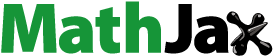
ABSTRACT
From the top-down view, an organism is constituted by an assembly of cells where a network of molecules interacts to create a regulated complex system. Cells are a universe of molecular interactions maintained under non-equilibrium regimes. The complex network of interactions drives life where DNA and epigenetic signals are considered as the memory of a cell. The full understanding of these interactions is the first step toward the design of artificial synthetic pathways controlling a program. In our review, we present some insights into the different methods and designs applicable for the development of synthetic artificial cells. A self-replicative process of these cells, thermodynamic and kinetic features, as well as equilibrium systems of artificial cells, will enable scientists to have an in-depth knowledge of this domain. Furthermore, the cascade of encoded molecular chemical interactions orchestrated by the genetic program DNA, biomolecules, epigenetic signals, and nano-factory modulation will be discussed in terms of their application in the entire set of interactomes within a cell.
Introduction
The interactions between biomolecules, metabolism from the mitochondrial factory, and the cell membrane give birth to cellular life. Cells need a set of information carriers and metabolic reactions to become functional in a changing environment. In the last century, discovery-driven biology as well as hypothesis-driven biology to design and control the new cellular functions and genetic circuits that trigger synthetic biology has been emerging domains [Citation1]. The complex network of interactions that drives life includes key features such as docking, adaptive systems, embedding, exchange, tethering, programs, non-covalent interactions, covalent linkages, and out of equilibrium and equilibrium states, self-replicative systems, self-organized and self-assembled systems, etc. The exploration and application of these interactions have led to the emergence of synthetic biology [Citation2]. The field of synthetic biology is mainly divided into two branches; first by synthetic protocell biology (SPB), where the synthetic units are assembled into chemical systems endued with inheritance, evolution, and reproduction (biological properties) [Citation3]. The second branch deals with the extraction and assembly of biological units from living systems to obtain a modified version of existing biological systems. The last one deals with the generation and rewiring of genetic circuits using elementary building blocks [Citation4]. One of the goals driven by these research branches is to obtain a programmable plug-in genetic device [Citation5], either by directed evolution techniques [Citation6] or by rational design [Citation7]. Chang (1957) was the first to propose the concept of artificial cells [Citation8]. The studies of primordial cells [Citation9] co-translational insertion of membrane proteins into liposomes [Citation10] and delivery of drugs [Citation11] are a few examples of applications made by the use of artificial cells in biotechnology and industrial field.
Artificial cells can be defined as simple, small entities made up of a few properties of natural cells [Citation12]. Certain phenotypes and functions of natural cells are mimicked by well defined in vitro artificial systems. The various types of created artificial cells are protocells [Citation13], where artificial cells were constructed using synthetic membranes and cellular components [Citation14] and natural cell extracts such as essential genes and genetic circuits useful for their maintenance [Citation15]. Artificial cells can be used to explore the dynamics of cell cycle regulation and to study the proliferation with minimal intervention. Second, to investigate the properties of biological cells, followed by the investigation of new applications and the expansion of biological regulations and mechanisms found in nature [Citation16]. The essential properties and related structures of living cells should ideally be presented in typical artificial cells but can be expanded to more complexity [Citation17]. A newly constructed artificial cell should possess three elementary features: a memory (DNA or RNA), a stable semi-permeable membrane, a series of metabolic pathways, and a complex epigenetic signal [Citation18].
In this mini-review, different methods involved in the construction of artificial cells are described, followed by the current research progress in synthetic biology. The second part discusses the design and assembly of synthetic and semi-synthetic constructs and secondary metabolites. The interaction leading to the biological responses is described in the third part. A few examples related to synthetic self-replicative programs and proteins are discussed in the fourth part. Finally, thermodynamic, out of equilibrium, and out of equilibrium systems that can be envisaged for artificial cells are also discussed in this review.
Construction of artificial cells
Artificial cells are made up of three parts, a shell (cellular compartment), an engine (transcription and translation machinery) and finally the information (genetic components). The constructed circuits in cell-free systems are tested in a second step to make sure that the synthetic circuits and machinery are active inside the designed artificial cells. In the final step, the cell-free systems are encapsulated inside the membrane made up of phospholipids or fatty acids [Citation12]. The different methods by which shell can be constructed are extrusion methods, lyophilization methods, water-in-oil methods and microfluidic devices. Artificial cells with uniform size, multi-lamellar membranes and controlled cellular diameter were generated by extrusion processes. Large artificial cells with heterogeneous size and lamellarity were prepared by lyophilization methods. Large unilamellar artificial cells with heterogenous size artificial cells were produced by water-in-oil methods. Artificial cells with a controllable size by adjusting the diameter of the channels and the flow speed were generated with microfluidic devices [Citation12]. Two different methods were used in the construction of artificial cells namely: Top-down approach and bottom-up approach.
Top -down approach for artificial cells
The top-down construction of ‘minimal cells’ is carried out by decreasing the genome of living cells. A primitive living organism does not require a high number of genes to be alive. Venter and colleagues discovered 517 genes in the parasitic bacterium Mycoplasma genitalium in 1995 [Citation19]. An artificial infectious poliovirus was created by a top-bottom approach in 2002. With this method, the full-length poliovirus DNA (cDNA) is synthesized de novo and later transcribed into highly infectious viral RNA with the help of T7 RNA polymerase. Then, the transcription and replication of viral RNA took place in the cytoplasmic extract of uninfected cells, producing poliovirus with identical physiological and pathological properties compared to natural virus [Citation20]. In 2004, minimal-gene sets were redefined for cell viability by Gil et al. [Citation21]. Recently, a computer-based genome sequencing named Mycoplasma mycoides JCVI-syn1.0 was designed by Venter et al. on two strains of M. mycoides subspecies capri GM12 [Citation22]. The expected phenotypic properties of M. mycoides, which can self-replicate, were seen in the resulting new cells. These types of cells are called ‘synthetic cells’ The developed synthetic DNA was integrated and accepted by the newly designed semi-synthetic artificial cell. Although the construction of large DNA sequences was enabled by synthetic biology, the creation of more complex artificial life still requires a long process to manipulate, modify, and develop them.
Bottom-up approach for artificial cells
A bottom-up approach is to build a stack of non-biotic components which are assembled to create de novo an artificial cell with a phospholipid bilayer and self-replicating DNA via a genetic program [Citation18]. Typically, the approach involves three basic elements, which include information-carrying molecules (DNA or RNA), metabolic systems, and cell membranes. Two membrane proteins, sn-glycerol-3-phosphate acyltransferase (GPAT) and lyo-phosphatidic acid transferase (LPAAT), are produced by protein synthesis using recombinant element systems (PURE) enclosed in liposomes [Citation23].
The synthesis of living cells from inanimate components is a challenging task [Citation24]. The main focus of this type of target involves intelligent re-configuration of living organisms by top-down approaches [Citation25]. The synthesis of life is now a realistic goal with the recent progress seen in the creation of synthetic cells like simple photocells and artificial cells. Here, we discuss the bottom-up approaches to the construction of artificial cells from molecular subsystems or components [Citation24].
An artificial cell should have the ability to self-replicate, get involved in a learning process, and keep homeostatic data (LYFE definition). In earlier concepts, von Neumann theory provides useful guidelines to build logically a self-replicating compartment [Citation25]. However, to capture the entire spirit of cellular life, there is a need to consider many other nongenetic processes, e.g. molecular self-organization/crowding, artificial environment for nutrient exchange etc. Although the bottom-up approach motivates and also addresses the fundamental question of non-genetic processes, selective exchanges through phospholipid bilayer, osmotic pressure, transcription and translation processes are still challenging issues towards the development of workable microscopic vesicles. Recently, in a top-down approach [Citation23], the genome of a living cell was knocked down to a minimum level to demonstrate that bacteria can be reprogrammed with synthetic genomes. Both bottom-up and top-down approaches towards the synthesis of artificial cells require the development of original methods and ideas [Citation26]. Noireaux et al. demonstrated the states and the development of artificial cell synthesis with experimental constraints. Based on the basic idea of the development of a phospholipid-containing compartment encapsulating synthetic DNA, the expression of genes was carried in vitro upon the exchange of adequate nutrients. Moreover, cell-free transcription and translation permitted the expression of many genes. Nevertheless, the development and integration of synthetic DNA is still challenging for the synthesis of self-reproducing automatons and bacterial genomes [Citation9] ().
Figure 1. Von neumann self-reproducing automaton and a bacterium[Citation9].
![Figure 1. Von neumann self-reproducing automaton and a bacterium[Citation9].](/cms/asset/3276f5c0-b6a7-4707-97e0-b1cfbb51df4c/teba_a_2104071_f0001_b.gif)
The integration of artificial cells with natural cells is also an important aspect in this area. Mansey and co-workers developed a system by integrating artificial with natural cells to evolve E. coli by translating a chemical message of a molecule (theophylline) to activate a specific cellular response. The method promotes better cellular organization and modification without affecting the organism genetic content [Citation9,Citation27,Citation28]. The artificial cells act as chemical translators, which allowed E. coli to sense molecules and induce various mechanisms and functions [Citation29] ().
Figure 2. Artificial cells translate chemical signals for e. coli[Citation27] .
![Figure 2. Artificial cells translate chemical signals for e. coli[Citation27] .](/cms/asset/883f1de2-0d92-48c1-b083-c6e38dd0e63f/teba_a_2104071_f0002_oc.jpg)
Mann group established different models of protocell assemblies [Citation30,Citation31]. Recently, they reported the predatory behavior of artificial cell communities [Citation32]. Previously, a large focus has been given to the integration and networking between components within a vesicle. Mann and his colleagues, on the other hand, concentrated on creating protocell communities and studying their collective response. A predatory behavior of protease containing coacervated microdroplets towards proteinosomers (protein-polymer microcapsures) was observed. The protease microdroplets as predators obliterate the prey (proteinosomers) via protease-induced lysis. The proteinosome payload includes dextran, single-strand DNA, and nanoparticles that are trafficked on predator microdroplets to release compositionally modified killer protocells (). The binary population of predators (positively charged) and prey (negatively charged) with predatory behavior demonstrated the possible use of synthetic protocell communities with compartmentalized colloidal objects as interacting artificial systems. Recently, new dual properties of membrane vesicles as promoters and regulators for many chemical reactions have been reported. The reaction or process involved a decarboxylative reaction, a selective binding to DNA or RNA, and the reactivation of fragmented enzymes to regulate enzyme functions [Citation33]. Szostak group studied the RNA exchanges between aqueous two-phase systems (ATPSs) and coacervate droplets. ATPSc and complex coacervates are defined as phase separation phenomena that result in the selective analysis of biomolecules. Cellular ATPSc composed of dextran and PEG has been commonly used for the partition of whole bacterial cells, cellular organelles and macromolecules. Coacervate droplets containing simple components like mononucleotides and short-chain polypeptides also aid in the selective transport of cellular molecules [Citation34,Citation35]. The exchange of RNA was studied via fluorescence recovery after photobleaching (FRAP) between dextran/polyethene glycol ATPS and ATP/poly-L-lysine coacervate droplets. Efforts towards simple protocells to complex artificial cells are going to help with the understanding of natural living systems [Citation24].
Therefore, the minimal representations of synthetic cellularity using biological processes generated by bottom-up strategies are generating a growing interest in the field of synthetic biology. The use and development of in vitro gene expression systems (IVGES) is considered an advance in photocell engineering to provide off-line biological content for storage and processing in synthetic cell-free environments. Tang group used carboxymethyl-dextran/polylysine (CM-dextran/PLys) coacervate for the sequestration and retention of a plasmid-containing IVGES to show cell-free gene expression and folding of the red fluorescent protein mCherry at pH = 8 [Citation30]. Another study on engineered cells in mammalian tissues is linked to a synthetic module (photoactivated synthesis of cyclic dimeric GMP) to obtain a 40-fold photoactivation of gene expression [Citation36] (). Recently, it was found that the oxygenation of stem cells is stimulated by artificial membrane-binding proteins during the engineering of large cartilage tissues [Citation37]. It was reported in 1997 that the vesicle growth was driven by the simple dipeptide catalyst seryl-histidine (Ser-His) through the catalytic synthesis of a hydrophobic dipeptide, N-acteyl-L-phenylalanine-leucinamide (AcPheLeuNH2) [Citation38].
Figure 3. Near infrared window (NIRW) light-activated c-di-GMP module regulates the diverse biological activities. The various biological activities are regulated by linking the NIRW light-controlled synthetic c-di-GMP module to the output through c-di-GMP receptor proteins and RNA.
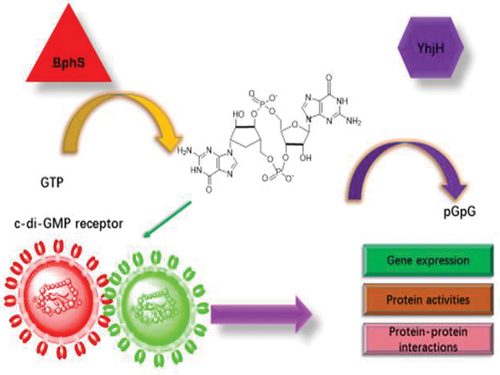
The polymerization rate and fidelity in the copying of a 3’-NP-DNA (N3’-P5’-linked phosphoramidate) template into a complementary strand of 3’-NP-DNA are increased by 2-thiothymidine modification. It is indicated that 3’-NP-DNA can be used as a genetic material in artificial biological systems [Citation39]. Jia and coworkers explained that fatty acid membranes are protected from the disruptive effects of high Mg2+ by introducing a screen made up of citrate during the process of copying RNA inside the model of protocells. The high rate of RNA exchanges between different stages in dextran/polyethylene glycol ATPS and ATP/poly-L-lysine coacervate droplets is confirmed by fluorescence recovery with the use of photobleaching (FRAP) microscopy. Aqueous two-phase systems (ATPSs) and complex coacervates were shown to be inefficient for biological processes and RNA evolution [Citation40]. Recently, the use of microfluidics in the bottom-up construction of artificial cells has resulted in membrane-encapsulated artificial cells with uniform size distribution, cellular dimensions, defined internal content, and controlled biomolecular membrane composition [Citation41]. Several inherent advantages are seen in building cells through a bottom-up approach. The design, control, and study of artificial cells are made easier by reducing the complexity of artificial cells. The intermediates and molecules toxic to biological cells can be simulated by a bottom-up approach. Another advantage of the bottom-up approach is the incorporation of non-biological building blocks that would interfere with the cellular processes [Citation40].
Current research status on synthetic artificial cells
In 1957, Chang at McGill University developed the first artificial cell made up of ultrathin membranes of nylon, cross-linked protein or collodin [Citation42]. This was made possible by one area of post-genomics research called synthetic biology, a method used for proposing and creating unique forms for human practice [Citation43]. Previously, artificial cells were produced using micro-sized cells such as enzymes, proteins, and hemoglobin. However, later cells with dimensions ranging from a hundred micrometers to nanometers, such as vaccines, drugs, and peptides, are used for the same purpose [Citation44]. Synthetic biology is likely to influence contemporary human life [Citation45] and is also a highly multi-disciplinary field that forces science to approach and decipher problems that cannot be readily encountered in the course of single analysis, motivating the design of new solutions [Citation46]. The need for an efficient plan to construct new biological systems, to remodel some natural biological systems and the adaptability of new designed biological systems to resolve new challenges faced by human kind provides the basis for studies in synthetic biology [Citation47]. The four blueprint functions in synthetic biology are parts, pathways, genomes, and systems [Citation48]. In 2011, the Bill and Melinda Gates Foundation announced the grant application ‘Apply Synthetic Biology to Global Health Challenges’ under its ‘Grand Challenges ExpA novelorations’ (GCE) program in order to enhance the emerging tools of synthetic biology for the goals of global health. A solution was delivered to global health with the creation of vaccines and drugs, a new diagnostic method at low manufacturing cost and improved production efficiency by the synthetic biology approach. A novel and major innovation towards a new global health solution is made possible by synthetic biology. Many projects are proposed to find a cure for many specific infectious pathogens threatening world health in the form of synthetic peptides and artificial transcription factors [Citation46]. Recently, a novel artificial ion channel molecule involving a mimic of dansylated polytheonamides was synthesized and the functional analysis was analyzed to create a new platform for the development of different cytotoxic molecular-based channels possessing effective desired functions [Citation49].
In China, the first project on synthetic biology funded by the Sixth Framework program of the European Union was launched in 2006 and is a part of the Programmable Bacteria Catalyzing Research (PROBACTYS) project [Citation50]. Later, a study on the governance of synthetic biology in China was conducted in 2010 and projected synthetic biology to be more dynamic with a new scientific framework [Citation51]. To date, many in silico, in vitro, and in vivo studies have been proposed, sanctioned, and successfully completed in synthetic biology. Some of his previous works are included: 1) IN SILICO: Many databases and software tools related to synthetic biology have been created, namely the synthetic biology software suite (SynBioSS) (synthetic genetic constructs), GenoCAD (synthetic DNA sequences), Cytoscape (analyses of complex networks), Funahashi cellDesigner (used to model network dynamics and also for the analysis of iron metabolism and RB/E2F pathway) [Citation52]. 2) IN VITRO: Computer-aided design of DNA synthesis, development of biological parts (synthetic RNA regulatory elements, synthetic enzymes, proteins) and synthetic biological circuits (regulation of transcription, RNA-based regulation and metabolism, metabolite-controlled intercellular signaling method) [Citation52]. The CD19-specific antigen presenting cells (APCs) (NIH3T3-CD19/86, NIH3T3-CD19/86/137 L) were prepared artificially to amplify functional CD19-CART cells and to obtain clinical scale high quality CD19-CART cells [Citation53]. 3) IN VIVO: Large scale production capacity for a biological network is made possible by in vivo synthetic biology. In vivo synthetic biology [Citation52]: development of the Bacillus subtilis genome, assembly of recombinant DNA of Saccharomyces cerevisiae, and endogenous carotenoid pathways in higher plants are some of the tasks carried out by in vivo synthetic biology [Citation53]. Previous researchers have also explored the role played by synthetic biology on biosecurity by challenging the myths [Citation54], by designing of directed molecular networks [Citation55], artificial bimolecular machines [Citation56] and artificial photosynthesis for plants growth [Citation57].
Design and assembly of artificial cells
Generally, artificial cells are made of three parts, namely cellular compartments, transcription and translation machinery, and genetic components. The three steps involved in the construction of artificial cells are: (1) use of synthetic modules to construct genetic circuits in vivo, (2) testing the constructed circuits in vivo, and (3) providing the transcription and translation engines in cell-free systems. The testing and optimization of newly-constructed genetic circuits illustrated the feedback loop between (1) and (2). The cell-free systems and the circuits are enclosed inside the synthetic artificial cells. The first step involved in the construction of artificial cells is the design and testing of genetic circuits such as logic gates, promoters, and different transcription factors. All the processes were conducted in cycles between in vivo and in vitro systems. The constructed circuits were tested in cell-free systems in the second step. The constructed circuits were tested to see whether their functions were affected by artificial chemical environments. Finally, the third step involved the encapsulation of the cell-free systems inside the membranes. The two major types of cell-free systems in use are whole cell extracts and protein synthesis using recombinant elements (PURE) systems. The prokaryotic or eukaryotic cytosols were used for the extraction of the whole cell by removing the natural cell walls. The pure system was built based on purified components from E. coli and the concentration of each component. A few examples of artificial cells involving viruses and their uses are described. A robust method (in vivo) is carried out to demonstrate the use of 60 bp synthetic recombination sequences to assemble multi-fragment expression vectors in Saccharomyces cerevisiae. This method is useful for the construction of high-throughput strains and complex pathways. The integration of the assembled constructs is facilitated by the introduction of a double strand break by meganuclease I-SceI. The use of meganuclease I-SceI increases the efficiency and integration of the same construct by 95% [Citation58]. Wang et al. generated a synthetic 170 bp dsDNA containing numerous specific neuraminidase inhibitor (NAI) resistance mutations as a positive control in downstream assays using synthetic paired-long oligonucleotides. The diagnosis of resistance mutations, molecular diagnosis, and fast molecular testing with respect to influenza virus drug resistance are the significant applications of the aforementioned advancement [Citation59].
Munshaw group work on a fully rational synthetic HCV subtype 1a virus (Bole1a) was designed by Bayesian phylogenetics, covariance analysis, and ancestral sequence reconstruction comprising mainly of 338 epitopes and envelope genes assembled and mediated in the entry into the target cells [Citation60]. The intracellular incorporation of synthetic enhancers can help in the generation of viruses with novel properties and is also a good example cited in the construction of artificial cells [Citation61]. Cottingham and his coworkers’ study is based on the clinically deployable viral vector by proof-of-concept experiments, modified Vaccinia virus Ankara bacterial artificial chromosomes (MVA-BCA), and it is found to be an effective new candidate for mutant and recombinant vaccines [Citation62]. The construction of synthetic CMV promoters of all strengths was carried out by a 10-mer synthetic enhancer spacer [Citation63]. A new approach for the treatment of DENV infection in humans is based on improving the nucleic acid inhibitors of the Dengue virus (DENV) by RNAi (RNA interference) based on multiple artificial microRNAs (amiRNAs) that target the conserved regions of the virus [Citation64]. The few studies on artificial cells mimicking bacteria report the construction of Artesimisin artesunate (ARS), a synthetic tetraxane drug candidate (RKA182) and a trioxolane equivalent (FBEG100), which have been used as an efficient drug for the treatment of P. falciparum malaria [Citation65]. Kissner and his group produced a synthetic dimeric BB-loop mimetic of MyD88 (EM-163) that inhibited pro-inflammatory signaling and was found to be toxic to Staphylococcal enterotoxin B therapeutically [Citation66].
The concept of coevolution was used to produce a series of 74 novel compounds which were produced with target proteins in an intracellular primary survival assay in baker yeast [Citation67]. Subsequently, the expressions of downstream genes were carried out to autocleave hammerhead ribozyme (HHR) to produce a ribosome-binding site [Citation68]. Here, studies on artificial cells involving RNA are described. An efficient system for antiviral drug development is designed using small artificial microRNAs (AmiRs) combined with pRNA-folate conjugates [Citation69]. The design of amiRNA (artificial micro RNA) by incorporating it into the miR168a gene as the backbone through one-step inverse PCR amplification; then plant amiRNA expression vectors are constructed and expressed in Nicotiana benthamiana by agro infiltration [Citation70]. Furthermore, in vitro transcription and RNA degradation experiments show that an artificial biochemical oscillator can be used to operate DNA-based nanomechanical devices and functional RNA molecule production [Citation71]. Experiments carried out using artificially auto-inhibited proteases have shown that protein-based molecular switches were constructed using structure-guided design and directed protein evolution [Citation72]. Many sophisticated genetic manipulations like point mutagenesis, scar-less gene substitution and deletion are facilitated by a DNA assembler synthetic biology tool [Citation73]. In addition, the cellular systems are studied by using nanoscale field effect transistors (nanoFETs) as probes [Citation74]. High affinity antigen-receptors expressed by the synthetic biology-based engineering of T-lymphocytes to prevail over immune tolerance is an equally important study [Citation75]. The Tanaka group used an artificial antigen-presenting cell, HLA-DP4-restriction, anti-surviving Th1 and Th2 cytokine responses were induced in cancer patients [Citation76]. For the purpose of generating a synthetic ECG (electrocardiogram), a Gaussian wave-based dynamical model is used, and the noise in the recordings is subsequently filtered out [Citation77]. When the synthetic phage, a genetically engineered M13 bateriophage, displayed a high density of cell-signaling peptides on the major coat proteins, it resulted in tissue regeneration [Citation78]. In fact, the cell proliferation and osteoblastic differentiation of human mesenchymal stem cells (MSCs) are found to have been promoted by synthetic peptides [Citation79]. Another key role played by the artificial receptors, synthetically endowing donor cells, and multifunctional nanomaterials resulted in the enhancement of the function of therapeutic living cells [Citation80]. Garriga-Canuta group studied the expression of mutant huntington (HTT) gene in the brain of R6/2 mice (in vivo) to be repressed in a dose dependent manner by synthetic zinc finger repressors [Citation81]. In the case of the synthetic symmetrization method, is used for the crystallization and determination of the structure of CelA from Thermotoga maritime [Citation82]. In addition, to the synthesis of fused tricyclic pyrido[1,2-α]imidazolium ring systems by single step synthesis method. The synthesis of ningeglanine hydrobromide in large quantity is also performed by the same method [Citation83]. On the positive side, an imidazolidinone-based compound is found to act as a catalyst and also as an autocatalyst in synthetic structure [Citation84]. Finally, the total synthesis of galmic, a neuropeptide using triacid platform 1 and the oxazole precursors synthesis is described to be known for its effectiveness and affinity at galanin receptors [Citation85] ().
Semi synthetic minimal cells
The artificial vesicle-based systems defined as living and made up of a minimal number of proteins, genes, and biomolecules are termed semi-synthetic minimal cells. The cell-free kits inside liposomes are entrapped, resulting in the formation of semi-synthetic minimal cells. One good example of semi-synthetic minimal cells is the use of resistant forms of the P. falciparum parasite by semi-synthetic artemisinin based therapy, which due to its high efficacy is recommended as a treatment for P. falciparum malaria [Citation86]. It is reported to induce neurotoxicity and embryotoxicity in a number of species [Citation87]. Also, the semi-synthetic minimal cells are used as a tool for biochemical-based information and communication technologies (ICTs) in medical sciences [Citation88].
Secondary metabolites
Secondary metabolites are compounds that play a crucial role in the antioxidant response of an organism in counteracting oxidative effects. The types of secondary metabolites include alkaloids, terpenoids, steroids, glycosides, natural phenols, phenoazines, biphenyls, dibenzofurans, beta-lactams, to name just a few examples [Citation89]. The process used in the systematic study of secondary metabolites is referred to as metabolomics. The objective of metabolomics techniques is to discover and characterize secondary metabolites in their metabolite state in natural and engineered bio-systems. The production of cryptic metabolites is fixed in the newly sequenced genomes in the modular biosynthesis apparatus found in nature, resulting in the production of novel chemistry and new strategies towards ambitious re-engineering. Consequently, these are the approaches used in the field of synthetic biology for the production of a secondary metabolite [Citation90]. By all means, within a living cell, the products of biochemical reactions (metabolites) determine the relationships among different pathways [Citation91]. The molecular mechanisms of development, reaction, and resistance to therapeutics in disease research are broadly carried out with the help of personal omic profiling as it is easy to control, low cost, and infer in both human and animal subjects [Citation92]. The single cell analysis resulted in a detailed understanding of cell function. It helps to identify the differences between cells among the cellular populace. In individual bacterial cells, metabolites are detected by Raman microspectroscopy (RMS), secondary ion mass spectrometry (SIMS), and Fourier transform infrared spectroscopy (FTIRS) [Citation93]. Using capillary electrophoresis-electrospray ionization-mass spectrometry (CE-ESI-MS), 300 distinct signals are detected in individual animal neurons [Citation94]. The potential applications and advantages for cell testing are in the metabolic analysis of cell cultures [Citation95]. In particular, the production of antibodies is carried out by cell culture optimization methods, cell transfections, apoptosis determination, novel metabolic pathway identification, lung cancer cell phenotype comparison, and drug testing are the main examples of metabolomics [Citation96]. To point out, some of the major challenges faced in cell metabolomics are the unknown facts about the physical and chemical properties of metabolites, extraction techniques, metabolic pathways, and inaccessible reference libraries of standard compounds [Citation97].
Mimetism of interactions coupled to a biological response
Artificial cells are able to mimic protein–protein interactions because these cells can be engineered as exact copies of protein fragments. The sites of protein–protein interactions seem to be conserved, clustered, and large compared to the binding sites of smaller molecules. The evolutionary conserved residues within interfaces appear to be unrelated to binding but have important implications for identifying protein–protein binding sites as well as being extremely useful for docking studies [Citation97]. The interaction is classified as obligate, oligomeric, tight (permanent), relatively weak (transient), and unstructured segments of mediated proteins [Citation98]. Cancer cells modify the protein-protein interactions taking place in apoptosis and escape potential molecular targets for anti-cancer drug development, which were identified by applying nonlinear stochastic modeling [Citation99]. In addition, the metabolic regulation and the broad annotated protein–protein interaction network of signal transduction in yeast are carried out by reconstruction [Citation100]. Eventually, the chemical and spatial patterns among protein–protein interfaces are studied using PCalign [Citation101]. The study of the predictive interactions among the specific biological pathways of the protein is done by protein–protein functional linkage prediction methods, namely gene neighborhood, expression similarity, phylogenetic profiling, a mirror tree variant, and orthologous gene co-presence in the same gene clusters [Citation102]. Muley group revealed that the purified human L1 ORF1p trimer played an important role in retrotransposition both as a nucleic acid binding protein and also as a nucleic acid chaperone [Citation103].
Synthetic self-replicative program
From the early Sanger work, efforts have been made to study the genetic information of various collections of species over the past 25 years. Studies of the biological roles played by all the genes present in a single cellular system are limited. Another team led by Craig Venter in 2010 was the first to transfect a synthetic genome and study the complete genetic sequence of a self-replicating bacterium, Mycoplasma mycoides, giving a new dimension to scientists working on synthetic self-replicative programs [Citation22]. A lot of effort has been put into the development of enzyme-free DNA replication for the development of a synthetic self-replicative program. The rate of polymerization and fidelity is increased by the replication process with a 3’-NP-DNA (for N3′P5′-linked phosphoramidate DNA) template and a complementary strand of 3’-NP-DNA by the modification of 2-thiothymidine. Thus, it is concluded that 3’-NP-DNA can be used as an efficient genetic material for artificial biological systems [Citation39]. The template-directed polymerization of activated 3’-amino-2’, 3’-dideoxyribonucleotides is used successfully for the synthesis of N3’-P5’-linked phosphoramidate DNA (3’-NP-DNA) [Citation104]. The next work involved the polymerization of TNA oligomers of at least 80 nucleotides in length by terminator DNA polymerase. It is found to be a competent DNA-dependent TNA polymerase with high fidelity [Citation105]. Chen et al. (2009) studied the non-enzymatic, template-directed ligation of 3’-imidazole-activated-2’-amino GNA (glycerol nucleic acid) dinucleotides used for the assembly of GNA oligonucleotides containing N2’-P3’ phosphoramidate linkages (np-GNA). It is also found that npGNA is capable of forming duplexes with itself and with GNA. It is inferred that, based on phosphoramidate linkages, npGNA is a latent self-replication system [Citation106]. Another study based on a cycloaddition reaction between a glycal and an azodicarboxylate followed by direct nucleosidation of the cycloadduct, resulted in the development of a novel method for the synthesis of 2’-amino modified TNA nucleosides (2’-NH2-TNA) was developed. The template of 2’-NH2-TNA was discovered to have poor kinetics and thus cannot be used as a genetic material by biological systems [Citation107].
Self replicative proteins
Mycoplasma mycoides JCVI-syn1.0 is the first synthetic organism to be designed and synthesized with the control of a synthetic chromosome. The newly synthesized cell exhibited competent self-replication and similar phenotypic properties [Citation22]. Another example of self-replicative protein is the amphipathic cyclic D,L–peptides, which exhibit systemic antibacterial activity, are capable of systemic administration, and have been used for the treatment of antibiotic-resistant infections [Citation108]. The first designed macrocyclic peptoid-containing histone deacetylase (HDAC) inhibited Class I histone deacetylases [Citation101]. A novel class of natural and synthetic HDACs lacking Zn2+-binding groups is designed to avoid interactions of off-targets with other metalloenzymes. This inhibitor interacts at the opening of the active site and not with the Zn2+-containing active site, which makes it useful for drug design [Citation109]. A new concise solid support-based synthetic method for the preparation of cyclic D,L -peptides with 1,4,5,8-napthalenetetracarboxylic diimide (NDI) side chains is described in order to provide insight into the basic blueprint for a series of self-assembling cyclic D,L -peptide nanotubes with remarkable optical and electronic properties [Citation110]. The last study describes the functional rotaxane yield by threading the -hemolysin transmembrane pore in a desirable orientation by DNA-PEG hybrid strands [Citation111]. The thermodynamic and kinetic properties for the design of artificial biomolecules such as foldamers and cells will be discussed in the following sections.
Thermodynamic, kinetic and out of equilibrium system dynamics applicable to artificial cells
The biological function of proteins is determined by their specific spatial conformation. The conformation is stabilized by different non-covalent interactions which can be classified into three main categories: electrostatic forces, van der Waals forces and hydrophobic forces [Citation112]. The structure of a protein can be subdivided in structural domains that are self-organizing and often fold independently from the rest of the protein [Citation113]. The thermodynamic depiction of the resulting assemblies is considered while studying the difference between the equilibrium self-assembly (ESA) and dynamic self-assembly (DySA) [Citation113]. These domains are in general not unique to one protein but occur in a variety of different proteins. Even on the scale of structural domains the number of possible conformations is exponentially large and just picking the interaction parameters at random will not give rise to anything that reproduces their biological function. Real biological systems must operate in special regions of parameter space. Anfinsen postulated that the native structure of a protein is a thermodynamically stable structure and it depends only on the primary structure and on the conditions of solution, and not on the kinetic folding route. The Anfinsen principle implies that there are two dominant interactions and that evolution can act to change an amino acid sequence, but the folding equilibrium and kinetics of a given sequence are then matters of physical chemistry. Indeed there is a dominant component to the folding code that is the hydrophobic interaction, that the folding code is distributed both locally and non-locally in the sequence, and that a proteins secondary structure is as much a consequence of the tertiary structure as a cause of it [Citation112].
Recent studies corroborate the fact that large-scale interactions are generally more important than strong intra protein interactions in determining properties and functions of large, complex systems [Citation114]. Given that the secondary structure of helices, strands, and loops is known, there is a tendency toward hydro neutrality, however, proteins often separate into distinct hydroph(ob/il)ic (inside/outside) structural domains. Hydration water in the proximity of protein surfaces plays an essential role in the structure, stability, and dynamics of proteins [Citation115]. Experimental studies show that a short-range and long-range solute-solvent interaction and a heterogeneous hydration dynamics toward functional sites, the so-called hydration funnel appear to be a fundamental element [Citation116]. The changes in collective enzyme-substrate-water coupled motions persist well beyond steady state for both substrates while displaying substrate-specific behaviors. The long lasting changes in the protein-water dynamics reflect a collection of local energetic equilibrium states specifically formed during substrate conversion. The observed long lasting water dynamics contribute to the net enzyme reactivity, impacting substrate binding, positional catalysis and product release.
An important observed phase-space restriction for the folded protein is the enthalpy-entropy compensation effect [Citation117]. The enthalpy-entropy compensation effect refers to the behavior of a series of closely related conformations, which exhibit a linear relationship between the thermodynamic parameters describing the conformal transformation. In mathematical terms enthalpy(H)-entropy(S) compensation can be described by:
in which α and β are the linear scaling constants. The linear scaling law leads to the equation for the Gibbs free energy (G) given by:
in which β is the iso-kinetic temperature. At the iso-kinetic temperature the change in Gibbs free energy is equal to the change in enthalpy i.e. there is no energy loss in the system and thus has the system the highest possible thermodynamic efficiency. Indeed conformational changes in physiological function are usually no greater than 0.5 A and closely scale to linear thermodynamic changes. The major evolutionary achievement in making proteins big is their crystal like phase behavior. That makes entropy as important as enthalpy so the scalar quantities of small-molecule chemistry can be replaced by the vector quantities that appear necessary to make biology possible [Citation118].
An important region in the phase space of an ensemble of molecules is at or near the critical temperature (Tc). At the critical point, phase boundaries vanish and the phases merge together into a single phase. It is important that the critical temperature of artificial catalytic molecules (proteins) is below the ambient temperature of the cell. In this context extremophiles form an interesting group of organisms. In the presence of two length scales, i.e. long scale hydrophobic interactions and short scale primary structure interactions, self-organized criticality can occur and the main interesting phenomenon at the critical point is self-organized criticality. In fact one of the mechanisms by which complex systems arise in nature is self-organized criticality (SOC) which is typically observed in non-linear systems with a large degree of freedom. SOC is frequently observed in cells, many base pairs make up a DNA strand and SOC can consolidate distinct states of gene expression [Citation119]. Many nerve cells make up a neural network and SOC is believed to be a fundamental property of neural systems [Citation120]. Many amino acids make up a single protein and proteins appear to be the most dramatic natural example of self-organized criticality [Citation115,Citation121]. SOC can consolidate the structure and hence the catalytic function of the protein. A fundamental question is whether all biological systems are poised at SOC [Citation122]. The physical description of systems that are in a self-organized critical state lay in the fields of statistical physics and non-equilibrium thermodynamics. Non-equilibrium thermodynamics predicts that there is a general tendency in driven many-particle systems towards self-organization into states formed through exceptionally reliable absorption and dissipation of work energy from the surrounding environment that is a minimum level of dissipation corroborating the enthalpy(H)-entropy(S) compensation effect [Citation123].
While the energetics and dynamics of folding in natural proteins is well studied, little is known about the forces that govern folding in modified backbones which determines the secondary structure of the protein. The thermodynamic consequences of backbone alteration on protein folding, focusing on two types of chemical changes made in different structural contexts of a compact tertiary fold can be studied employing foldamers. Foldamers are sequence-specific oligomers similar to peptides, proteins and oligonucleotides that fold into well-defined three dimensional structures. The latest results show an increase of the folding entropy that induced by the modified cations increase disorder in the ensemble of unfolded states. This is attributed mainly due to differences in the solvation of natural and unnatural backbones [Citation124]. Although natural proteins are made up exclusively of α-amino acids, numerous unnatural polypeptides exhibit ordered folds with interesting biological activities. The thermodynamics of the backbone is determined by the non-covalent interactions. However, the rules used in most studies have been developed with peptides and proteins composed of α amino acids, which leads to the question of whether our understanding is only valid for conventional peptides, or whether it is truly molecular in nature [Citation125]. In natural biological systems amino acids having both the amine and the carboxylic acid groups attached to the rest (alpha-) carbon atom are almost exclusively present. New folded backbones, including hybrids of aromatic and aliphatic backbones, will lead to increasingly diverse platforms for recognition of a variety of biomolecules and surfaces. In general, replacing ɑ-residues with β3-residues is enthalpically unfavorable and entropically favorable to the thermodynamic stability of the tertiary structure [Citation124]. A key structural difference between ɑ-residues and β3-residues are an additional rotatable bond in the backbone, which results in increased conformational flexibility. Chandramouli et al. gave a beautiful example of the design of an artificial helically folded aromatic oligoamide for the selective encapsulation of fructose [Citation126]. The molecular recognition of saccharides by both natural and artificial receptors is seriously challenged by the issues of affinity and selectivity. Using fructose as a test case, extensive and unprecedented structural information about saccharide recognition at the atomic level was provided, shedding light on the binding mechanism of these intractable natural substrates [Citation127].
Conclusions
In conclusion, synthetic biology has been gaining importance during the past decade. The biological cell-like structures that exhibit a few key characteristics of living biological cells have been used to construct artificial cells by either the top-down or bottom-up approach, including the building of non-living materials. Nowadays, scientists are consistently relating various genetic materials to produce a wide range of consumer products, from biofuels to cosmetics. In medicine, synthetic biology has emerged as a relatively young field that is pushing boundaries in the creation of microbes that are able to destroy cancer cells in the human body and also clean up our environment. The advantages of using artificial cells are as follows: (a) engineered organisms are replaced to produce pharmaceuticals and fuels; (b) a better way to understand and investigate cellular life; (c) the non-living is connected with the living world; (d) applications in biomedical fields, medical imaging, and drug delivery; and (e) many new functions which are absent in biological cells can be added.
Challenges, opportunities and future perspectives
Substantial research has been conducted on artificial cells with biological cell-like architecture and some of the key properties of living biological cells. Artificial cells can be created in two ways: top-down, in which non-essential genes are taken out of organisms or completely replaced with synthetic ones; or bottom-up, in which non-living materials are assembled from scratch. Non-typical artificial cells, often known as ‘cell mimics’ are materials that have surface features, forms, morphology, or functions that are similar to those of biological cells. Many questions remain unanswered despite an impressive development to date, such as (i) methods by which artificial cells can communicate with each other and with the surrounding environment; (ii) procedures to produce artificial cell constructs; (iii) a technique to improvise the evolution, replication, and division of artificial cells; and (iv) a process by which artificial cells can take in nutrients and act as living organisms, (v) learning processes and memory, (homeostasis regulation). Answering these questions will put our technology and basic understanding of biological cells to the test. Although creating ‘completely living” artificial cells is still a long way off, progress toward this end goal will almost certainly bring many benefits and new applications. Artificial cells have the potential to provide a variety of advantages, including (i) providing a less-interfering way to investigate and understand cellular life, (ii) connecting the non-living to the living world, (iv) replacing engineered organisms to produce pharmaceuticals and fuels, (v) biomedical applications such as replacing or supplementing deficient cells, drug delivery, or medical imaging, and (vi) adding new functions that are absent in biological cells. Artificial cell creation will undoubtedly open up exciting new possibilities in industries like biotechnology, medicine, and industry.
The stepwise progress in development of artificial cells is due to a major progress in other areas such as polymer chemistry, bio-material, biotechnology, genomics and molecular biology. It is expected that unlimited progress in the area of artificial cells is foreseen due to the important progress in other fields. Artificial cells are now made in many dimensions (nano, micro, macro and molecular dimensions). The surface of the enormous potential of artificial cells is touched in spite of the unlimited variations of the artificial cell membranes and their contents. The new developments and expansion of ‘artificial cells’ to be concealed under various new names such as polymersome, bioencapsulation, polymer tethered lipid, nanotubules, nanocapules, conjugate hemoglobin etc. The interdisciplinary field of artificial cells needs researchers from other fields to come together and move the field ahead which will result in the progress of the ‘artificial cells’ beyond one vision. The principle of artificial cells play an important role in the field of enzyme and gene therapy, blood substitutes, drug delivery, regenerative medicine, genome editing, nanomedicine, hemoperfusion, nano-computers, nano-robotics, nano-sensors, agriculture, aquatic culture, cells and stem cell therapy.
The future of artificial cells will be important for environmental purposes, nanomedecine, and material science. The design of new artificial cells able to recycle organic waste and filter water will be of great importance for keeping a safe ecosystem. The development of artificial cells for nanomedecine will be important in combination of CAR-T cell therapy for the treatment of diseases such as cancers, infectious diseases. It requires nano-printing of desired biochemical networks, epigenetic regulations, receptors-signal transmission, retrocontrol systems, homeostatic far-from-equilibrium systems, release of molecules upon demand. To mention a few examples of the other uses of artificial cells in nanomedicine are the miniaturization of medical devices using artificial cells made up of ultrathin membranes, use of artificial cells in the modification of hemoglobin to polyhemoglobin as an oxygen carrier in transfusions, to remove oxygen radicals, oxygen and carbon dioxide with enhanced antioxidant activity was created by assembling hemoglobin with enzymes by nanobiotechnology, conjugated hemoglobin and production of blood substitutes. The relationship between artificial cells and polymer chemistry had resulted in the creation of artificial kidneys, oxygenators, artificial hearts, and vascular prothesis and other artificial replacements, enzyme immobilization, receptors and immunogens.
Acknowledgments
This work was supported by grant from Nano-Micro Architecture Chemistry (NMAC), College of Chemistry, Jilin University, China.
Disclosure statement
No potential conflict of interest was reported by the author(s).
Correction Statement
This article has been corrected with minor changes. These changes do not impact the academic content of the article.
References
- Santos-Merino M, Singh AK, Ducat DC. New applications of synthetic biology tools for cyanobacterial metabolic engineering. Front Bioeng Biotechnol. 2019;7:33.
- Garrett WS. From cell biology to the microbiome: an intentional infinite loop. J Cell Biol. 2015;210(1):7–8.
- Riley LA, Guss AM. Approaches to genetic tool development for rapid domestication of non-model microorganisms. Biotechnol Biofuels. 2021;14(1):30.
- Endy D. Foundations for engineering biology. Nature. 2005;438(7067):449.
- Kobayashi T, Horiuchi T, Tongaonkar P, et al. SIR2 regulates recombination between different rDNA repeats, but not recombination within individual rRNA genes in yeast. Cell. 2004;117(4):441–453.
- Tominaga M, Nozaki K, Umeno D, et al. Robust and flexible platform for directed evolution of yeast genetic switches. Nat Commun. 2021;12(1):1846.
- Li X, Daniel R. Synthetic nonlinear computation for genetic circuit design. Curr Opin Biotechnol. 2022;76:102727.
- Xu C, Hu S, Chen X. Artificial cells: from basic science to applications. Mater Today (Kidlington). 2016;19(9):516–532.
- Chang TM. Artificial cells in medicine and biotechnology. Appl Biochem Biotechnol. 1984;10(1–3):5–24.
- Yanagisawa M, Iwamoto M, Kato A, et al. Oriented reconstitution of a membrane protein in a giant unilamellar vesicle: experimental verification with the potassium channel KcsA. J Am Chem Soc. 2011;133(30):11774–11779.
- Schroeder A, Goldberg MS, Kastrup C, et al. Remotely activated protein-producing nanoparticles. Nano Lett. 2012;12(6):2685–2689.
- Ding Y, Wu F, Tan C. Synthetic biology: a bridge between artificial and natural cells. Life. 2014;4(4):1092–1116.
- O’Flaherty DK, Zhou L, Szostak JW. Nonenzymatic template-directed synthesis of mixed-sequence 3′-NP-DNA up to 25 nucleotides long inside model protocells. J Am Chem Soc. 2019 Jul 3; 141(26):10481–10488.
- Agarwal R, Iezhitsa I, Agarwal P, et al. Liposomes in topical ophthalmic drug delivery: an update. Drug Deliv. 2016;23(4):1075–1091.
- Garcia-Morales L, Ruiz E, Gourgues G, et al. A RAGE based strategy for the genome engineering of the human respiratory pathogen mycoplasma pneumoniae. ACS Synth Biol. 2020 Oct 16; 9(10):2737–2748.
- Malinova V, Nallani M, Meier W, et al. Synthetic biology, inspired by synthetic chemistry. FEBS Lett. 2012;586(15):2146–2156.
- Dzieciol AJ, Mann S. Designs for life: protocell models in the laboratory. Chem Soc Rev. 2012;41(1):79–85.
- Xu C, Hu S, Chen X. Artificial cells: from basic science to applications. Mater Today. 2016;19(9):516–532.
- Fookes MC, Hadfield J, Harris S, et al. Mycoplasma genitalium: whole genome sequence analysis, recombination and population structure. BMC Genomics. 2017;18(1):993.
- Cello J, Paul AV, Wimmer E. Chemical synthesis of poliovirus cDNA: generation of infectious virus in the absence of natural template. science. 2002;297(5583):1016–1018.
- Gil R, Silva FJ, Peretó J, et al. Determination of the core of a minimal bacterial gene set. Microbiol Mol Biol Rev. 2004;68(3):518–537.
- Gibson DG, Glass JI, Lartigue C, et al. Creation of a bacterial cell controlled by a chemically synthesized genome. science. 2010;329(5987):52–56.
- Kuruma Y, Stano P, Ueda T, et al. A synthetic biology approach to the construction of membrane proteins in semi-synthetic minimal cells. biochimica Et Biophysica Acta (BBA) - Biomembranes. 2009;1788(2):567–574.
- Blain JC, Szostak JW. Progress toward synthetic cells. Annu Rev Biochem. 2014;83(1):615–640.
- Von Neumann J. The general and logical theory of automata. Cerebral mechanisms in behavior. 1951;1:1–2.
- Bedau M, Church G, Rasmussen S, et al. Life after the synthetic cell. Nature. 2010;465:422–424.
- Mutalik VK, Guimaraes JC, Cambray G, et al. Precise and reliable gene expression via standard transcription and translation initiation elements. Nat Methods. 2013;10(4):354–360.
- Smolke CD, Silver PA. Informing biological design by integration of systems and synthetic biology. Cell. 2011;144(6):855–859.
- Lentini R, Santero SP, Chizzolini F, et al. Integrating artificial with natural cells to translate chemical messages that direct E. coli behaviour. Nat Commun. 2014;5(1):4012.
- Tang T-YD, van Swaay D, deMello A, et al. In vitro gene expression within membrane-free coacervate protocells. Chem Commun. 2015;51(57):11429–11432.
- Sun S, Li M, Dong F, et al. Chemical signaling and functional activation in colloidosome-based protocells. Small. 2016;12(14):1920–1927.
- Qiao Y, Li M, Booth R, et al. Predatory behaviour in synthetic protocell communities. Nat Chem. 2017;9(2):110–119.
- Walde P, Umakoshi H, Stano P, et al. Emergent properties arising from the assembly of amphiphiles. Artificial vesicle membranes as reaction promoters and regulators. chem. Commun 2014;50(71):10177–10197.
- Mann S. Systems of creation: the emergence of life from nonliving matter. Acc Chem Res. 2012;45(12):2131–2141.
- Mann S. The origins of life: old problems, new chemistries. Angew Chem Int Ed. 2013;52(1):155–162.
- Ryu M-H, Gomelsky M. Near-infrared light responsive synthetic c-di-GMP module for optogenetic applications. ACS Synth Biol. 2014;3(11):802–810.
- Armstrong JP, Shakur R, and Horne JP, et al. Artificial membrane-binding proteins stimulate oxygenation of stem cells during engineering of large cartilage tissue. Nat Commun. 2015;17(6):7405.
- Feliciano A, Cabral J, Prazeres D. Quantitative structure activity relationships in the synthesis of AcXYNH2 dipeptides by α-chymotrypsin in reversed micelles. enzyme and Microbial Technology. 1997;21(4):284–290.
- Zhang S, Blain JC, Zielinska D, et al. Fast and accurate nonenzymatic copying of an RNA-like synthetic genetic polymer. Proc Natl Acad Sci USA. 2013;110(44):17732–17737.
- Jia TZ, Hentrich C, Szostak JW. Rapid RNA exchange in aqueous two-phase system and coacervate droplets. origins of Life and Evolution of Biospheres. 2014;44(1):1–12.
- Elani Y. Construction of membrane-bound artificial cells using microfluidics: a new frontier in bottom-up synthetic biology. Biochem Soc Trans. 2016;44(3):723–730.
- Patwardhan B, Mutalik G, Tillu G. Integrative approaches for health: biomedical research. Ayurveda and Yoga: Academic Press); 2015.
- Andrianantoandro E, Basu S, Karig DK, et al. Synthetic biology: new engineering rules for an emerging discipline. Mol Syst Biol. 2006;2(1).
- Chang TMS. Artificial cells: biotechnology, nanomedicine, regenerative medicine, blood substitutes, bioencapsulation, and cell/stem cell therapy. World Scientific); 2007.
- Purnick PEM, Weiss R. The second wave of synthetic biology: from modules to systems. nature Reviews Molecular Cell biology. 2009;10(6):410–422.
- Rooke J. Synthetic biology as a source of global health innovation. systems and Synthetic Biology. 2013;7(3):67–72.
- Rabinow P, Bennett G. Synthetic biology: ethical ramifications 2009. systems and Synthetic Biology. 2009;3(1–4):99–108.
- Ross J, Arkin AP. Complex systems: from chemistry to systems biology. Proc Natl Acad Sci USA. 2009;106(16):6433–6434.
- Itoh H, Matsuoka S, Kreir M, et al. Design, synthesis and functional analysis of dansylated polytheonamide mimic: an artificial peptide ion channel. J Am Chem Soc. 2012;134(34):14011–14018.
- Yang H (2009). Synthetic biology and the future of man. international symposium on opportunities and challenges in the emerging field of synthetic biology. The National Academies’ Keck Center: Washington DC, pp. 9–10.
- Zhang JY. The ‘National’ and the ‘Cosmos’. EMBO Rep. 2011;12(4):302–306.
- Alterovitz G, Muso T, Ramoni MF. The challenges of informatics in synthetic biology: from biomolecular networks to artificial organisms. Brief Bioinform. 2010;11(1):80–95.
- Peng Y, Wu Q, Liu H, et al. Construction of specific artificial antigen-presenting cells for in vitro activation of CD19 chimeric antigen receptor T cells. Nan fang yi ke da xue xue bao =. J South Med Uni. 2017;37:581.
- Jefferson C, Lentzos F, Marris C. Synthetic biology and biosecurity: challenging the “Mythsâ€. Front Public Health. 2014;2.
- Ashkenasy G, Jagasia R, Yadav M, et al. Design of a directed molecular network. Proc Natl Acad Sci USA. 2004;101(30):10872–10877.
- Erbas-Cakmak S, Leigh DA, McTernan CT, et al. Artificial molecular machines. Chem Rev. 2015;115(18):10081–10206.
- Barber J, Tran PD. From natural to artificial photosynthesis. journal of the Royal society Interface. 2013;10(81):20120984.
- Kuijpers NG, Solis-Escalante D, Bosman L, et al. A versatile, efficient strategy for assembly of multi-fragment expression vectors in Saccharomyces cerevisiae using 60 bp synthetic recombination sequences. microbial Cell factories. 2013;12(1):47.
- Wang B, Steain MC, Dwyer DE, et al. Synthetic long oligonucleotides to generate artificial templates for use as positive controls in molecular assays: drug resistance mutations in influenza virus as an example. Virol J. 2011;8(1):405.
- Munshaw S, Bailey JR, Liu L, et al. Computational reconstruction of Bole1a, a representative synthetic hepatitis C virus subtype 1a genome. J Virol. 2012;86(10):5915–5921.
- Günther V, Strassen T, Lindert U, et al. Simian virus 40 strains with novel properties generated by replacing the viral enhancer with synthetic oligonucleotides. J Virol. 2012;86(6):3135–3142.
- Cottingham MG, Andersen RF, Spencer AJ, et al. Recombination-mediated genetic engineering of a bacterial artificial chromosome clone of modified vaccinia virus Ankara (MVA). PloS One. 2008;3(2):e1638.
- Schlabach MR, Hu JK, Li M, et al. Synthetic design of strong promoters. Proc Natl Acad Sci USA. 2010;107(6):2538–2543.
- P-W XE, Xie Y, X-J Z, et al. Inhibition of Dengue virus 2 replication by artificial micrornas targeting the conserved regions. Nucleic Acid therapeutics. 2013;23(4):244–252.
- Copple IM, Mercer AE, Firman J, et al. Examination of the cytotoxic and embryotoxic potential and underlying mechanisms of next-generation synthetic trioxolane and tetraoxane antimalarials. Mol Med. 2012;18(7):1045.
- Kissner TL, Ruthel G, Alam S, et al. Therapeutic inhibition of pro-inflammatory signaling and toxicity to staphylococcal enterotoxin B by a synthetic dimeric BB-loop mimetic of MyD88. PloS One. 2012;7(7):e40773.
- Klein J, Heal JR, Hamilton WDO, et al. Yeast synthetic biology platform generates novel chemical structures as scaffolds for drug discovery. ACS Synth Biol. 2014;3(5):314–323.
- Saragliadis A, Krajewski SS, Rehm C, et al. Thermozymes: synthetic RNA thermometers based on ribozyme activity. RNA Biol. 2013;10(6):1009–1016.
- Ye X, Liu Z, Hemida MG, et al. Targeted delivery of mutant tolerant anti-coxsackievirus artificial microRNAs using folate conjugated bacteriophage Phi29 pRNA. PloS One. 2011;6(6):e21215.
- Bhagwat B, Chi M, Han D, et al. Construction, and validation of artificial microrna vectors using agrobacterium-mediated transient expression system. Methods Mol Biol. 2016;1405:149–162.
- Franco E, Friedrichs E, Kim J, et al. Timing molecular motion and production with a synthetic transcriptional clock. Proc Natl Acad Sci USA. 2011;108(40):E784–E93.
- Stein V, Alexandrov K. Protease-based synthetic sensing and signal amplification. Proc Natl Acad Sci USA. 2014;111(45):15934–15939.
- Shao Z, Zhao H. Exploring DNA assembler, a synthetic biology tool for characterizing and engineering natural product gene clusters. Meth Enzymol. 2012;517:203.
- Tian B, Lieber CM. Synthetic nanoelectronic probes for biological cells and tissues. Annu Rev Anal Chem. 2013;6(1):31–51.
- Kalos M, June CH. Adoptive T cell transfer for cancer immunotherapy in the era of synthetic biology. Immunity. 2013;39(1):49–60.
- Tanaka M, Butler MO, Ansén S, et al. Induction of HLA-DP4–restricted anti-survivin Th1 and Th2 responses using an artificial antigen-presenting cell. Clin Cancer Res. 2011;17(16):5392–5401.
- Sayadi O, Shamsollahi MB, Clifford GD. Synthetic ECG generation and Bayesian filtering using a Gaussian wave-based dynamical model. physiological Measurement. 2010;31(10):1309.
- Yoo SY, Merzlyak A, Lee S-W. Synthetic phage for tissue regeneration. Mediators Inflamm. 2014;2014:1–11.
- Katayama N, Kato H, Taguchi Y, et al. The effects of synthetic oligopeptide derived from enamel matrix derivative on cell proliferation and osteoblastic differentiation of human mesenchymal stem cells. Int J Mol Sci. 2014;15(8):14026–14043.
- Stephan MT, Irvine DJ. Enhancing cell therapies from the outside in: cell surface engineering using synthetic nanomaterials. Nano Today. 2011;6(3):309–325.
- Garriga-Canut M, Agustín-Pavón C, Herrmann F, et al. Synthetic zinc finger repressors reduce mutant huntingtin expression in the brain of R6/2 mice. Proc Natl Acad Sci USA. 2012;109(45):E3136–E45.
- Forse GJ, Ram N, Banatao DR, et al. Synthetic symmetrization in the crystallization and structure determination of CelA from thermotoga maritima. Protein Sci. 2011;20(1):168–178.
- Sather AC, Berryman OB, Rebek J Jr. Synthesis of fused indazole ring systems and application to nigeglanine hydrobromide. Org Lett. 2012;14(6):1600–1603.
- Kamioka S, Ajami D, Rebek J. Autocatalysis and organocatalysis with synthetic structures. Proc Natl Acad Sci USA. 2010;107(2):541–544.
- Ceide SC, Trembleau L, Haberhauer G, et al. Synthesis of galmic: a nonpeptide galanin receptor agonist. Proc Natl Acad Sci USA. 2004;101(48):16727–16732.
- Organization WH. Guidelines for the treatment of malaria. Geneva, USA: World Health Organization); 2015.
- Clark RL. Embryotoxicity of the artemisinin antimalarials and potential consequences for use in women in the first trimester. Reprod Toxicol. 2009;28(3):285–296.
- Stano P, Luisi PL. Semi-synthetic minimal cells: origin and recent developments. Curr Opin Biotechnol. 2013;24(4):633–638.
- Chizzali C, Beerhues L. Phytoalexins of the Pyrinae: biphenyls and dibenzofurans. Beilstein J Org Chem. 2012;8:613.
- Wohlleben W, Mast Y, Muth G, et al. Synthetic biology of secondary metabolite biosynthesis in actinomycetes: engineering precursor supply as a way to optimize antibiotic production. FEBS Lett. 2012;586(15):2171–2176.
- Wang TJ, Larson MG, Vasan RS, et al. Metabolite profiles and the risk of developing diabetes. Nat Med. 2011;17(4):448–453.
- Chen R, Mias GI, Li-Pook-Than J, et al. Personal omics profiling reveals dynamic molecular and medical phenotypes. Cell. 2012;148(6):1293–1307.
- Wagner H, Dunker S, Liu Z, et al. Subcommunity FTIR-spectroscopy to determine physiological cell states. Curr Opin Biotech. 2013;24(1):88–94.
- Li Z, Chu L-Q, Sweedler JV, et al. Spatial correlation of confocal raman scattering and secondary ion mass spectrometric molecular images of lignocellulosic materials. Anal Chem. 2010;82(7):2608–2611.
- Sansone S-A, Fan T, Goodacre R, et al. The metabolomics standards initiative. Nat Biotechnol. 2007;25:846–848.
- Zhang A, Sun H, Xu H, et al. Cell metabolomics. OMICS. 2013;17(10):495–501.
- Guharoy M, Chakrabarti P. Conserved residue clusters at protein-protein interfaces and their use in binding site identification. BMC bioinformatics. 2010;11(1):286.
- Lua RC, Marciano DC, Katsonis P, et al. Prediction and redesign of protein–protein interactions. Prog Biophys Mol Biol. 2014;116(2–3):194–202.
- Babu MM, Kriwacki RW, Pappu RV. Versatility from protein disorder. science. 2012;337(6101):1460–1461.
- Chu L-H, Chen B-S. Construction of a cancer-perturbed protein-protein interaction network for discovery of apoptosis drug targets. BMC Syst Biol. 2008;2(1):56.
- Nandy SK, Jouhten P, Nielsen J. Reconstruction of the yeast protein-protein interaction network involved in nutrient sensing and global metabolic regulation. BMC Syst Biol. 2010;4(1):68.
- Cheng S, Zhang Y, Brooks CL. PCalign: a method to quantify physicochemical similarity of protein-protein interfaces. BMC bioinformatics. 2015;16(1):33.
- Muley VY, Ranjan A, Ouzounis CA. Evaluation of physical and functional protein-protein interaction prediction methods for detecting biological pathways. PloS One. 2013;8(1):e54325.
- Zhang S, Zhang N, Blain JC, et al. Synthesis of N3′-P5′-linked phosphoramidate DNA by nonenzymatic template-directed primer extension. J Am Chem Soc. 2013;135(2):924–932.
- Ichida JK. High fidelity TNA synthesis by therminator polymerase. nucleic Acids research. 2005;33(16):5219–5225.
- Chen JJ, Cai X, Szostak JW. N2′→P3′ phosphoramidate glycerol nucleic acid as a potential alternative genetic system. J Am Chem Soc. 2009;131(6):2119–2121.
- Blain JC, Ricardo A, Szostak JW. Synthesis and nonenzymatic template-directed polymerization of 2′-amino-2′-deoxythreose nucleotides. J Am Chem Soc. 2014;136(5):2033–2039.
- Dartois V, Sanchez-Quesada J, Cabezas E, et al. Systemic antibacterial activity of novel synthetic cyclic peptides. Antimicrob Agents Chemother. 2005;49(8):3302–3310.
- Vickers CJ, Olsen CA, Leman LJ, et al. Discovery of HDAC inhibitors that lack an active site Zn 2+ -binding functional group. ACS Med Chem Lett. 2012;3(6):505–508.
- Horne WS, Ashkenasy N, Ghadiri MR. Modulating charge transfer through CyclicD,L-?-Peptide self-assembly. Chem Eur J. 2005;11(4):1137–1144.
- Montero A, Beierle JM, Olsen CA, et al. Design, synthesis, biological evaluation, and structural characterization of potent histone deacetylase inhibitors based on cyclic α/β-tetrapeptide architectures. J Am Chem Soc. 2009;131(8):3033–3041.
- Rokitskaya TI, Nazarov PA, Golovin AV, et al. Blocking of single α -hemolysin pore by rhodamine derivatives. Biophys J. 2017;112(11):2327–2335.
- Sevim S, Sorrenti A, Franco C, et al. Self-assembled materials and supramolecular chemistry within microfluidic environments: from common thermodynamic states to non-equilibrium structures. Chem Soc Rev. 2018;47(11):3788–3803.
- Phillips J. Scaling and self-organized criticality in proteins I. Proc Natl Acad Sci USA. 2009;106(9):3107–3112.
- Conti Nibali V, Havenith M. New insights into the role of water in biological function: studying solvated biomolecules using terahertz absorption spectroscopy in conjunction with molecular dynamics simulations. J Am Chem Soc. 2014;136(37):12800–12807.
- Dielmann-Gessner J, Grossman M, Nibali VC, et al. Enzymatic turnover of macromolecules generates long-lasting protein–water-coupled motions beyond reaction steady state. Proc Natl Acad Sci USA. 2014;111(50):17857–17862.
- Ryde U. A fundamental view of enthalpy–entropy compensation. Med Chem Comm. 2014;5(9):1324–1336.
- Kennedy IR, Hodzic M. Partitioning entropy with action mechanics: predicting chemical reaction rates and gaseous equilibria of reactions of hydrogen from molecular properties. Entropy (Basel). 2021 16;23(8):1056.
- Tsuchiya M, Giuliani A, Hashimoto M, et al. Emergent self-organized criticality in gene expression dynamics: temporal development of global phase transition revealed in a cancer cell line. PloS One. 2015;10(6):e0128565.
- Alain C, Roye A, Salloum C. Effects of age-related hearing loss and background noise on neuromagnetic activity from auditory cortex. Front Syst Neurosci. 2014;8:8.
- Phillips J. Scaling and self-organized criticality in proteins II. Proc Natl Acad Sci USA. 2009;106(9):3113–3118.
- Mora T, Bialek W. Are biological systems poised at criticality? J Stat Phys. 2011;144(2):268–302.
- Naderi M. On the evidence of thermodynamic self-organization during fatigue: a review. Entropy (Basel). 2020;22(3):372.
- Reinert ZE, Horne WS. Folding thermodynamics of protein-like oligomers with heterogeneous backbones. Chem Sci. 2014;5(8):3325–3330.
- Teng P, Zheng M, Cerrato DC, et al. The folding propensity of α/sulfono-γ-AA peptidic foldamers with both left- and right-handedness. Commun Chem. 2021;4(1):58.
- Chandramouli N, Ferrand Y, Lautrette G, et al. Iterative design of a helically folded aromatic oligoamide sequence for the selective encapsulation of fructose. Nat Chem. 2015;7(4):334–341.
- Lucia U, Grisolia G. Thermal physics and glaucoma: from thermodynamic to biophysical considerations to designing future therapies. applied Sciences. 2020;10(20):7071.