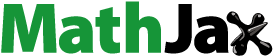
Abstract
In the view of the total energy consumption by economic sectors of Thailand, it was shown that the greatest energy consumption derived from the industrial sector, where consumed both thermal energy and electrical energy for the processes. In this study, a novel concept of Organic Rankine Cycle (ORC) power generation is proposed. The power is generated from a low-grade industrial waste heat (IWH) with temperature below 70°C. The system is also combined with a solar water heating system (SWHS) and a vapor compression heat pump (VCHP) as a heating booster. A 400 kW thermal capacity VCHP, with R365mfc as the working fluid, is used to rise the heat from IWH and SWHS before supplying to a 60 kWe ORC power generator with R245fa. Three types of solar collectors were used to generate heat: flat-plate, heat pipe evacuated-tube and compound parabolic concentrator (CPC). Between 300 and 700 units of each type of the collectors were connected in parallel with of 0.740, 0.572, 0.718, FRUL of 3.620, 0.750, 0.974 W/m2-K, and gross area of 2.081, 2.369, 2.160 m2 per unit, respectively. The system is designed to produces 10 m3/day of hot water at 70°C. The system is mathematically modeled and simulated to evaluate the net power output, the CO2 emission, and the levelized cost of electricity (LCOE). Six areas of industrial estate consisting of, Chiang Mai (18.80°N, 98.98°E), Bangkok (13.75°N, 100.52°E), Ratchaburi (13.54°N, 99.82°E), Songkhla (7.21°N, 100.56°E), Nakhon Ratchasima (13.75°N, 100.52°E), and Chon Buri (13.40°N, 101.00°E), that represent the north, central, west, south, north-east and east part of Thailand. Their weather data was taken for the simulations. The simulation results show that the system produces high electricity when the number of the collectors is increased. Moreover, the system located in Chiang Mai produced the highest amount of electricity with the lowest LCOE. When the temperature of low-grade IWH was around 64°C, with 700 solar collector units of each type of flat-plate, heat pipe evacuated-tube, and compound parabolic concentrator (CPC) solar collectors, the system can produce 84.4, 107.0, and 117.1 MWh/Year with LCOE of 0.35, 0.28, and 0.25 USD/kWh, respectively. In terms of the environmental impact, the system can reduce CO2 emission of 46.2, 58.6, and 64.2 Ton CO2 eq./Year, respectively. From this study, it can be concluded that the VCHP-ORC system can be integrated with the SWHS and used in industrial processes for power production as well as reduction of the energy intensity and CO2 emission of the industries.
Public interest statement
In industrial processes, there is approximately 20 to 50% of energy supply wasted and released into the environment. Unfortunately, a large amount of the wasted has the temperature below 70°C and cannot directly be converted into electricity by an Organic Rankine Cycle (ORC) power generation. In this research, a concept for a VCHP-ORC power generation from a low-grade industrial waste heat with temperature below 70°C combined with solar water heating system (SHWS) was proposed and investigated. Three types of solar collectors consisting of flat-plate, heat pipe evacuated-tube, and compound parabolic concentrator (CPC) solar collectors were compared in terms of heat generation to feed the system. Six areas of industrial estate of Thailand were taken as input weather data for the simulations. The system was mathematically modeled and simulated to evaluate the net power output, the environmental impact, and the levelized cost of electricity (LCOE) of the system.
1. Introduction
Energy demand is continuously growing as the world economy expands. There will be more demand of fuel required for higher levels of activity and living standards (BP Energy Outlook 2017 Edition, Citation2017). From BP Energy reports, the world’s primary energy consumption increased from 10.9 GTOE in 2005 to 13.2 GTOE in 2015, with a growth rate of 1.9% per year (Statistical Review of World Energy, Citation2017), and is expected to increase by 34% between 2014 and 2035 (BP energy Outlook 2016 Edition, Citation2016). In Thailand, it is predicted that the total energy consumption in 2036 will be around 131.0 MTOE with an increase of 40.5% as compared with that of 2015 (Energy Policy & Planning Office, Citation2017). Moreover, the total energy consumption classified by-economic sectors showed that the greatest share of 36.6% derived was from consumption of thermal and electrical energy in the industrial sector (Department of Alternative Energy Development & Efficiency, Citation2017a). Therefore, reduction of energy consumption or increase of energy efficiency in industries refers to energy saving of the whole country. Thailand is considered as one of the countries having the highest average total solar radiation with less annual direct normal solar radiation within the range of 1,350–1,400 kWh/m2-year (Department of Alternative Energy Development & Efficiency, Citation2006). Thus, it is insufficient for the Concentrating Solar Power (CSP) technology requiring the radiation above 1,500 kWh/m2-year (Domínguez Bravo, García Casals, & Pinedo Pascua, Citation2007; International Energy Agency, Citation2017; Purohit & Purohit, Citation2010). Therefore, the low grade heat, as a form of low-to-medium temperature hot water is consumed in industries, hotels, hospitals, and etc. Nowadays, the Department of Alternative Energy Development and Efficiency (DEDE) of Ministry of Energy in Thailand has promoted and subsidized solar hot water system using waste heat such as waste heat from condensing units of air conditioners, industrial processes, etc. (Department of Alternative Energy Development & Efficiency, Citation2017b).
In the industrial sector, waste heat is one of the most abundant sources of energy, as it accounts for more than 2,000 Ton BTU/Year of wasted energy (Guillen & Zia, Citation2013). Moreover, industrial waste heat is the unusable heat generated from hot combustion gases discharged to the atmosphere, heated products exiting industrial processes, and heat transfer from hot equipment surfaces. From the research publication of BSC Incorporated (BSC Incorporated, Citation2008), it was reported that the quantity of industrial waste heat was as much as 20 to 50% of industrial energy consumption. In addition, 60% of the below 230°C heat released into the environment shows a large potential for heat recovery (BSC Incorporated, Citation2008; Viswanathan, Davies, & Holbery, Citation2006; U.S. Department of Energy’s Industrial Technologies Program’s, Citation2004). Unfortunately, this heat cannot directly be converted into electricity by a steam Rankine cycle because it becomes less profitable with below 340°C heat sources (BSC Incorporated, Citation2008). However, it is still productive for power generation by employing an Organic Rankine Cycle (ORC) system (Bao, Zhao, & Zhang, Citation2011; Calise, d’Accadia, Vicidomini, & Scarpellino, Citation2015; Jung, Taylor, & Krumdieck, Citation2015; Pei, Li, & Ji, Citation2010). The ORC system is a scalable thermodynamic solution for converting low-temperature thermal sources. It can be configured to meet variable project demands (Garg, Orosz, & Kumar, Citation2016) and is proved to be a reliable technology that can efficiently convert the low-to-medium grade heat sources into useful power (Rahbar, Mahmoud, Al-Dadah, Moazami, & Mirhadizadeh, Citation2017). Moreover, it is possible to be implemented in decentralized lower-capacity power plants (Quoilin & Lemort, Citation2009; Quoiln, Van Den Broek, Sébastien, Dewallef, & Lemort, Citation2013).
Recently, many researchers are working on the design, analysis, and development of ORC systems for low-temperature waste heat conversion. Tchanche, Lambrinos, Frangoudakis, and Papadakis (Citation2011) showed that the market of the ORC in waste heat recovery (ORC-WHR) application grows faster among the ORC solutions with an enormous potential in industry and combined cycle power plant. Campana et al. (Citation2013) evaluated the energy savings and CO2 emission of the ORC units based on real operating data of cement, steel, glass, and oil & gas industries. Liu, Zhou, Zhao, and Wang (Citation2015) designed and modified a hybrid energy supply system, including the gas engine-driven heat pumps system and ORC using a gas engine waste heat as low-grade heat source in order to transfer the low-grade gas engine waste heat into high-grade electricity by ORC. Van de Bor, Infante Ferreira, and Kiss (Citation2015) investigated the potential of several alternative technologies for upgrading the low-temperature waste heat such as compression-resorption, vapor compression and trans-critical heat pumps, or for the conversion by using organic Rankine, Kalina and trilateral cycle engines. Chaiyat (Citation2014) proposed a concept to generate electricity from low-temperature heat by using an absorption heat transformer (AHT) coupled with an ORC. In addition, Chaiyat and Kiatsiriroat (Citation2015) studied the concept of combined cooling heating and power (CCHP) of a 25 kWe R245fa ORC system integrated with a 20 kW LiBr-H2O absorption chiller. Sonsaree, Asaoka, Jiajitsawat, Aguirre, and Tanaka (Citation2016a) presented power generation using the ORC system combined with gas engine-driven heat pump (GEHP) by utilizing the low-grade industrial waste heat. It was found that, the GEHP-ORC system is applicable for an industry with low-temperature heat sources.
For concentrating and non-concentrating solar collectors combined with the ORC power generation, Bocci et al. (Citation2015) analyzed of a power plant producing electrical power, heat, cooling and fresh water needs for a house. The power plant included compound parabolic concentrator (CPC) solar collectors, the thermal storage tank, the ORC system, the absorption chiller system, and the reverse osmosis desalination unit. Delgado-Torres and García-Rodríguez (Citation2010) presented the theoretical analysis of the solar thermal driven seawater and brackish water reverse osmosis desalination technology. The system was analyzed of twelve substances as working fluids of the ORC system and four different models of stationary solar collectors (flat-plate, CPC, and evacuated-tube solar collectors) are taken into account. Baral and Kim (Citation2014) presented a solar ORC power plant for combined heat and power (CHP) application in Busan, Korea. Flat plate solar collectors were analyzed for the ORC power plant with 90°C as the hot fluid temperature, whereas vacuum tube solar collectors were analyzed for the ORC power plant with 125°C as the hot fluid high temperature. Zhang et al. (Citation2006) presented of a transcritical CO2 Rankine cycle for CHP application. The system included evacuated-tube solar collectors, the ORC system, the heat recovery system, and the feed pump. The results suggested that the cycle has a good potential for distributed energy supply system. Freeman, Hellgardt, and Markides (Citation2015) investigated the potential of a small-scale combined solar heat and power (CSHP) system based on an ORC for provision of hot water and power for domestic use in the UK.
Moreover, there is an ongoing research on ORC system for low-grade heat conversion from renewable resources (e.g. solar energy, geothermal, etc.) combined with low-grade industrial waste heat. Garg et al. (Citation2016) evaluated various working fluids for an ORC system coupled to waste heat and solar source. It was found that for this system, R152a and R161 yielded the lowest investment cost. Li, Li, Pei, Munir, and Ji (Citation2016) proposed a cascade ORC and an optimization criterion for the hybridization of solar energy and LNG for power generation. In the system, energy from solar collectors drives the evaporation of the working fluid in the top cycle. The heat released by the top cycle facilitates the evaporation of the working fluid in the bottom cycle, and LNG is used for the cold source of the bottom cycle. Higgo and Zhang (Citation2015) designed and fabricated a small-scale ORC power generation by utilizing waste heat from high-concentration photovoltaic (HCPV) arrays, and solar energy from evacuated-tube solar collectors. Pikra, Rohmah, Pramana, and Purwanto, (Citation2015) analyzed the potential of ORC power generation from the hot springs in Indonesia, with heat source temperatures between 70 and 80°C. Chaiyat (Citation2015a) studied the possibility of power generation by using alternative energy in Thailand which are geothermal energy, solar energy and waste heat based on the energy and economy indicators. Sonsaree, Asaoka, Jiajitsawat, Aguirre, and Tanaka (Citation2016b) proposed a novel concept of ORC power generation from industrial waste heat recovery (IWHR) combined with solar water heating system (SWHS) by using a vapor compression heat pump (VCHP) as heating booster. It was found that the number of solar collectors affects the system in terms of the economic and the environmental impact.
From the literature review, it can be seen that the ORC system has been widely applied for power generation from heat sources such as solar energy, geothermal energy, wasted heat from industrial processes, etc. However, it is less applicable for below 70°C heat sources due to a combination of market and technical barriers (Quoilin & Lemort, Citation2009; Tchanche, Pétrissans, & Papadakis, Citation2014). Moreover, there are only a few designed to utilize lower temperature thermal energy supplies on a small scale ORC. If an ORC system can be applied for power generation at below 70°C heat sources, the industrial sector could benefit from this waste heat as well as reduction of the energy intensity, increase of energy efficiency of industrial processes (Huang, Zheng, Baleynaud, & Lu, Citation2016), and reduce of pollutants (greenhouse gas emissions (GHG), and thermal pollution). Furthermore, in the future, the system can improve or refine the application or processes involved in generating electricity from low-temperature heat source. From the above mentioned (Huang et al., Citation2016; Quoiln et al., Citation2013; Sonsaree et al., Citation2016a, Citation2016b; Tchanche et al., Citation2011), to rise the low-temperature heat to the high-temperature heat is an interesting approach for ORC power generation. This system would help the strategy of Ministry of Energy in Thailand to reduction of the energy intensity around 30% in 2036 (Energy Policy & Planning Office, Citation2017). In this research, such system is proposed with the use of existing technology, a vapor compression heat pump (VCHP), to rise the low-temperature heat source to the high-temperature heat sink before supplying it to the ORC power generation. The objectives of this research are:
• | Mathematical modeling and simulating to evaluate the net power output, the environmental impact, and the levelized cost of electricity (LCOE) of the VCHP-ORC power generation from low-grade industrial waste heat (IWH) combined with solar water heating system (SWHS). | ||||
• | The evaluation of three types of solar collectors consisting of flat-plate, heat pipe evacuated-tube, and compound parabolic concentrator (CPC) used for hot water generation. | ||||
• | Test of the system in six representative areas of industrial estate in Thailand consisting of Chiang Mai (18.80°N, 98.98°E), Bangkok (13.75°N, 100.52°E), Ratchaburi (13.54°N, 99.82°E), Songkhla (7.21°N, 100.56°E), Nakhon Ratchasima (13.75°N, 100.52°E), and Chon Buri (13.40°N, 101.00°E) by using their weather data during the simulations. |
However, it could be noted that based on the above mentioned literature review, there is no adequate knowledge of this topic in recent works of literature.
2. Descriptions and simulation conditions of system
A schematic diagram of the VCHP-ORC power generation from low-grade IWH combined with SWHS is shown in Figure . The main components of the system are: solar collectors, the VCHP system, and the ORC system. In the system operation, during the daytime or sunrise as shown in Figure (a): low-grade IWH at constant quantity () and quality (TWH,i) is used to increase or maintain the outlet temperature of hot water from the ORC system (TORC,o) before supplying it to the solar collectors (TColl,i) for hot water production. In this step, if the outlet hot water temperature from solar collectors (TColl,o) is equal to or higher than 70°C, one part of the heat is supplied for industrial usage and the other part is directly supplied to the ORC system (TORC,i) for power generation. Otherwise, the temperature is raised by the VCHP system as shown in Figure (b), to an equal-to or higher temperature heat and then is stored in the heat reservoir/thermal storage tank. After that, the heat from the thermal storage tank is supplied to the industrial process and the ORC system (TORC,i) to generate electricity. During the nighttime or sunset as shown in Figure (b): low-grade IWH with the same quantity (
) and quality (TWH,i) is directly supplied to the VCHP system where the temperature is raised before supplying it to the industrial process and the ORC power generation. Moreover, when the VCHP system was used to raise the low-temperature heat, the power generation from the ORC system (WTur), has feedback to the VCHP system (WVCHP). After that, the net power output (WNet) will be supplied for industrial usage.
2.1. Solar water heating system (SWHS)
From Figure (a), the collectors absorb solar radiation and convert it into heat. This heat is then absorbed by a heat transfer fluid (HTF) (the water is used in this study) and then directly supplied for industrial usage and the ORC system for power generation. The energy equations of the SWHS are summarized as follows:
The rate of heat transfer from the solar collectors at any time ():
(1)
(1)
where TAmb is the ambient temperature which could be determined from (Chaichana, Kiatsiriroat, & Nuntaphan, Citation2010):(2)
(2)
where Tmax and Tmin are the maximum and minimum ambient temperature at each location as shown in the Appendix A.
For the proposed system, three types of solar collectors were compared, consisting of flat-plate, heat pipe evacuated-tubes, and CPC solar collectors of 300 to 700 units connected in parallel used for hot water generation. Which these numbers of solar collectors is appropriate as combined with a 60 kWe ORC power generation. The characteristics of the solar collectors such as aperture area (m2) (Aperture area is defined as the unobstructed cover area or the total cover area less the area of cover supports), gross area (m2) (Gross area is defined as the total area occupied by a collector module, that is, the total area of a collector array divided by the number of modules in the array), optical efficiency (), and overall heat transfer coefficient (FRUL, W/m2 − K) are shown in Table and Figure . The solar collector efficiency shown as follows:
(3)
(3)
Table 1. Characteristic of three types of solar collectors: flat-plate, heat pipe evacuated-tube, and CPC solar collectors
2.2. Vapor compression heat pump (VCHP)
The VCHP system, used as the heat boosting technology, as an energy storage technology presents unique advantages for environmental protection and energy usage (Fan, Zhang, Ju, & Wang, Citation2013). In addition, these technologies are more efficient than the electrical heating system (Amin & Hawlader, Citation2013; Aye, Charters, & Chaichana, Citation2002; Banister & Collins, Citation2015; Hepbasli & Kalinci, Citation2009; Ibrahim, Fardoun, Younes, & Louahlia-Gualous, Citation2014). Generally, it uses the external energy input to drive a cycle that extracts heat from the low-temperature heat source to the high-temperature heat sink/heat reservoir. The VCHP system is a thermal upgrading device driven by electrical power. In general, it consists of compressor, condenser, expansion valve, and evaporator. A schematic diagram of the VCHP system and corresponding T-s diagram are shown in Figure . At state 1, a low-temperature HTF of the VCHP system is heated by the heat source via a heat exchanger. From state 1 to 2, the HTF is compressed in the compressor to increase its temperature and pressure, and 2s is the corresponding states for the isentropic case. From states 2 to 3, the high temperature HTF is condensed by releasing the heat at the condenser via a heat exchanger and its pressure due to throttling effect. From state 3 to 4, the low-temperature HTF is entering the evaporator to restart next cycle.
The energy equations of the VCHP system are summarized as follows:
The rate of heat transfer to the VCHP evaporator ():
(4)
(4)
The rate of heat transfer to the VCHP condenser ():
(5)
(5)
The work input to the VCHP system ():
(6)
(6)
(7)
(7)
(8)
(8)
For this study, 400 kW thermal capacity of the VCHP system with R365mfc as working fluid is used to increase the below 70°C heat either from IWH or SWHS before supplying it to the ORC power generation. Moreover, the initial condition of the VCHP system consists of isentropic (ηComp,VCHP,s), mechanical (ηComp,ME) and motor (ηComp,MO) compressor efficiency set at 90, 95, and 95%, respectively. From the Equation (7), it can be seen that the mechanical and motor compressor efficiency have an effect on the work input to the VCHP system. When the efficiency was high, the power input or power requirement of the VCHP system become lower.
2.3. Organic Rankine cycle (ORC)
ORC is an alternative technology applicable for small-scale power generation. It is used for low-temperature heat recovery, by converting low-temperature thermal energy to electricity. The ORC system has the same operating principle of the steam Rankine cycle but an organic working fluid is used instead of water. Historically the cycle has been employed for the conversion of heat from low-to-medium temperature sources (e.g. geothermal, biomass combustion, process waste heat), with systems being operational, often with little need for maintenance, over 2–3 decades (Freeman et al., Citation2015). Moreover, the ORC technology is well established worldwide with a number of commercial systems in operation, and typical size ranges from the order of a few to 10 MW for a wide range of working fluids and operating temperatures (Vélez et al., Citation2012).
The ORC system mainly consists of condenser, pump, evaporator, turbine expander and generator. A schematic diagram of the ORC system and the corresponding T-s diagram are shown in Figure . At state 2, low-temperature heat transfer fluid (HTF) of the ORC system is heated by the heat source via a heat exchanger to state 3 at which the HTF is at saturated vapor state with high pressure through an evaporator. From state 3 to 4, the vapor is expanded through a turbine expander to generate power. Finally, it is condensed to a saturated liquid in the condenser at state 1 to complete the cycle. In addition, states 2a and 4a are the actual exit states of the pump and the turbine, respectively. In this study, 60 kWe ORC power generation with R245fa as working fluid (ORC model: MB-70H from KOBELCO Company (Kobelco, Citation2017)) was simulated to find a power generation of the system as shown in Figure and Table .
Figure 5. ORC model: MB-70H from KOBELCO Company (60 kWe electrical capacities with R245fa as working fluid) (Kobelco, Citation2017).
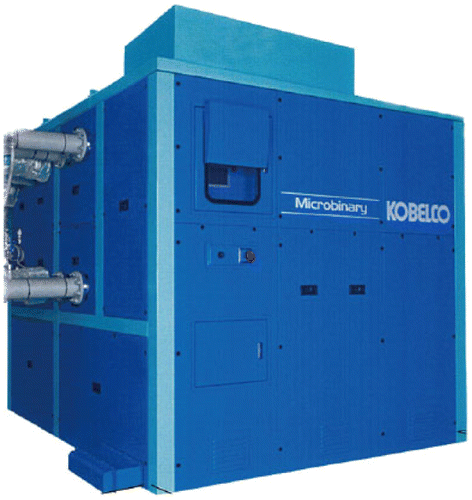
Table 2. The performance characteristic of ORC model: MB-70H from KOBELCO Company
In Table , the hot water temperature is the outlet temperature from solar collectors during the daytime or sunrise, and is from the VCHP system during the nighttime or sunset. In addition, the cooling water temperature is from the cooling tower. Furthermore, to find out the power output from the performance characteristic of ORC model: MB-70H from KOBELCO Company. For instance, when the hot water flow rate is 25 Ton/h, the cooling water, and the hot water temperature is 20 and 85°C, respectively. The ORC system can generate the power of 29 kWe.
2.4. Simulation conditions
In this study, six areas of industrial estate consisting of Chiang Mai (18.80°N, 98.98°E), Bangkok (13.75°N, 100.52°E), Ratchaburi (13.54°N, 99.82°E), Songkhla (7.21°N, 100.56°E), Nakhon Ratchasima (13.75°N, 100.52°E), and Chon Buri (13.40°N, 101.00°E) represent the north, central, west, south, north-east and east part of Thailand as shown in Figure . The weather data from these provinces was taken as input data for simulations consisting of ambient temperature (°C) and total average solar radiation on the tilted surface (kWh/m2-day) (Atmospheric Science Data Center, Citation2017) are shown in Appendix A. Moreover, solar radiation in terms of the hourly global radiation is estimation as presented in the studies of Zhang, Zhao, Deng, Xu, and Zhang (Citation2017); and Duffie and Beckman (Citation2013).
Figure 6. The location of Chiang Mai, Bangkok, Ratchaburi, Songkhla, Nakhon Ratchasima, and Chon Buri.
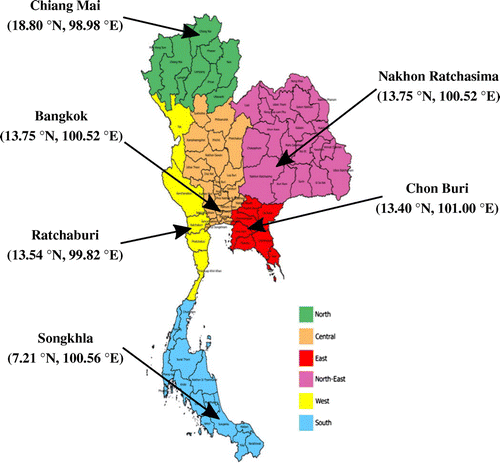
The system was mathematically modeled based on steady state condition. Heat loss from the system components such as the evaporator, the condenser, and the piping system was neglected. Also, the power input for the water pump consists of the collector, the condenser, and the supply pump as shown in Figure were neglected. The low-grade IWH, under the assumption of the waste heat temperature in the range of 60–70°C, and constant waste heat flow rate were used as input to the systems. It is used to increase or maintain the outlet temperature of hot water from the ORC system before supplying it to the solar collectors for hot water production during the daytime or sunrise. Moreover, the low-grade IWH is directly supplied to the VCHP system during the nighttime or sunset. The water temperature stored in the heat reservoir/thermal storage tank is assumed to be 70°C. In addition, the system normally produces 10 m3/day of hot water at 70°C. The degree of superheating, subcooling, and the pinch-point temperature difference was set at 5°C. In addition, heat exchanger effectiveness (ɛ) was assumed to be 90%. The thermodynamic properties of the VCHP system were calculated by REFPROP NIST7.0 (National Institute of Standard & Technology, Citation2000).
3. Economic analysis
The economic analysis of the integrated system was carried out in terms of the levelized cost of electricity (LCOE) as presented in the studies of (Chaiyat, Citation2015b; Chaiyat & Kiatsiriroat, Citation2015; Pitz-Paal, Dersch, & Milow, Citation2003), which could be calculated by:(9)
(9)
(10)
(10)
In the economic assessment, the initial condition and the commercial cost of the VCHP system was used to evaluate the capital cost of the system as shown in Table . Capital cost of the ORC power plant varies between 2,000 and 3,400 USD/kWe (Arvay, Muller, Ramdeen, & Cunningham, Citation2011; Rowshanzadeh, Citation2011; Senechal, Citation2017) as given in Table . From the table, was selected a micro scale ORC power plant for this study at around 2,500 USD/kWe. The investment in solar collectors is not included in the calculation, since the costs are partially subsidized by a project that promotes the use of SWHS to produce hot water in hotels, hospitals, and industries, of the DEDE (Department of Alternative Energy Development and Efficiency, Citation2017). From 2008 to 2015, it had been installed 46,139 m2 of solar collectors. Moreover, the Alternative Energy Development Plan (AEDP2015), has the target to support and subsidize the installation cost of 9.17 million-m2 of solar collectors for solar water heating systems (SWHS) used in hot water production by 2036 (Energy Policy & Planning Office, Citation2017).
Table 3. Initial condition, and cost data used for the economic evaluation of the system
Table 4. Commercial cost of the ORC power plant
4. Result and discussion
In this study, a concept for a VCHP-ORC power generation from a low-grade IWH combined with SWHS was proposed and investigated. Three types of solar collectors consisting of flat-plate, heat pipe evacuated-tube, and CPC solar collectors were compared in their heat output at six areas of industrial estate in Thailand. The results were compared based on the net power output, the environmental impact, and the LCOE of the system as shown in the following:
4.1. Net power output of the system
The net power output (MWh/Year) of the system, when the number of solar collectors (Units) and the heat source temperature (°C) increases are shown in Figure . The results showed that the more number of solar collectors are available and the higher the heat source temperature is the more electricity the system can generate. This means that the solar field can produce more thermal energy to supply to the ORC power generation. Moreover, when the heat source temperature increases, the power requirement of the VCHP system to increase the low-temperature heat source to the high-temperature heat sink/heat reservoir is reduced. Furthermore, the power output of the system, when the number of CPC solar collectors was higher than that 600 units, and the heat source temperature of 70°C. It was found that the power output of the system had decreased due to the collector outlet temperature over the temperature limit of the ORC power generation (95°C).
From Figure , it could be presented the factors that influence the power output by:(11)
(11)
where W is the power output (kWe). ηORC is the ORC system efficiency, it depends on the temperature of IWH and the number of solar collectors (Unit). qIWH is energy input from IWH (kWth), it is constant in this study. And ACollIT is energy input from the solar collectors, AColl is increases by the number of solar collectors (Unit).
4.2. Effect of the heat source temperature
According to the concept developed in this study, the VCHP system was used to increase the low-temperature heat source to the high-temperature heat sink/heat reservoir before supplying to the ORC power generation. The VCHP system is a thermal upgrading device driven by electrical power, with its power requirement depending on the temperature difference between the heat source and heat sink. Focusing on the heat source temperature, Figure shows the effect of the source on the hourly net power output of the system when the number of solar collectors is 700 units and the heat source temperature increases from 60 to 70°C. The results showed that, the heat source temperature impacted on the net power output of the system. The system can be operated during day and night time or 24 h per day, when the temperature of heat source is above 62°C. This means that, at this temperature the power output of the VCHP-ORC system was higher than the power fed back to the VCHP system.
Figure 8. Hourly net power output (kW) of the system, when the number of solar collectors is 700 units connected in parallel, and the heat source temperature increases from 60 to 70°C (Collector type: Flat-plate solar collectors and Location: Bangkok).
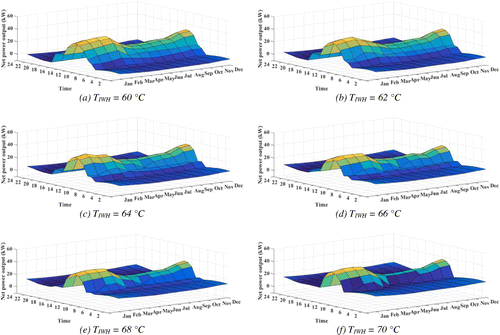
From Figure , it can be noted oscillation trend exist in the morning (7.00–10.00 am) and the afternoon (14.00–18.00 pm). It can be explained in terms of the energy balance of the system with consists of rate of heat transfer from solar collectors () (day time or sunrise), rate of heat transfer from the VCHP system (
) (nigh time or sunset), rate of heat transfer supply to the load (
), power output from the ORC system (
), and power input to the VCHP system (
) as shown in Figure . From the Figure, it seems that in the morning and the afternoon, the SWHS could maintain the thermal energy from the solar collectors (
) to supply the ORC power generation even though solar radiation was slightly low, because of the number of solar collectors available. In addition, at night time with the same quality and quantity of waste heat, the power output from the ORC system was higher when the ambient temperature was lower. In other words, the ORC system could produce the highest power at the lowest ambient temperature.
4.3. Net power output
As mentioned above, Figure shows a comparison of the net power output of three types of solar collectors, when the number of solar collectors were varied from 300 to 700 units connected in parallel, and the heat source temperature was around 64°C. The comparing results presented that with increment of 50 units, the system with CPC solar collector provided the net power outputs of 48.8, 53.7, 60.1, 67.1, 74.5, 82.6, 90.0, 97.7, and 98.7 MWh/Year, respectively due to the highest thermal energy and collector outlet temperature of the collector. In addition, the system combined with heat pipe evacuated-tube solar collectors provided their net power output of 47.5, 50.2, 53.4, 59.6, 65.6, 71.3, 78.9, 85.8, and 92.2 MWh/Year, respectively and for flat-plate solar collectors provided 45.1, 45.6, 48.3, 51.0, 55.3, 60.1, 64.3, 69.4, and 72.6 MWh/Year, respectively.
Figure 10. Comparison of the net power output (MWh/Year) of the system, when the number of solar collectors varies from 300 to 700 units connected in parallel, the heat source temperature of 64°C, and three types of solar collectors (Location: Bangkok).
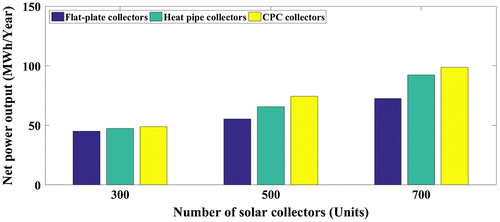
For average monthly net power output (kWh/Month) of the system with different types of the collector, it is shown in Figure . It shows that during daytime operations the highest electricity generation occurred in March and April or in the summer season (Summer: March to May, Rainy: June to October, and Winter: November to February). In addition, the result indicates that the system can generate the highest amount of electricity depending on: (1) rate of heat transfer from solar collectors, (2) ORC inlet hot water temperature or Collector outlet temperature, and (3) ambient temperature or the weather of each season.
Figure 11. Average monthly net power output (kWh/Month) of the system, when the number of solar collectors is 700 units connected in parallel, the heat source temperature of 60°C, and three types of solar collectors (Location: Bangkok, and Daytime operations).
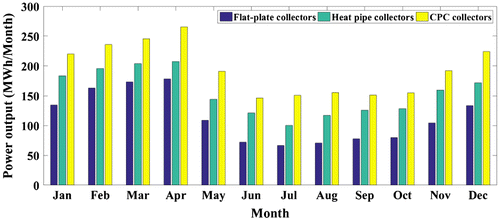
Figure shows the hourly ORC inlet hot water temperature (°C) of the system, when the number of solar collectors is 700 units connected in parallel and the heat source temperature is around 64°C. The results were found that the highest ORC inlet hot water temperature or collector outlet temperature can be produced when the system is combined with the CPC solar collectors. Although their efficiency can slightly decrease when the solar collector inlet temperature increases. These results are consistent with the highest power generation that the system could generate, when the system was combined with the CPC solar collectors.
4.4. Environment assessment
In this study, carbon dioxide intensity of electricity in Thailand was used to estimate CO2 emission. It refers to 0.548 kg CO2 eq./kWh (Energy Policy and Planning Office, Citation2015) for CO2 reduction of the system. This emission values were calculated from consumption of different fuel sources as Natural Gas (NG), Oil, and Coal/Lignite required for power generation in Thailand. Moreover the capability of reducing the CO2 emission of the system depends on the amount of the electricity the system can generate, and it can be calculated from Equation (12). Based on net power output of the system, it was found, that the tendency of CO2 reduction by the system increases when the number of solar collectors and the heat source temperature increase.
(12)
(12)
From Figure , it was shown that the CO2 reduction by the system has the upward trend when the number of solar collector increases. When the number of solar collectors varies from 300 to 700 units with 50 unit increments, the system with CPC collector can provide the lowest CO2 emissions of 26.8, 29.4, 33.0, 36.8, 40.8, 45.3, 49.3, 53.5, and 54.1 Ton CO2 eq./Year, respectively. In addition, the system with the heat pipe evacuated-tube solar collectors and the flat-plate solar collectors can decrease the CO2 emission of 26.0, 27.5, 29.3, 32.6, 35.9, 39.1, 43.2, 47, and 50.4 Ton CO2 eq./Year and 24.7, 25.0, 26.5, 28.0, 30.3, 33.0, 35.3, 38.0, 40.0 Ton CO2 eq./Year, respectively. Therefore, the VCHP-ORC power generation could assist the industrial sectors to reduce pollutants such as greenhouse gas emissions (GHG) and thermal pollution from the low-grade IWH that normally wasted and released into the environment.
4.5. Economic assessment
In this assessment, the investment of solar collectors was not included as mentioned in a previous Section 3. The LCOE was selected to represent the economic results of the VCHP-ORC power generation from low-grade IWH combined with SWHS. The results found that, the LCOE of the system had decreased when the number of solar collectors and the heat source temperature were increasing. The LCOE of the system with three different types of solar collectors was shown in Figure . The results showed that the system with 50 unit increments of the CPC solar collectors provided the lowest LCOE of 0.60, 0.55, 0.49, 0.44, 0.40, 0.36, 0.33, 0.30, and 0.29 USD/kWh, respectively. Moreover, the system with the heat pipe evacuated-tube solar collectors and the flat-plate solar collectors provide the LCOE of 0.62, 0.59, 0.55, 0.50, 0.45, 0.41, 0.37, 0.34, and 0.32 USD/kWh and 0.65, 0.64, 0.61, 0.58, 0.53, 0.49, 0.46, 0.43, and 0.41 USD/kWh, respectively. Furthermore, the net power output (MWh/Year), the CO2 reduction (Ton CO2 eq./Year), and the LCOE (USD/kWh) of the system are shown in Table , when the number of solar collectors of 700 units is connected in parallel and the heat source temperature increase from 60 to 70°C.
Figure 14. LCOE (USD/kWh) of the system, when the number of solar collectors varies from 300 to 700 units connected in parallel, and the heat source temperature of 64°C (Location: Bangkok).
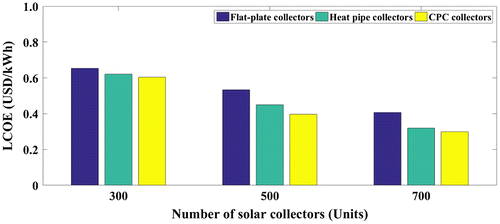
Table 5. Net power output (MWh/Year), CO2 Emission (Ton CO2 eq./Year), and LCOE (USD/kWh) of the system, when the number of solar collectors and the heat source temperature of 700 units connected in parallel, and 60 to 70°C, respectively (Location: Bangkok)
Therefore, it is noted that the VCHP-ORC power generation from a low-grade IWH combined with SWHS is an interesting system as the industries produce large amounts of low-grade wasted heat with temperatures above 62°C. In addition, a large number of solar collectors already installed can assist the industries in terms of the economic and the environmental impact. Moreover, from the Table , when the heat source temperature is above 66°C, it was found that the system with CPC solar collectors produced the lower net power output as compared with that of the heat pipe evacuated-tube solar collectors due to the ORC inlet temperature or collector outlet temperature over the temperature limit of the ORC power generation (for this study 95°C). In other word the ORC system cannot generate electricity.
4.6. System evaluation in difference areas
The evaluation results of the system at different locations were compared. When the number of solar collectors is 700 units connected in parallel with the heat source temperature of 64°C as shown in Table . It was found that in Chiang Mai province, as a representative of the north part of Thailand, generated the highest electricity because of the highest annual solar energy collected. With the same number of solar collectors Chiang Mai can produce the maximum thermal energy required for ORC power generation. In addition, the average maximum and minimum ambient temperatures of this province were the lowest compared to other areas (the results are consistent with previous studies of Thawonngamyingsakul and Kiatsiriroat (Citation2012), followed by Nakhon Ratchasima, Chonburi, Bangkok, Ratchaburi, and Songkhla, respectively.
Table 6. comparison of the net power output (MWh/Year), the CO2 Emission (Ton CO2 eq./Year), and the LCOE (USD/kWh) of the system of six areas, when the number of solar collectors of 700 units connected in parallel, and the heat source temperature was at around 64°C.
From the evaluation results above, the weather data was only taken into account. However, these results can help the government to make a decision whether to support an industry based on the quality and quantity of low-grade waste heat, type and number of solar collectors, and the number of factories in the estate. Therefore, the larger number of factories in an industrial estate is, the more quantity of low-grade waste heat is available. Thus, it makes the system a good candidate for power generation from low heat waste. In this study, Bangkok province presented the highest number of factories, follow by Nakhon Ratchasima, Chonburi, Chiang Mai, Songkhla, and Ratchaburi, respectively (Department of Industrial Works, Citation2017). Moreover, LCOE of each location is shown in Table . the VCHP-ORC power generation from a low-grade IWH combined with SWHS has a LCOE between 0.25 and 0.50 USD/kWh, while CSP technologies have a LCOE between 0.20 and 0.35 USD/kWh, and the solar photovoltaic has a LCOE between 0.14 and 0.47 USD/kWh (International Renewable Energy Agency, Citation2015). Therefore, the VCHP-ORC system is the most interesting.
5. Conclusion
In this research, a concept for a VCHP-ORC power generation from a low-grade IWH with temperature below 70°C combined with SWHS was proposed and investigated. Three types of solar collectors consisting of flat-plate, heat pipe evacuated-tube, and compound parabolic concentrator (CPC) solar collectors were compared in terms of heat generation to feed the system. Six areas of industrial estate consisting of Chiang Mai (18.80°N, 98.98°E), Bangkok (13.75°N, 100.52°E), Ratchaburi (13.54°N, 99.82°E), Songkhla (7.21°N, 100.56°E), Nakhon Ratchasima (13.75°N, 100.52°E), and Chon Buri (13.40°N, 101.00°E) that represent the north, central, west, south, north-east and east part of Thailand were taken as input weather data for the simulations. The system was mathematically modeled and simulated to evaluate the net power output, the environmental impact, and the LCOE of the system. The main conclusions can be summarized as follows:
• | The VCHP-ORC power system is applicable for the below 70°C heat source from low-grade IWH combined with SWHS. Moreover, it is a technology solution for power generation from low-grade industrial waste heat, which is generally wasted and released into the environment. | ||||
• | This technology can help the industrial sector to reduce the energy intensity, increase the energy efficiency of the industrial processes, as well to reduce the pollution (greenhouse gas emissions (GHG), and thermal pollution). | ||||
• | Type and number of solar collectors, quality and quantity of low-grade IWH, and location and number of industries in industrial estates have an effect on the system in terms of the net power output, the environmental impact, and the LCOE of the system. | ||||
• | Chiang Mai province as a representative of the north part of Thailand provided the highest net power output and the lowest LCOE of the system due to the highest annual solar energy and the lowest average maximum and minimum ambient temperature compared to other areas. For instance, when the temperature of the heat source was around 64°C with 700 solar collectors units connected in parallel; the system combined with flat-plate, heat pipe evacuated-tube, and CPC solar collectors can produce 84.4, 107.0, and 117.1 MWh/Year with a LCOE of 0.35, 0.28, and 0.25 USD/kWh, respectively. In terms of the environmental impact, the system can reduce the CO2 emissions by 46.2, 58.6, and 64.2 Ton CO2 eq./Year, respectively. In terms of the economics analysis, the LCOE of the VCHP-ORC system with the CSP technologies is in the LCOE range of the solar photovoltaic technology which makes it much interesting. |
For future work, since the proposed system still consumed some electrical power to operate the VCHP. It would be interesting to develop a power generation system to only consume the heat such as an absorption heat transformer (AHT) using the thermal energy to upgrade the heat either from low-grade IWH or SWHS before supplying to the ORC power generation. This could lead to a better understanding and improvement of this system and also allow it to use other renewable sources such as geothermal and biomass.
Nomenclature | ||
A | = | Area (m2) |
cp | = | Specific heat (kJ/kg − K) |
CInvest | = | Investment cost (USD) |
CO\M | = | Operation and Maintenance cost (USD/Year) |
h | = | Enthalpy (kJ/kg) |
IT | = | Total solar radiation on the tilted surface (W/m2) |
= | Mass rate (kg/s) | |
= | Heat transfer rate (kWth) | |
T | = | Temperature (°C) |
= | Power (kWe) |
Subscript | ||
Amb | = | Ambient |
Coll | = | Solar collectors |
Comp | = | Compressor |
Cond | = | Condenser |
el | = | Electric energy |
Evap | = | Evaporator |
i | = | Inlet |
ME | = | Mechanical |
MO | = | Motor |
o | = | Outlet |
r | = | Refrigeratn or Subtance |
s | = | Isentropic process |
th | = | Thermal energy |
w | = | Water |
1–4 | = | Position in the cycle |
Funding
The authors would like to thank to the Naresuan University for revenue budget in 2017 for this project.
Acknowledgements
The authors would like to thank to the Interdisciplinary Graduate School of Science and Technology, Faculty of Engineering, Shinshu University, Japan, and Energy Research and Promotion Center, Faculty of Science, Naresuan University, Thailand for supporting.
Additional information
Notes on contributors
Sorawit Sonsaree
In 2006, Sorawit Sonsaree graduated from Faculty of Engineering, Naresuan University, Phitsanulok, Thailand with Bachelor degree in Mechanical Engineering. After his first degree, he had worked with Dutch Mill Company in Thailand as Engineering Supervisor to maintain all building facility for one year, and then he shifted his interest toward academic field. Therefore, he was accepted to work a research assistant from 2007 to 2013 at the School of Renewable Energy Technology (SERT), Naresuan University. During his working period, he involved in several projects relative to renewable energy and energy conservation. Moreover, he received the scholarship from the university to continue for his master degree in Energy Engineering, Chiang Mai University, Chiang Mai Thailand, and graduated in 2011. Currently, he is working towards the PhD degree at the Interdisciplinary Graduate School of Science and Technology, Shinshu University, Nagano, Japan. His research interests include renewable energy technologies, specially, solar thermal technology.
References
- Amin, Z. M., & Hawlader, M. N. A. (2013, October). A review on solar assisted heat pump systems in Singapore. Renewable and Sustainable Energy Reviews, 26, 286–293.10.1016/j.rser.2013.05.032
- Arvay, P., Muller, M. R., Ramdeen, V., & Cunningham, G. (2011). Economic implementation of the organic Rankine cycle in industry. In ACEEE Summer Study on Energy Efficiency in Industry (pp. 12–22), New York, NY.
- Atmospheric Science Data Center. (2017). NASA surface meteorology and solar energy - location. Retrieved January 26, 2017, from https://eosweb.larc.nasa.gov/cgi-bin/sse/[email protected]
- Aye, L., Charters, W. W. S., & Chaichana, C. (2002, September). Solar heat pump systems for domestic hot water. Solar Energy, 73, 169–175.10.1016/S0038-092X(02)00043-9
- Banister, C. J., & Collins, M. R. (2015, July). Development and performance of a dual tank solar-assisted heat pump system. Applied Energy, 149, 125–132.10.1016/j.apenergy.2015.03.130
- Bao, J. J., Zhao, L., & Zhang, W. Z. (2011, November). A novel auto-cascade low-temperature solar Rankine cycle system for power generation. Solar Energy, 85, 2710–2719.
- Baral, S., & Kim, K. C. (2014, September). Thermodynamic modeling of the solar organic Rankine cycle with selected organic working fluids for cogeneration. Distributed Generation & Alternative Energy Journal, 29, 7–34.10.1080/21563306.2014.10879015
- Bocci, E., Villarini, M., Vecchione, L., Sbordone, D., Di Carlo, A., & Dell’Era, A. (2015). Energy and economic analysis of a residential solar organic Rankine plant. Energy Procedia, 81, 558–568.10.1016/j.egypro.2015.12.135
- BP energy Outlook 2016 Edition. (2016). Retrieved January 26, 2017, from https://www.bp.com/content/dam/bp/pdf/energy-economics/energy-outlook-2016/bp-energy-outlook-2016.pdf
- BP Energy Outlook 2017 Edition. (2017). Retrieved January 26, 2017, from https://www.bp.com/content/dam/bp/pdf/energy-economics/energy-outlook-2017/bp-energy-outlook-2017.pdf
- BSC Incorporated. (2008, March). Waste heat recovery: Technology and opportunities in U.S. industry. Washington, DC: U.S. Department of Energy, Industrial Technologies Program.
- Calise, F., d’Accadia, M. D., Vicidomini, M., & Scarpellino, M. (2015, January). Design and simulation of a prototype of a small-scale solar CHP system based on evacuated flat-plate solar collectors and Organic Rankine Cycle. Energy Conversion and Management, 90, 347–363.10.1016/j.enconman.2014.11.014
- Campana, F., Bianchi, M., Branchini, L., De Pascale, A., Peretto, A., Baresi, M., … Vescovo, R. (2013, December). ORC waste heat recovery in European energy intensive industries: Energy and GHG savings. Energy Conversion and Management, 76, 244–252.10.1016/j.enconman.2013.07.041
- Chaichana, C., Kiatsiriroat, T., & Nuntaphan, A. (2010). Comparison of conventional flat-plate solar collector and solar boosted heat pump using unglazed collector for hot water production in small slaughterhouse. Heat Transfer Engineering, 31, 419–429.10.1080/01457630903375475
- Chaiyat, N. (2014, November). Upgrading of low temperature heat with absorption heat transformer for generating electricity by organic Rankine cycle. Global Advanced Research Journal of Engineering, Technology and Innovation, 3, 235–247.
- Chaiyat, N. (2015a, February–March). Assessment alternative energy for organic Rankine cycle power plant in Thailand. International Journal of Engineering and Technology (IJET), 7, 317–326.
- Chaiyat, N. (2015b). Sustainability of alternative energy for organic rankine cycle power plant in Thailand. Naresuan University Journal: Science and Technology, 23, 45–62.
- Chaiyat, N., & Kiatsiriroat, T. (2015, November). Analysis of combined cooling heating and power generation from organic Rankine cycle and absorption system. Energy, 91, 363–370.10.1016/j.energy.2015.08.057
- Delgado-Torres, A. M., & García-Rodríguez, L. (2010). Analysis and optimization of the low-temperature solar organic Rankine cycle (ORC). Energy Conversion and Management, 51, 2846–2856.10.1016/j.enconman.2010.06.022
- Department of Alternative Energy Development and Efficiency. (2006). Potentials of concentrating solar power technologies in Thailand. Thailand: Ministry of Energy.
- Department of Alternative Energy Development and Efficiency. (2017). Thailand alternative energy situation 2015. Retrieved January 26, 2017, from https://www.dede.go.th/download/state_59/Thailand%20alternative%20energy%202015.pdf
- Department of Alternative Energy Development and Efficiency (2017). Solar hot water. Thailand: Ministry of Energy. Retrieved from https://www.solarhotwaterdede.com/en/
- Department of Industrial Works. (2017). Retrieved February 24, 2017, from https://www.diw.go.th/hawk/content.php?mode=spss59
- Domínguez Bravo, J., García Casals, X., & Pinedo Pascua, I. (2007, October). GIS approach to the definition of capacity and generation ceilings of renewable energy technologies. Energy Policy, 35, 4879–4892.10.1016/j.enpol.2007.04.025
- Duffie, J. A., & Beckman, W. A. (2013). Solar engineering of thermal processes. Hoboken, NJ: John Wiley & Sons.10.1002/9781118671603
- Energy Policy and Planning Office. (2015). Energy statistics of Thailand 2015. Thailand: Ministry of Energy.
- Energy Policy and Planning Office. (2017). Alternative energy development plan: AEDP2015. Ministry of Energy. Retrieved January 26, 2017, from https://www.eppo.go.th/images/POLICY/ENG/AEDP2015ENG.pdf
- Fan, X.-W., Zhang, X.-P., Ju, F-J., & Wang, F. (2013). Theoretical study of heat pump system using CO2/dimethylether as refrigerant. Thermal Science, 17, 1261–1268.10.2298/TSCI1305261F
- Freeman, J., Hellgardt, K., & Markides, C. N. (2015, January). An assessment of solar-powered organic Rankine cycle systems for combined heating and power in UK domestic applications. Applied Energy, 138, 605–620.10.1016/j.apenergy.2014.10.035
- Garg, P., Orosz, M. S., & Kumar, P. (2016, October). Thermo-economic evaluation of ORCs for various working fluids. Applied Thermal Engineering, 109, 841–853.10.1016/j.applthermaleng.2016.06.083
- Guillen, D. P., & Zia, J. (2013). Final report: Modifications and optimization of the organic Rankine cycle to improve the recovery of waste heat. Idaho Falls, ID: Idaho National Laboratory.10.2172/1116747
- Hepbasli, A., & Kalinci, Y. (2009, August-September). A review of heat pump water heating systems. Renewable and Sustainable Energy Reviews, 13, 1211–1229.10.1016/j.rser.2008.08.002
- Higgo, A. R., & Zhang, T. J. (2015, May). Characterization of a compact organic Rankine cycle prototype for low-grade transient solar energy conversion. Energy Procedia, 69, 1113–1122.10.1016/j.egypro.2015.03.223
- Huang, F., Zheng, J., Baleynaud, J. M., & Lu, J. (2016). Heat recovery potentials and technologies in industrial zones. Journal of Energy Institute, 1–11.
- Ibrahim, O., Fardoun, F., Younes, R., & Louahlia-Gualous, H. (2014). Air source heat pump water heater: Dynamic modeling, optimal energy management and mini-tubes condensers. Energy, 64, 1102–1116.10.1016/j.energy.2013.11.017
- International Energy Agency. (2017). Renewables for Power Generation Status & Prospects 2003 Edition. Retrieved January 26, 2017, from https://www.antoniolima.web.br.com/arquivos/renewpower_2003.pdf
- International Renewable Energy Agency. (2015). Renewable power generation costs in 2014. Bonn: Author.
- Jung, H.-C., Taylor, L., & Krumdieck, S. (2015, March). An experimental and modelling study of a 1 kW organic Rankine cycle unit with mixture working fluid. Energy, 81, 601–614.10.1016/j.energy.2015.01.003
- Kobelco. (2017). Retrieved January 26, 2017, from https://www.kobelco.co.jp/products/standard_compressors/microbinary/files/microbinary_catalog.pdf
- Li, P., Li, J., Pei, G., Munir, A., & Ji, J. (2016, April). A cascade organic Rankine cycle power generation system using hybrid solar energy and liquefied natural gas. Solar Energy, 127, 136–146
- Liu, H., Zhou, Q., Zhao, H., & Wang, P. (2015, January). Experiments and thermal modeling on hybrid energy supply system of gas engine heat pumps and organic Rankine cycle. Energy and Buildings, 87, 226–232.10.1016/j.enbuild.2014.11.046
- National Institute of Standard and Technology. (2000). REFPROP version 7, thermodynamic properties of refrigerants and refrigerant mixtures software.
- Pei, G., Li, J., & Ji, J. (2010, June). Analysis of low temperature solar thermal electric generation using regenerative organic Rankine cycle. Applied Thermal Engineering, 30, 998–1004.10.1016/j.applthermaleng.2010.01.011
- Pikra, G., Rohmah, N., Pramana, R. I., & Purwanto, A. J. (2015, April). The electricity power potency estimation from hot spring in Indonesia with temperature 70–80°C using organic Rankine cycle. Energy Procedia, 68, 12–21.10.1016/j.egypro.2015.03.227
- Pitz-Paal, R., Dersch, J., & Milow, B. (2003). European concentrated solar thermal road-mapping. Co-funded: European Commission.
- Purohit, I., & Purohit, P. (2010, June). Techno-economic evaluation of concentrating solar power generation in India. Energy Policy, 38, 3015–3029.10.1016/j.enpol.2010.01.041
- Quoilin, S., & Lemort, V. (2009, April). Technological and Economical Survey of Organic Rankine Cycle Systems. In 5th European Conference Economics and Management of Energy In Industry.
- Quoiln, S., Van Den Broek, M., Sébastien, D., Dewallef, P., & Lemort, V. (2013, June). Techno-economic survey of organic Rankine cycle (ORC) systems. Renewable and Sustainable Energy Reviews, 22, 168–186.10.1016/j.rser.2013.01.028
- Rahbar, K., Mahmoud, S., Al-Dadah, R. K., Moazami, N., & Mirhadizadeh, S. A. (2017, February). Review of organic Rankine cycle for small-scale applications. Energy Conversion and Management, 134, 135–155.10.1016/j.enconman.2016.12.023
- Rowshanzadeh, R. (2011). Performance and cost evaluation of organic Rankine cycle at different technologies (Sustainable Energy Engineering Master Student). Department Of Energy Technology, KTH Royal Institute of Technology, Sweden.
- Senechal, S. (2017). Turboden ORC: Recent developments and new applications in organic Rankine cycle technology. Retrieved February 2, 2017, from https://www.all-energy.co.uk/__novadocuments/54368?v=635376693736400000
- Solarbayer. (2017). Compound parabolic concentrating (CPC) solar collectors. Retrieved January 26, 2017, from https://www.solarbayer.com/Vacuum-tube-collector-CPC.html
- Sonsaree, S., Asaoka, T., Jiajitsawat, S., Aguirre, H., & Tanaka, K. (2016a). Application of low-grade industrial waste heat for power generation using organic Rankine cycle power generator combined with gas engine-driven heat pump. In The Asian Conference on Sustainability, Energy & the Environment 2016 (pp. 443–451), Kobe
- Sonsaree, S., Asaoka, T., Jiajitsawat, S., Aguirre, H., & Tanaka, K. (2016b). Organic Rankine Cycle Power Generation from Industrial Waste Heat Recovery Integrated with Solar Hot Water System by using Vapor Compression Heat Pump as Heating Booster in Thailand. In International Conference on Cogeneration, Small Power Plants and District Energy (pp. 1–6), Bangkok
- Soroka, B. (2015). Application note - industrial heat pumps. Brussels: European Copper Institute Copper Alliance.
- SPF Institute for Solar Technology. (2017a). Flat-plate solar collectors. Retrieved January 26, 2017, from https://www.spf.ch/fileadmin/daten/reportInterface/kollektoren/factsheets/scf1623en.pdf
- SPF Institute for Solar Technology. (2017b). Heat pipe evacuated-tube solar collectors. Retrieved January 26, 2017, from https://www.spf.ch/fileadmin/daten/reportInterface/kollektoren/factsheets/scf1655en.pdf
- Statistical Review of World Energy. (2017). Retrieved January 26, 2017, from https://www.bp.com/en/global/corporate/energy-economics/statistical-review-of-world-energy.html
- Tchanche, B. F., Lambrinos, G., Frangoudakis, A., & Papadakis, G. (2011, October). Low-grade heat conversion into power using organic Rankine cycles - A review of various applications. Renewable and Sustainable Energy Reviews, 15, 3963–3979.10.1016/j.rser.2011.07.024
- Tchanche, B. F., Pétrissans, M., & Papadakis, G. (2014, November). Heat resources and organic Rankine cycle machines. Renewable and Sustainable Energy Reviews, 39, 1185–1199.10.1016/j.rser.2014.07.139
- Thawonngamyingsakul, C., & Kiatsiriroat, T. (2012). Potential of a solar organic rankine cycle with evacuated-tube solar collectors as heat source for power generation in Thailand. Energy Science and Technology, 4, 25–35.
- U.S. Department of Energy’s Industrial Technologies Program. (2004, December). Energy use, loss and opportunities analysis: U.S. manufacturing and mining. Washington, DC: Author.
- Van de Bor, D. M., Infante Ferreira, C. A., & Kiss, A. A. (2015, September). Low grade waste heat recovery using heat pumps and power cycles. Energy, 89, 864–873.10.1016/j.energy.2015.06.030
- Vélez, F., Segovia, J. J., Carmen Martin, M., Antolin, G., Chejne, F., & Quijano, A. (2012, August). A technical, economical and market review of organic Rankine cycles for the conversion of low-grade heat for power generation. Renewable and Sustainable Energy Reviews, 16, 4175–4189.10.1016/j.rser.2012.03.022
- Viswanathan, V. V., Davies, R. W., & Holbery, J. (2006, April). Opportunity analysis for recovering energy from industrial waste heat and emission. Washington, DC: Pacific Northwest National Laboratory Operated by Battelle for the U.S. Department of Energy.10.2172/1218710
- Zhang, X. R., Yamaguchi, H., Uneno, D., Fujima, K., Enomoto, M., & Sawada, N. (2006). Analysis of a novel solar energy-powered Rankine cycle for combined power and heat generation using supercritical carbon dioxide. Renewable Energy, 31, 1839–1854.10.1016/j.renene.2005.09.024
- Zhang, J., Zhao, L., Deng, S., Xu, W., & Zhang, Y. (2017). A critical review of the models used to estimate solar radiation. Renewable and Sustainable Energy Reviews, 70, 314–329.10.1016/j.rser.2016.11.124
Appendix A
The total solar radiation for a day on the tilted surface and an average maximum-minimum ambient temperature at Chiang Mai, Bangkok, Ratchaburi, Songkhla, Nakhon Ratchasima, and Chonburi as shown in Table and Figure , and Table , respectively.
Table A1. The total solar radiation for a day on the tilted surface at each location
Figure A1. The total solar radiation for a day on the tilted surface at each location (Atmospheric Science Data Center, Citation2017).
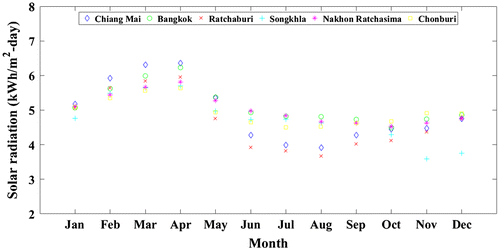