1435.8 (r = 0.9996); apigenin: Y = 13572x + 1087.3 (r = 0.9995); kaempferol: Y = 11865x + 1172.3 (r = 0.9999); chlorogenic acid: Y = 13756x + 1259.4 (r = 0.9998); quercetin: Y = 10791 + 1285.3 (r = 0.9997); rutin: Y = 10938x + 1186.9 (r = 0.9999) and luteolin: Y = 13157x + 1247.3 (r = 0.9995). All chromatography operations were carried out in triplicate (n = 3) at ambient temperature.
Limit of detection (LOD) and limit of quantification (LOQ)
LOD and LOQ were calculated based on the standard deviation of the responses and the slope using three independent analytical curves, as defined by Oboh et al. [Citation28]. Limit of detection (LOD) and limit of quantification (LOQ) were calculated as 3.3 and 10 σ/S, respectively, where σ is the standard deviation of the response and S is the slope of the calibration curve. LOD and LOQ are terms used to describe the smallest concentration of a measurand that was reliably measured by the procedure used. Whereas, LOQ is the lowest concentration at which the compounds were reliably detected and predefined/bias imprecision were met.
Statistical analysis
All results were analyzed by with one-way ANOVA test (SPSS Version 20) followed by Duncan multiple range test. Differences were considered significant when p < 0.05. The graphical analyses were carried out using GraphPad Prism5 Program (GraphPad Software, San Diego, CA, USA).
Results
In vitro antioxidant assays
shows the antioxidant potentials in the ethylacetate and aqueous extracts of T. triangulare. The results, however, showed that ethylacetate extract had higher phophomolybdenum reducing total antioxidant power (11.52 ± 0.78 mg AAE/g) than aqueous extract (2.46 ± 0.26 mg AAE/g). The values were expressed as mean±S.E of three trials (n = 3).
represents metal chelating potentials of ethylacetate and aqueous extracts of T. triangulare. However, the results indicated that aqueous extract had higher metal chelating potential than the ethylacetate extract in concentration-dependent manner when incubated with Fe2+. The values were expressed as Mean± S.E. of three trials (n = 3).
Enzyme activity inhibitory potentials of ethylacetate and aqueous extracts of T. triangulare
shows the inhibitory potentials of ethylacetate and aqueous extracts of T. triangulare against pancreatic α-Amylase activity. The data showed that there was no significant (p > 0.05) difference in the inhibitory activities of ethylacetate and aqueous extracts in a concentration-dependent manner (0.00–0.25 mg/ml). The values were expressed as Mean± S.E. of duplicate determinations (n = 2).
shows the inhibitory potentials of ethylacetate and aqueous extracts of T. triangulare against intestinal α-glucosidase activity. The results, however, indicated that there was a significant (p < 0.05) difference in the values, with ethylacetate extract showing higher inhibitory values (IC50 = 0.17 ± 0.01 mg/ml) than aqueous extract (0.85 ± 0.01 mg/ml) in a concentration-dependent manner. The values were expressed as Mean± S.E. of duplicate determinations (n = 2).
represents inhibitory potentials of ethylacetate and aqueous extracts of T. triangulare against MAO in the brain tissue. The results showed that ethylacetate extract caused significant inhibition (p < 0.05) than aqueous extract in a concentration-dependent manner (0.00–0.25 mg/ml). The values were expressed as Mean± S.E. of duplicate trials (n = 2).
Characterization of the components of ethylacetate and aqueous extracts of T.triangulare by HPLC-DAD
Discussion
Plant-based polyphenolic compounds have been considered important in moderating the predominance of diabetes mellitus disorder [Citation29]. Currently, there is a growing evidence that plant polyphenols including flavonoids are unique nutraceuticals and supplementary treatments for various DM and its various complications [Citation29,Citation30]. The medicinal activities of plant-derived materials have been attributed to the naturally available polyphenolic constituents, in the same vein, many plants, however, have demonstrated different degrees of inhibitions against some enzymes involved in the pathogenesis of DM as a result of the different endowed polyphenolic constituents [Citation20,Citation31].
Besides, studies have significantly revealed that, the target of most therapeutical oral drugs (also called starch blockers) involved in the management of DM is to control/decrease postprandial hyperglycemia via the inhibition of carbohydrate-hydrolyzing enzymes such amylases and glucosidases in the gastrointestinal tract (GIT) [Citation32–Citation34]. However, some plant-based phytonutrients such as; flavonoids and total phenolic contents (, and ) have been shown to be potential inhibitors of these key carbohydrate-metabolizing enzymes [Citation35], as well as their ability to enhance the re-generation of pancreatic islets and subsequently aiding insulin release hence, causing increase in hepatic glucokinase activity [Citation36,Citation37]. In our work, we found out that, ethylacetate showed a strong α-glucosidase inhibitory activity and aqueous extracts of T. triangulare also demonstrated this same activity against pancreatic α-amylase and intestinal α-glucosidase (, and ), which are two key enzymes in type 2 DM. These inhibitory activities could be attributed to the presence of polyphenolic components (, and ), with earlier reports establishing that antioxidant properties of plant food correlate with the phenolic contents [Citation37].
Table 1. HPLC-DAD compositional analysis of the ethyacetate and aqueous extracts of T. triangulare.
Table 2. The IC50 values (mg/ml).
Figure 1. (a, b, c & d): Some compounds detected from ethylacetate and aqueous extracts of T. triangulare. By HPLC-DAD.
Key: a, Chlorogenic acid; b, caffeic acid; c, quercetin; d, luteolin.
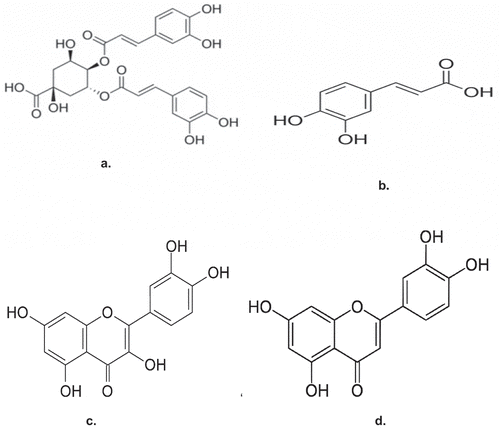
Furthermore, oxidative stress following excessive production of free radicals has been described to play a vital role in the pathophysiology of DM through various mechanisms [Citation24]. However, the importance of antioxidants (polyphenolic compounds) in protecting the human body from the activities of free radicals, chelating agents and activities of oxidases due to redox properties of their hydroxyl groups has been reported [Citation38]. In this study, we realized that both the extracts chelated Fe2+ significantly (), in order word, this could as well be demonstrated in vivo by preventing the catalytic role of Fe2+ in the generation of hydroxyl-radical (●OH) from H2O2 through the Fenton reaction [Citation39].
Figure 4. α-Amylase activity inhibitory potentials of ethylacetate and aqueous extracts of T. triangulare.
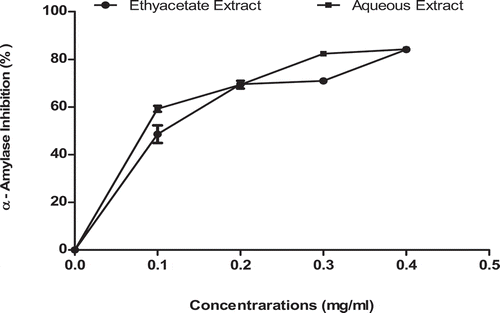
Figure 5. α-Glucosidase activity inhibitory potentials of ethylacetate and aqueous extracts of T. triangulare.
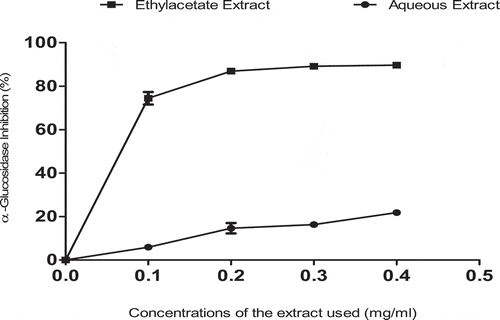
Similarly, DM has unequivocally been connected to contractile dysfunction via ROS-induced mitochondria dysfunction [Citation40,Citation41]. Mitochondria have emerged as the most relevant source of reactive oxygen species [Citation42]. The generation of ROS in the mitochondria has been reported to be via the activities of monoamine oxidases (MAOs) with 2 isoforms (A and B) at the outer membrane [Citation43].
Nevertheless, MAO potential inhibitors have shown an expanded level of catecholaminergic system which is thought to build insulin activity [Citation15]. In our finding, both the extracts demonstrated inhibition on MAO (), ethylacetate extract, however, demonstrated milder inhibition on MAO than the aqueous extract. This could be attributed to its antioxidant potential as shown in . Howbeit, inhibition of MAO has been described to signal reduction the generation of ROS (H2O2) and degeneration of mitochondrial dysfunction in DM.
Figure 6. MAO activity Inhibitory potentials of ethylacetate and aqueous extracts of T. triangulare.
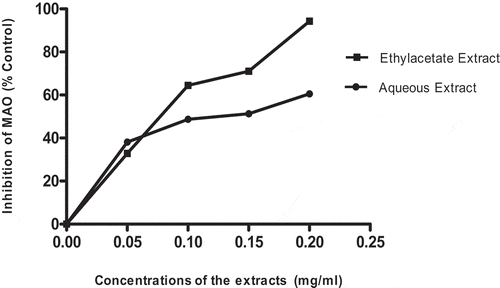
Figure 7. (a&b): Representative of HPLC-DAD profile of ethylacetate (a) and aqueous (b) extracts of T. triangulare. Chromatograms showing the presence of chlorogenic acid (peak 1), caffeic acid (peak 2), rutin (peak 3), quercetin (peak 4), kaempferol (peak 5), luteolin (peak 6) and apigenin (peak 7).
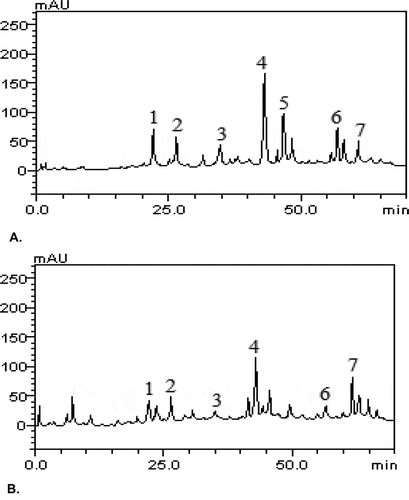
Conclusion
In our study, ethylacetate and aqueous extracts were able to demonstrate inhibition on two key carbohydrate-metabolyzing enzymes and a prominent contributor of oxidative stress in DM in vitro. It could, therefore, be concluded that the two extracts of T. triangulare if properly annexed could be a promising alternative in the management of DM.
Acknowledgments
The authors indeed appreciate the contributions of all the staff members of the Department of Chemical Sciences, Afe Babalola University (ABUAD), Ado-Ekiti, Ekiti State, Nigeria.
Disclosure statement
No potential conflict of interest was reported by the authors.
References
- Shaw J, Chisholm D. Epidemiology and prevention of type II diabetes and the metabolic syndrome. MJA. 2010;179:379–383.
- Celik IE, Yegin FO. Effect of experimental diabetes mellitus on plasma lactate dehydrogenase and glutamic oxaloacetic transaminase levels in rabbits. Turk J Biol. 2002;26:151–154.
- Madhusudhan T, Kirankumar H. In-vitro α-amylase and α-glucosidase inhibitory activity of Adiantum caudatum Linn. and Celosia argentea Linn. extracts and fractions. Indian J Pharmacol. 2015;47(4):425–429.
- World health organization (WHO) consultation. Definition, diagnoses, and classification of diabetes mellitus and its complication. Part 1: diagnosis and classification of diabetes mellitus. Report of a WHO consultation. Geneva; 1999.
- Inga P, Mark IM, Cecilia ML. Type 2 diabetes: new genes, new understanding. Trends Genet. 2008;24(12):613–621.
- Mitra A, Dewanjee D, Dey B. Mechanistic studies of lifestyle interventions in type 2 diabetes. World J Diabetes. 2012;3(12):201–207.
- Sophia D, Manoharan S. Hypolipidemic activities of Ficus racemosa Linn bark in alloxan induced diabetic rats. Afr J Trad CAM. 2007;4(3):279–288.
- Yan LJ. Pathogenesis of chronic hyperglycemia: from reductive stress to oxidative stress. J Diabetes Res. 2014;2014:137919.
- Jakus V. The role of free radicals, oxidative stress and antioxidant systems in diabetic vascular disease. Bratisl Lek Listy. 2000;101(10):541–551.
- Yin Y, Zheng Z, Jiang Z. Effects of lycopene on metabolism of glycolipid in type 2 diabetic rats. Biomed Pharmacother. 2019;109:2070–2077.
- Siddiqui A, Hanson I, Andersen JK. Mao-B elevation decreases parkin’s ability to efficiently clear damaged mitochondria: protective effects of rapamycin. Free Radical Res. 2012;46(8):1011–1018.
- Oana MD, Rodica L, Adrian S, et al. Monoamine oxidases as potential contributors to oxidative stress in diabetes: time for a study in patients undergoing heart surgery. BioMed Res Int. 2015:9.
- Sukhwinder SL, Gurcharan K. Effect of experimental diabetes on monoamine oxidase activity from discrete areas of rat brain: relationship with diabetes associated reproductive failure. Mol Cell Biochem. 1997;177:15–20.
- Nita M, Grzybowski A. The role of the reactive oxygen species and oxidative stress in the pathomechanism of the age-related ocular diseases and other pathologies of the anterior and posterior eye segments in adults. Oxid Med Cell Longev. 2016;2016:2016:3164734.
- Kaludercic N, Carpi A, Nagayama T, et al. Monoamine oxidase B prompts mitochondrial and cardiac dysfunction in pressure overloaded hearts. Antioxid Redox Signal. 2014;20(2):267–280.
- Arumugam G, Manjula P, Paari N. A review: anti diabetic medicinal plants used for diabetes mellitus. J Acute Dis. 2013; 2(3):196–200.
- Afolabi OB, Oloyede OI, Agunbiade SO. Inhibitory potentials of phenolic-rich extracts from Bridelia ferruginea on two key carbohydrate-metabolizing enzymes and Fe2+-induced pancreatic oxidative stress- In vitro. J Integr Med. 2018;16(3):192–198.
- Aja PM, Okaka AN, Onu PN, et al. Phytochemical composition of Talinum triangulare (waterleaf) leaves. Pak J Nutr. 2010;9(6):527–530.
- Sridhar R LG. Lipid classes, fatty acids and tocopherols of leaves of six edible plant species. J Agric Food Chem. 1993;41:61–63.
- Afolabi OB, Oloyede OI. Antioxidant properties of the extracts of Talinum triangulare and its effect on antioxidant enzymes in tissue homogenate of Swiss Albino rat. Toxicol Int. 2014;21:307–313.
- Afolabi OB, Oloyede OI, Olayide II, et al. Antioxidant enhancing ability of different solvents extractable components of Talinum traingulare in some selected tissue homogenates of albino rat-in vitro. J Appl Pharm Sci. 2015;5(9):56–61.
- Prieto P, Pineda M, Aguliar M. Spectrophotometric quantitation of antioxidant capacity through the formation of phosphomolybdenum complex: specific application to the determination of vitamin E. Anal Biochem. 1999;269(2):337–341.
- Puntel RL, Nogueira CW, Rocha JBT. Krebs cycle intermediates modulate thiobarbituric acid reactive species (TBARS) production in Rat Brain In vitro. Neurochem Res. 2005;30:225–235.
- Shai LJ, Masoko P, Mokgotho MP, et al. Yeast alpha glucosidase inhibitory and antioxidant activities of six medicinal plants collected in Phalaborwa, South Africa. South Afr Bot. 2010;76:465–470.
- Ademiluyi A, Oboh G. Soybean phenolic-rich extracts inhibit key-enzymes linked to type 2 diabetes (α-amylase and α -glucosidase) and hypertension (angiotensin I converting enzyme) in vitro. Exp Toxicol Pathol. 2013;65:305–309.
- Green AL, Haughton TMA. Colorimetric method for the estimation of monoamine oxidase. Biochem J. 1961;78:172–176.
- Duarte AE, Waczuk EP, Roversi K, et al. Polyphenolic composition and evaluation of antioxidant activity, osmotic fragility and cytotoxic effects of Raphiodon echinus (Nees & Mart.) Schauer. Molecules. 2016;21(2):1–15.
- Oboh G, Ademosun AO, Akinleye M, et al. Starch composition, glycemic indices, phenolic constituents, and antioxidative and antidiabetic properties of some common tropical fruits. J Ethnic Foods. 2015;2:64–73.
- de Sales PM, Souza PM, Simeoni LB, et al. α-amylase inhibitors: a review of raw material and isolated compounds from plant source. J Pharm Sci. 2012;15:141–183.
- Rana MI, Ali MH, Dalia OS, et al. HPLC-DAD-MS/MS profiling of phenolics from Securigera securidaca flowers and its anti-hyperglycemic and anti-hyperlipidemic activities. Braz J Pharmacogn. 2015;25(2):134–141.
- Karthikesan K, Pari L, Menon VP. Antihyperlipidemic effect of chlorogenic acid and tetrahydrocurcumin in rats subjected to diabetogenic agents. Chem Biol Inter. 2010;188(3):643–650.
- Cheng AYY, Fantus IG. Oral antihyperglycemic therapy for type 2 diabetes Mellitus. Can Med Assoc J. 2005;172:213–226.
- Upadhyay RK, Ahmad S. Management strategies for control of stored grain insect pests in farmer stores and public ware houses. World J Agric Sci. 2011;7(5):527–549.
- Zohre MD, Foroogh N, Mohamad EA. Alpha amylase inhibition activity of some plants extract of Teucrium species. Eur J Biol Sci. 2015;7(1):26–31.
- Kwon YI, Apostolidis E, Kim YC, et al. Health benefits of traditional corn, beans and pumpkin: in vitro studies for hyperglycemia and hypertension management. J Med Food. 2007;10:266–275.
- Andrea L, Judit H, Katalin VS, et al. Epidemiological studies have shown a correlation between an increased consumption of phenolic antioxidants and a reduced risk of cardiovascular disease and certain types of cancer. Acta Biol Szegediensis. 2003;47(1–4):119–125.
- Chu Y, Sun J, Wu X, et al. Antioxidant and antiproliferative activity of common vegetables. J Agric Food Chem. 2002;50:6910–6916.
- Amic D, Davidovic-Amic D, Beslo D, et al. Structure-radical scavenging activity relationship of flavonoids. Croatia Chem Acta. 2003;76:55–61.
- Britton RS, Leicester KL, Bacon BR. Iron toxicity and chelation therapy. Int J Hematol. 2002;76:219–228.
- Flarsheim CE, Grupp IL, Matlib MA. Mitochondrial dysfunction accompanies diastolic dysfunction in diabetic rat heart. Am J Physiol Heart Circulatory Physiol. 1996;271(1):192–202.
- Montaigne D, Marechal X, Coisne A, et al. Myocardial contractile dysfunction is associated with impaired mitochondrial function and dynamics in type 2 diabetic but not in obese patients. Circulation. 2014;130(7):554–564.
- Chen YR, Zweier JL. Cardiac mitochondria and reactive oxygen species generation. Circ Res. 2014;114(3):524–537.
- Kaludercic N, Mialet-Perez J, Paolocci N, et al. Monoamine oxidases as sources of oxidants in the heart. J Mol Cell Cardiol. 2014;73:34–42.