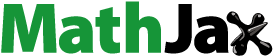
Abstract
Various research works have been carried out on cement and lime stabilization of lateritic soil. The production of cement is too expensive and environmentally unfriendly due to the required huge embodied energy as well as the environmental pollution resulting in ozone layer depletion. In order to minimize these shortcomings, researchers are exploring a sustainable alternative to cement. There is limited scholarly work done on the use of a combination of agro-waste ashes for the stabilization of lateritic soil. This study is aimed at examining the performance of lateritic soil stabilized with a combination of bone ash (BA) and palm bunch ash (PBA) for sustainable building applications. Different cube sizes (50 mm3, 70 mm3, and 100 mm3) were used to produce lateritic bricks of different matrices which were used to obtain the compressive strength test results. Addition of a minimal percentage of BA (2%) and PBA (2%) improved the compressive strength of lateritic bricks by 129% compared to the natural lateritic soil. Brick specimen produced with the smallest mould size (2500 mm2) gave higher compressive strength compared to larger mould size. The findings of this study confirm the potential of using lateritic soil stabilized with a minimal percentage of BA (2%) and PBA (2%) for sustainable building applications.
PUBLIC INTEREST STATEMENT
The production of cement is too expensive and environmentally unfriendly due to the required huge embodied energy as well as the environmental pollution resulting in ozone layer depletion. In order to minimize these shortcomings, researchers are exploring a sustainable alternative to cement. This study explores the use of agro-waste ash to stabilize lateritic soil for sustainable building applications. The addition of a combination of 4% stabilizers (BA and PBA) improved the compressive strength of lateritic soil by 129%. Also, the use of PBA for a partial replacement for BA reduces the embodied energy consumed in the production of BA since the PBA takes lesser embodied energy to produce. The findings of this study confirm the potential of using lateritic soil stabilized with a minimal percentage of BA (2%) and PBA (2%) for sustainable building applications.
1. Introduction
Affordable building for mass housing is the panacea for the housing deficit affecting the increasing population of the African continent especially Nigeria. Harnessing the abundant local building materials present in Africa is key for making adequate houses affordable for the populace. Lateritic soil is an earth-based building material (Jayasinghe & Kamaladasa, Citation2006) that is among the topmost available local building materials abundantly available in Nigeria and other tropical countries (Oluremi et al., Citation2012). Earth-based building materials are cheaper than cement and eco-friendly (Alam et al., Citation2015). Earth-based building materials are cheaper than cement because of the high embodied energy used for cement production. Unlike the cement, earth-based materials production do not release greenhouse gases to the environment and this makes them non-toxic and eco-friendly. The lateritic soil is composed mainly of quartz, iron–magnesium–manganese (amphibole group), and kaolinite (Egenti & Khatib, Citation2016). Soils having a ratio of silica to sesquioxide [SiO2/(Fe2O3 + Al2 O3)] which are less than 1.33 are classified as laterites whereas the ones between 1.33 and 2.00 are classified as lateritic soil, and those above 2.00 are classified as non-lateritic soils (Nnochiri & Adetayo, Citation2019). Lateritic soil can easily be recycled and that makes it a sustainable building material (Onakunle et al., Citation2020). However, due to their lower compressive strength, water absorption, and dimensional stability compared to cementitious materials, lateritic blocks are considered as non-durable building materials. A wide range of chemical stabilization techniques such as the use of cement, lime, agro-waste ash, and plastic waste has been investigated to improve the physical, chemical, and mechanical properties of earth-based materials (Apampa, Citation2017; Fadele & Ata, Citation2018; Hamada et al., Citation2020; Jamil et al., Citation2013; Moraes et al., Citation2019; Onyelowe, Citation2017a, Citation2017b; Rimal et al., Citation2019). The properties improved include compressive strength, water absorption, durability, ultrasonic pulse velocity, dry shrinkage, flexural strength, splitting tensile strength and workability.
Stabilization of earth materials has been employed in the construction industry in order to improve the strength of structures constructed with these materials (Oluwatuyi et al., Citation2018). The effectiveness of using lime for the stabilization of lateritic soil had been established by various researchers (Ajayi, Citation2012; Akoto & Singh, Citation1981; Alavéz-Ramírez et al., Citation2012; Amadi & Okeiyi, Citation2017; Dang et al., Citation2016; Malkanthi et al., Citation2020; Obianyo, Onwualu et al., Citation2020; Ola, Citation2013; Rao & Shivananda, Citation2005). However, hydrated lime is more expensive than cement in Nigeria, as the cost of 50 Kg of cement can only purchase 12.5 kg of hydrated lime (Joel & Edeh, Citation2013). Currently, the average cost of 50 kg of cement and 50 kg of hydrated lime in Nigeria are approximately 7.5 and 525 dollars, respectively. This high cost of hydrated lime has made it a non-viable material for the construction of sustainable buildings; hence, the need to seek an alternative to lime for stabilization of soil. Agro-waste ash is a promising alternative to lime as agro–wastes are produced in hundreds of metric tonnes yearly in Nigeria (Sadh et al., Citation2018). The presence of oxides of calcium makes agro-waste ash a viable material for lime replacement. This is because the major constituent of lime is oxide/hydroxide of calcium. The utilization of agro-waste for soil stabilization will result in value addition to the agro-wastes as well as cleaning of the environment from pollution caused by the agro-wastes.
The valorization of agro-wastes for application in the field of civil engineering is a profitable alternative to cement for sustainable construction applications and has been investigated by various researchers (Arancon et al., Citation2013; García-Díaz et al., Citation2016; Madurwar et al., Citation2013). Previous work done on lateritic soils indicated the effective stabilization of lateritic soil using bone ash in place of hydrated lime (Obianyo, Onwualu et al., Citation2020). However, the embodied energy required for the production of the bone ash is still an issue of concern. This is because a temperature of about 650°C is required to calcine the bone ash after open air burning and the cost of such energy is high despite the benefit of reducing the environmental pollution caused by these wastes. Hence, the need to find alternative agro-waste ash for possible partial replacement of bone ash for lateritic soil stabilization. Oil palm bunch is abundantly available in Nigeria and it is usually discarded as waste after removal of the palm fruit. The estimated value of palm bunch in Nigeria could be put at zero since it is dumped as waste. Besides, the production of palm bunch ash requires considerably less embodied energy than bone ash. While bone ash is produced at a temperature of about 650°C, palm bunch ash is produced at a temperature of 300°C. Based on the temperatures and time taking to produce both materials, the cost of producing palm bunch ash is lower than that of bone ash. This is because the higher the temperature used for the production of materials, the higher the cost of energy and the release of CO2 into the atmosphere is increased. Udoetok (Citation2012) characterized palm bunch ash and found out that it contains metals such as chromium (0.088 mg/kg), zinc (0.38 mg/kg), calcium (146.15 mg/kg), potassium (139.35 mg/kg), sodium (0.63 mg/kg), and magnesium (1.68 mg/kg). It means that palm bunch ash contains calcium and the presence of calcium is required for the formation of cementitious materials (calcium silicate hydrate and calcium aluminate hydrate) which will probably result in improved strength of materials stabilized with it. The impact and applications of palm bunch for the production of building materials have been investigated by some researchers (Omoniyi, Citation2019; Otunyo & Chukuigwe, Citation2018; Series & Science, Citation2019). This study investigates the effective utilization and stabilization of lateritic soils using agro-waste ash (bone ash and palm bunch ash) for sustainable and affordable construction applications. The effects of curing temperature, curing age, water content, the weight percentage of stabilizers (BA and PBA), and size effect of the samples on the compressive strength of lateritic bricks were also explored in this work. The understanding of how these factors influence the compressive strength of stabilized lateritic soil will enhance the production of an improved and more durable lateritic bricks for sustainable buildings.
2. Materials and methods
2.1. Materials
The lateritic soil used in this study was obtained from Galadimawa, Airport Road, Abuja, Nigeria. The cattle bones used for the production of BA were sourced from Gosa Market, Airport Road, Abuja whereas the palm bunches used for the production of the PBA were obtained from Oba, Anambra State, Nigeria. The chemical composition and particle size distribution of the lateritic soil used are presented in and respectively. The ratio of silica to sesquioxide [SiO2/(Fe2O3 + Al2O3)] for the soil sample used for the study is 1.546. This ratio of silica to sesquioxide falls between 1.33 and 2.00 and hence it is lateritic soil. From XRF results of , it can be seen that the summation of the SiO2, Al2O3, and Fe2O3 is 81.361%. ASTM C618 (Pourkhorshidi et al., Citation2010) classified pozzolanic material as material that has the summation of the SiO2, Al2O3, and Fe2O3 up to 70%. Hence, the lateritic soil sample used for the study is pozzolanic which implies that it possesses little or no cementitious value but in finely divided form and the presence of water will react chemically with calcium hydroxide at ordinary temperature to form compounds having cementitious properties (Obianyo, Onwualu et al., Citation2020). In other words, lateritic soil, being a pozzolanic material contains binding properties that can react with calcium hydroxide to form cementitious material and thus making it a good replacement for cement. The particle size distribution curve observed in represents a well-graded soil that contains silt predominantly, according to BS 1377: Part 2: 1990: 4.3 classification. The Atterberg limits test results indicated that the lateritic soil sample possesses the following properties: liquid limits (41%); plastic limit (17.75%); plastic index (23.25%); linear shrinkage (13.63%); and average water content (17.75%).
Table 1. Chemical composition of the lateritic soil sample
2.2. Production of bone ash and palm bunch ash
Cattle bones obtained from meat vendors were washed and sun-dried for two weeks after which they were burnt in a furnace at a temperature of 650°C. The burnt cattle bones were ground with a Jaw Crusher and then passed through a 75 μm mesh sieve to obtain a fine particle of BA as shown in ). The collected palm bunches were sun-dried for 1 week after which they were burnt in the open air and allowed to cool before passing the resulting PBA through a 75 μm mesh sieve to obtain a fine particle of PBA as presented in ). The chemical compositions of BA and PBA analyzed using Thermo Scientific X-ray Fluorescence (XRF) Epsilon Spectrometer are shown in respectively. XRF analysis was done using the standard method with Montana soil SRM 2710 as a Thermo Fisher Scientific standard reference material. The result of the chemical composition analysis indicates the presence of oxide of calcium in both BA and PBA needed for the pozzolanic reaction with the lateritic soil is present both in the BA and PBA. The main oxides present in Portland cement which include CaO, SiO2, Al2O3, Fe2O3, MgO, and K2O were also present in BA and PBA as shown in the chemical composition of the BA and PBA represented in . BA used for the study contains 52.20% of CaO and this aligns with the results of other researchers that reported 55.30% (Gouvêa et al., Citation2015); 63.86% (Okeyinka et al., Citation2018); and 54.14% (Ifka et al., Citation2012) as the percentages of CaO in BA. The presence of P2O5 (less than 1%) in the BA has the ability of improving the hydraulic properties of di-calcium silicate (C2S) by entering into it and could also increase setting times of the stabilized bricks. However, higher amount of P2O5 inhibits the reaction of C2S with C to form tri-calcium silicate (C3S) in the stabilized soil. Thus, high P2O5 enters the clinker minerals {C2S, C3S and C3A (tri-calcium aluminate)} to form solid solutions which affect the phase composition of the stabilized soil matrix (Ifka et al., Citation2012). Soil stabilization can be achieved by the pozzolanic reaction between the CaO present in the stabilizers and the soil particles (Obianyo, Onwualu et al., Citation2020). When BA and PBA are added to the lateritic soil and mixed with water, the Ca (OH)2 disassociates into Ca2+ and OH− ions. These ions cause the silica and alumina contained in the lateritic soil to dissolve and combine with Ca2+ ions to form calcium-silicate-hydrates (C-S-H) and calcium-aluminate-hydrates (C-A-H) which are the main product of the hydration of Portland cement that is responsible for the strength in cement-based materials (Muntohar, Citation2011; Pacheco-Torgal & Jalali, Citation2011). The amorphous gel (C-S-H and C-A-H) bind the soil matrix and it is principally responsible for the increase in strength.
Table 2. Chemical composition of the palm bunch ash
Table 3. Chemical composition of the bone ash (Obianyo, Onwualu et al., Citation2020)
2.3. Materials characterization
Characterization of the soil sample and the agro-waste ash using specific tests will aid the proper understanding of the properties of the materials as well as their mechanical behaviours. Nicolet is5 Thermo Scientific FTIR spectrometer was used in the characterization of the powdered samples of lateritic soil, BA, and PBA. The mineralogical compositions of the laterite samples used for this research were analyzed using Rigaku Miniflex 600 XRD machine with range 10–70° at a rate of 2°/min and Cu K alpha radiation (Obianyo, Onwualu et al., Citation2020). Energy Dispersive X-ray Spectroscopy (EDS) maps and scanning electron micrographs were obtained with the use of the Evo/LS10 ZEISS Scanning Electron Microscope (SEM).
2.4. Sample preparation and research design
Samples of BA and PBA were used as soil stabilizers to improve the strength of lateritic soil obtained from Galadimawa in Abuja, Nigeria. The soil samples were obtained from a depth of 1.5 m to 2.0 m below the ground surface using the method of disturbed sampling. The soil sample was air-dried at room temperature for 2 weeks at the AfDB laboratory at the African University of Science and Technology and was ground to fine particles. The cattle bones were washed and dried for 2 weeks. The BA was obtained by calcination of cattle bones at 650°C in an electric furnace. The choice of the calcination temperature is because at 600°C, the organic component of the bones would be removed and carbonate apatite would be obtained, and this resulted in the production of oxides of carbon at temperatures of 650°C and above (Figueiredo et al., Citation2010). Palm bunch was dried thoroughly under the sun for 1 week, cut into smaller sizes, and burnt for about an hour using open-air burning. The resulting BA and PBA were passed through No. 200 sieve (75 μm) before usage to obtain finer particles.
Preliminary laboratory tests on soil sample for the purpose of characterization, which include natural moisture content, particle size distribution, and Atterberg limits were carried out in order to understand the properties and behaviour of the soil sample used. The BA and PBA were added to the soil sample in equal proportion at a varying proportion of 0%, 2%, 4%, and 6% by weight of the soil and mixed thoroughly before adding a calculated amount of water. Different water contents of 18%, 24%, and 30% based on the Atterberg limits test results were used in the mix design to determine the effects of water content on the mechanical properties of the stabilized bricks. The homogenized mixture of the raw materials (lateritic soil, BA, PBA, and water) was used to mould lateritic brick samples of different material matrices and compositions. The lateritic bricks were obtained with the aid of a locally made mould of 50 × 50 × 50 mm3; 70 × 70 × 70 mm3; and 100 × 100 × 100 mm3 sizes which were constructed to correspond to the shape and sizes of the samples to be made. The freshly moulded lateritic bricks were carefully extruded in good shape after 24 hours on a clean, hard, and flat surface to prevent the development of cracks. The moulded samples were cured for 7 days, 14 days, and 28 days before testing. Also, three different temperatures that were used to cure the brick samples in this study are 23 ± 2°C(air-drying), 37 ± 2°C (sun-drying), and 105°C (oven-drying). The brick samples of different sizes are presented in . Compressive strength test was carried out on the lateritic bricks to measure the mechanical behaviour of agro-waste stabilized lateritic bricks.
2.5. Compressive strength of lateritic bricks
The compressive strengths of the lateritic bricks were determined in accordance with EN 206 (EN 206: Citation2013, Citation2013) using Model 70-S12V02 Universal Testing Machine Controls. Load measurements were taken during testing at a displacement rate of 0.02 mm/s and a loading rate of 24 kN/s until lateritic brick failure occurred as shown in . EquationEquation 1(1)
(1) was used to calculate the maximum compressive stress in the lateritic bricks at failure:
Where σ = calculated normal stress (MPa); P = measured applied load (N) and A = net area of the surface on which the load was applied (m2).
During the compressive strength test, care was taken to ensure that the lateritic bricks were properly positioned and aligned with the axis of the thrust of the compression machine to ensure uniform loading on the lateritic bricks (Neville, Citation2000). The results of the compressive strength tests were used to evaluate the effect of different levels of stabilizers (BA and PBA), curing age, water content, curing temperature, and size effects of the sample on the compressive strength of the lateritic bricks.
3. Results and discussion
3.1. XRD pattern for lateritic soil
The mineralogical analysis of lateritic soil sample is presented in . The XRD patterns indicated that quartz is the predominant mineral present in the lateritic soil sample. Quartz is silicon dioxide (SiO2) having a Moh’s hardness of 7. It is a durable material because it is highly resistant to both chemical and mechanical weathering. The presence of quartz in lateritic soil adds to its durability and will aid the formation of cementitious material (calcium silicate hydrate) when mixed with BA and PBA in the presence of water. Other minerals present in the lateritic soil include kaolinite{Al2Si2O5(OH)4}, moganite {SiO2}, gismondine {CaAl2Si2O8 · 4(H2O)}, sanidine {K(AlSi3O8)}, muscovite {(KF)₂(Al₂O₃)₃(SiO₂)₆} and dickite {Al2Si2O5(OH)4)}.
Figure 5. XRD pattern for lateritic soil (Obianyo, Onwualu et al., Citation2020)
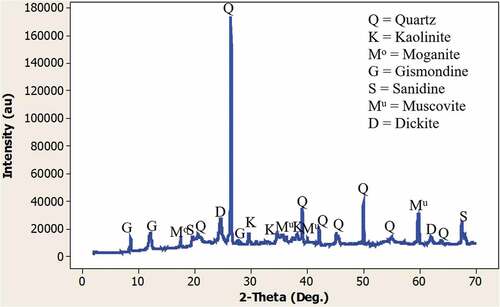
3.2. Infrared (IR) spectra of lateritic soil, BA and PBA
In order to properly understand the reactions and bonding types responsible for the strength improvement in the stabilized lateritic bricks, FTIR spectra of lateritic soil, BA and PBA were obtained as shown in respectively. The IR spectra of lateritic soil and PBA are dominated by Si-O-Al and Si-O-Si bands at 1030 cm−1 whereas the dominant band of BA had a strong peak with higher intensity at a higher wave number of 1050 cm−1. The bands at 440 cm−1, 470 cm−1, 540 cm−1, 580 cm−1 and 690 cm−1 are typical bands representing Si-O-Si possibly from quartz. The band with the wave number of 1050 cm−1 is O-C-O stretching bonds of the carbonate groups probably the calcium carbonate. These dominant bands of lateritic soil and PBA (Si-O-Al) imply that the combination of these materials with BA (containing CaO) will become cementitious when mixed with water as a result of the formation of calcium silicate hydrate (C-S-H) and calcium aluminate hydrate (C-A-H) (Moraes et al., Citation2019). The infrared spectra presented in indicated a broad absorbance at 2800–3850 cm−1 assigned to vibration of O-H due to the presence of chemically bound water which contributes to formation of cementitious materials between the soil particles and the stabilizers (Ojo et al., Citation2019). The chemically bound water provide sites for bonding of the soil matrix. indicates the typical bands of the hydroxyl stretch of kaolinite at 3620 cm−1 and 3690 cm−1 (Lemougna et al., Citation2014; Wi et al., Citation2018).
3.3. Microstructure of the materials and lateritic bricks
The polished products of BA, PBA, non-stabilized and stabilized lateritic bricks were analyzed by the Scanning Electron Microscopy (SEM) and the results are shown in . The morphology of BA in indicated the presence of spongy-like materials due to the presence of oxides of phosphorus in the BA (Ifka et al., Citation2012). The presence of flake-shaped particles was observed in the morphology of PBA as shown in indicating that palm bunch contains fibres and these could improve the tensile strength of the lateritic brick stabilized with PBA. The morphologies of both non-stabilized and stabilized lateritic bricks are shown in . These morphologies are similar at higher magnification, indicating the presence of quartz particles predominantly. However, the non-stabilized lateritic brick contains porous materials as shown in . Agglomerated morphology due to the accumulation of cementitious materials was observed for the stabilized lateritic soil as shown in . Quartz was found to be present in the morphology of the sample stabilized with 4% stabilizers (2% BA and 2% PBA) as represented in and this accounts for the higher compressive strength obtained for sample stabilized with 4% stabilizers.
Figure 7. Morphologies of (a) BA, (b) PBA, (c) non-stabilized lateritic brick, (d) 2% stabilized lateritic brick, (e) 4% stabilized lateritic brick, (f) 6% stabilized lateritic brick
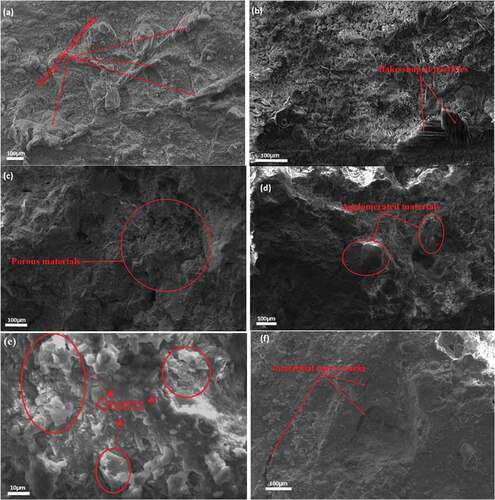
Presence of interstitial micro-cracks is observed in (which is for 6%) and this could be responsible for the lower compressive strength obtained for this sample compared to that obtained for samples stabilized with 2% and 4% proportions of stabilizers. The micro-cracks could be as a result of high silica content present in both the lateritic soil and PBA.
3.4. Influence of curing temperatures and water content on the compressive strength of lateritic bricks
These physical-chemical reactions can be enhanced by controlling the curing temperatures (Rahmouni et al., Citation2020). The results in indicated that the samples cured using the oven drying method (105°C) gave higher compressive strength compared to those cured at room temperature (23 ± 2°C) and sun drying temperature (37 ± 2°C). This implies that using oven drying temperature as low as 105°C provides for better development of the compressive strength unlike using oven drying temperature as high as 650°C (Obianyo, Onwualu et al., Citation2020). This trend of having lower strength development for samples cured at a higher temperature (650°C) could be as a result of the quick setting of the cementitious components of the stabilizers whereas the better development of the compressive strength for samples cured at lower oven drying temperature (105°C) could be attributed to the completion of the pozzolanic reactions between the soil particles and the soil stabilizers.
Figure 8. Influence of curing temperatures and water content on the compressive strength of lateritic bricks
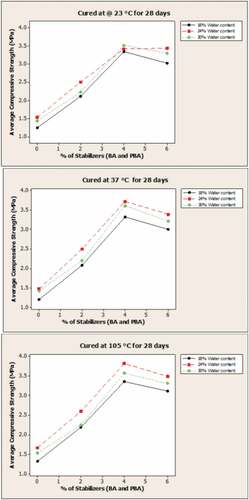
The pozzolanic reactions between the soil, soil stabilizers/additives, and water had been identified by previous research as the major contributor to the improvement of the compressive strength of the stabilized soil (Obianyo, Onwualu et al., Citation2020; Onyelowe, Citation2017b). Also, the behaviour of lateritic soil depends on the mineralogical constituents present in the soil as well as the amount of water in the soil (Joel & Edeh, Citation2013). The effects of water content on the compressive strength of lateritic brick samples are shown in . Among the different water contents (18%, 24%, and 30%) explored in this study, brick samples mixed with 24% water content gave higher compressive strength. The higher compressive strength obtained for 24% water content implies that the water content is adequate to ensure good workability of the composite (BA, PBA, and lateritic soil). Brick samples mixed with 18% water content gave lower compressive strength compared to that of 24% and this could be attributed to the inability for the mixture to mix thoroughly resulting in micro-cracks and debonding in the stabilized bricks. The compressive strength of the stabilized brick samples increased with an increase in the percentage of stabilizers up to 4% and then decreased with a further increase to 6% stabilizer as shown in . This implies that 4% stabilizers (2% BA and 2% PBA) by weight of the soil is the optimum quantity needed for the stabilization of the lateritic soil. Hence, the addition of excess stabilizers up to 6% will result in the formation of voids in the bricks leading to lower compressive strength.
3.5. Effect of weight percentage of the soil stabilizers and size effects of the specimens on the compressive strength of lateritic brick
The addition of the stabilizers (BA and PBA) influences the development of the compressive strength of the lateritic bricks as shown in . An increase in the compressive strength of the brick samples was observed up to 4% (2% BA and 2% PBA) after which it decreases. The increase in the compressive strength is due to the pozzolanic reactions between the lateritic soil and soil stabilizers resulting in the formation of cementitious materials (Calcium Silicate Hydrate), which accounts for the hardening and strengthening of the stabilized bricks (Obianyo, Anosike-francis et al., Citation2020; Obianyo, Onwualu et al., Citation2020; Onyelowe, Citation2017b). The decrease in the compressive strength observed after the addition of 6% stabilizers could be attributed to the excess of BA and PBA that do not take part in the pozzolanic reactions between the soil and the stabilizers. These unreacted stabilizers occupy spaces within the soil structure forming defects and weak bonds between the cementitious compounds and soil particles resulting in a decrease in the compressive strength of the stabilized bricks. Also, the addition of the soil stabilizers more than the optimum value produces voids within the bricks that impact negatively on the mechanical properties of the brick samples resulting in reduced compressive strength obtained for the samples stabilized with 6% stabilizers (3% BA and 3% PBA) as shown in . However, the compressive strength of all the stabilized samples was above 2.0 MPa and this conforms to the NBRRI compressive strength specifications of 1.65 MPa for laterite bricks (Aguwa, Citation2010). The higher compressive strength result obtained for the tested samples is 3.818 MPa and was obtained for the sample mixed with 24% water content and stabilized with 4% stabilizers, processed at 105°C for 24 hours and cured for 28 days.
Figure 9. Effects of weight percentage of the soil stabilizers and size effects of the specimens on the compressive strength of lateritic bricks
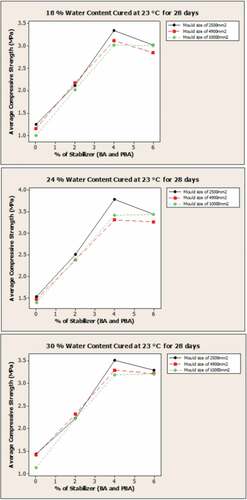
The effects of the specimen size on the compressive strength of lateritic bricks are shown in . For all the specimens with different water contents cured at different temperatures, the compressive strength increases as the size of the specimen gets smaller and vice versa. In other words, for bigger specimen sizes (10,000 mm2 and 4900 mm2), lower compressive strength was obtained whereas for smaller specimen size (2500 mm2), higher compressive strength was obtained as represented in .
This lower compressive strength obtained for the bigger specimen size could be attributed to the increase in the number of defects as the surface area of the specimen becomes larger. Hence, the smaller the size of the specimen, the lesser the defects present and the better the compressive strength. The increase in the compressive strength of the bricks as the specimen sizes reduce is consistent with the trends obtained in previous studies (Aubert et al., Citation2016; Knox et al., Citation2018; Mohammed et al., Citation2011; Segura et al., Citation2019).
3.6. Influence of curing age on the compressive strength of lateritic bricks
The effect of curing age on the compressive strength of earth-based materials has been studied (Chen et al., Citation2019; Johnson et al., Citation2014). The findings indicate that the compressive strength of earth-based materials increases as the curing age increases and vice versa. The influences of curing age on the compressive strength of the lateritic brick sample are shown in . It was observed that the compressive strength of the sample increases as the curing age increases and vice versa. Thus, brick samples cured for 28 days gave higher compressive strength compared to those cured for 7 and 14 days, respectively, as represented in .
This increase in the compressive strength with curing age is attributed to enough time provided for the complete pozzolanic reactions between the soil particles and the cementitious content of the soil stabilizers (BA and PBA) to take place.
4. Conclusions
The following conclusions were made from this research work.
Agro-waste ash stabilization of lateritic soil could be used to manufacture affordable and eco-friendly brick building materials for the fast growing population of Nigeria;
The higher compressive strength result obtained for the tested samples is 3.818 MPa and was obtained for the sample mixed with 24% water content and stabilized with 4 % stabilizers, processed at oven drying temperature (105°C) for 24 hours and cured for 28 days. Hence, the addition of a combination of 4% stabilizers (BA and PBA) improved the compressive strength of lateritic soil by 129%;
Brick samples produced with the smallest mould size (2500 mm2) and cured for 28 days gave higher compressive strength compared to that produced with larger mould size;
The use of PBA for a partial replacement for BA reduces the embodied energy consumed in the production of BA since the PBA takes lesser embodied energy to produce;
The FTIR result indicated that the IR spectra of lateritic soil and PBA were dominated by Si-O-Al band which accounted for the formation of cementitious materials (C-S-H and C-A-H) in the stabilized soil matrix;
The morphology of the tested stabilized lateritic soil indicated the presence of quartz and agglomerated materials due to the accumulation of cementitious materials and this accounted for the higher compressive strength obtained for sample stabilized with 4% stabilizers. Therefore, eco-friendly and sustainable brick building materials can be obtained by agro-waste ash (bone ash and palm bunch) stabilization of lateritic soil.
Acknowledgements
My immense gratitude goes to my family for all their contributions during the entire period of this research work. My acknowledgement is also due to Dr Emeso Ojo, Mr Njoku, Mr Sylvanus, and Mr Wisdom, who are members of staff at the Nigeria Building and Road Research Institute, Jabi, FCT, Abuja for all their support in my Thesis research. Laboratory assistance by Mr Tony and Mr Cyril of AfDB Laboratory, AUST is highly appreciated. Financial support of this work by the Pan African Materials Institute (PAMI) is tremendously appreciated.
Additional information
Funding
Notes on contributors

Ifeyinwa Ijeoma Obianyo
Ifeyinwa Ijeoma Obianyo is a recent PhD graduate of the Department of Materials Science and Engineering, African University of Science and Technology, Abuja, Nigeria. Her present research focus is on the development of sustainable building materials using agro-waste ash. The motive behind her research theme is providing affordable housing and other engineering infrastructure to the populace. This drive led to the research results presented in this paper.
References
- Aguwa, J. I. (2010). Performance of laterite-cement blocks as walling units in relation to sandcrete blocks. Leonardo Electronic Journal of Practices and Technologies, (16), 189–19.
- Ajayi, E. S. (2012). Effect of lime variation on the moisture content and dry density of lateritic soil in Ilorin, Nigeria. International Journal of Forest, Soil and Erosion, 2(November), 165–168.
- Akoto, B. K., & Singh, G. (1981). Some geotechnical properties of a lime-stabilized laterite containing a high proportion of aluminium oxide. Engineering Geology, 34(3), 185–199. https://doi.org/10.1016/0013-7952(81)90083-1
- Alam, I., Naseer, A., & Shah, A. A. (2015). Economical stabilization of clay for earth buildings construction in rainy and flood prone areas. Construction and Building Materials, 77, 154–159. https://doi.org/10.1016/j.conbuildmat.2014.12.046
- Alavéz-Ramírez, R., Montes-García, P., Martínez-Reyes, J., Altamirano-Juárez, D. C., & Gochi-Ponce, Y. (2012). The use of sugarcane bagasse ash and lime to improve the durability and mechanical properties of compacted soil blocks. Construction and Building Materials, 34, 296–305. https://doi.org/10.1016/j.conbuildmat.2012.02.072
- Amadi, A. A., & Okeiyi, A. (2017). Use of quick and hydrated lime in stabilization of lateritic soil: Comparative analysis of laboratory data. International Journal of Geo-Engineering, 8(1). https://doi.org/10.1186/s40703-017-0041-3
- Apampa, O. A. (2017). Environmental benefits of Corn Cob Ash in lateritic soil cement stabilization for road works. African Journal of Science, Technology, Innovation and Development, 1338, 1–5. https://doi.org/10.1080/20421338.2017.1399533
- Arancon, R. A. D., Lin, C. S. K., Chan, K. M., Kwan, T. H., & Luque, R. (2013). Advances on waste valorization: New horizons for a more sustainable society. Energy Science & Engineering, 1(2), 53–71. https://doi.org/10.1201/b19941
- Aubert, J. E., Maillard, P., J.c., M., & Rafii, M. A. (2016). Towards a simple compressive strength test for earth bricks? Materials and Structures, 49(5), 1641–1654. https://doi.org/10.1617/s11527-015-0601-y
- Chen, F., Wu, K., Ren, L., Xu, J., & Zheng, H. (2019). Internal curing effect and compressive strength calculation of recycled clay brick aggregate concrete. Materials (Basel), 12(1815), 1–14.
- Dang, L. C., Fatahi, B., & Khabbaz, H. (2016). Behaviour of expansive soils stabilized with hydrated lime and Bagasse fibres. Procedia Engineering, 143(Ictg), 658–665. https://doi.org/10.1016/j.proeng.2016.06.093
- Egenti, C., & Khatib, J. M. (2016). Sustainability of compressed earth as a construction material. Sustainable Construction Materials, 309–341. https://doi.org/10.1016/b978-0-08-100370-1.00013-5
- EN 206: 2013. (2013). Concrete – Specification, Performance, Production and Conformity. British Standards Institution.
- Fadele, O. A., & Ata, O. (2018). Water absorption properties of Sawdust Lignin stabilised compressed laterite bricks. Case Studies in Construction Materials, 9, e00187. https://doi.org/10.1016/j.cscm.2018.e00187
- Figueiredo, M., Fernando, A., Martins, G., Freitas, J., Judas, F., & Figueiredo, H. (2010). Effect of the calcination temperature on the composition and microstructure of hydroxyapatite derived from human and animal bone. Ceramics International, 36(8), 2383–2393. https://doi.org/10.1016/j.ceramint.2010.07.016
- García-Díaz, I., Gázquez, M. J., Bolivar, J. P., & López, F. A. (2016). Characterization and valorization of norm wastes for construction materials. Management Hazardous Waste Environment, 13–36. https://doi.org/10.5772/57353
- Gouvêa, D., Tisse, T., Kahn, H., De Souza, E., & Antoniassi, J. L. (2015). Using Bone Ash as an Additive in Porcelain Sintering. Ceramics International, 41(1), 487–496. https://doi.org/10.1016/j.ceramint.2014.08.096
- Hamada, H. M., Al-attar, A. A., Yahaya, F. M., Muthusamy, K., Tayeh, B. A., & Humada, A. M. (2020). Effect of high-volume ultrafine palm oil fuel ash on the engineering and transport properties of concrete. Case Studies in Construction Materials, 12. https://doi.org/10.1016/j.cscm.2019.e00318
- Ifka, T., Palou, M. T., & Bazelová, Z. (2012). The influence of CaO and P2 O5 of Bone Ash upon the reactivity and the burnability of cement raw mixtures. Ceramics - Silikaty, 56(1), 76–84.
- Jamil, M., Kaish, A. B. M. A., Raman, S. N., & Zain, M. F. M. (2013). Pozzolanic contribution of rice husk ash in cementitious system. Construction and Building Materials, 47, 588–593. https://doi.org/10.1016/j.conbuildmat.2013.05.088
- Jayasinghe, C., & Kamaladasa, N. (2006). Compressive strength characteristics of cement stabilized rammed earth walls. Construction and Building Materials, 21(11), 1971–1976. https://doi.org/10.1016/j.conbuildmat.2006.05.049
- Joel, M., & Edeh, J. E. (2013). Soil modification and stabilization potential of calcium carbide waste. Advanced Materials Research, 824, 29–36. https://doi.org/10.4028/www.scientific.net/AMR.824.29
- Johnson, O. A., Madzlan, N., & Kamaruddin, I. (2014). Effect of curing age on the compressive strength of petrovege blocks. Advanced Materials Research, 980, 91–96. https://doi.org/10.4028/www.scientific.net/AMR.980.91
- Knox, C. L., Dizhur, D., & Ingham, J. M. (2018). Experimental study on scale effects in clay brick masonry prisms and wall panels investigating compression and shear related properties. Construction and Building Materials, 163, 706–713. https://doi.org/10.1016/j.conbuildmat.2017.12.149
- Lemougna, P. N., Madi, A. B., Kamseu, E., Melo, U. C., Delplancke, M. P., & Rahier, H. (2014). Influence of the processing temperature on the compressive strength of Na activated lateritic soil for building applications. Construction and Building Materials, 65, 60–66. https://doi.org/10.1016/j.conbuildmat.2014.04.100
- Madurwar, M. V., Ralegaonkar, R. V., & Mandavgane, S. A. (2013). Application of Agro-Waste for sustainable construction materials: A review. Construction and Building Materials, 38, 872–878. https://doi.org/10.1016/j.conbuildmat.2012.09.011
- Malkanthi, S. N., Balthazaar, N., & Perera, A. A. D. A. J. (2020). Lime stabilization for compressed stabilized earth blocks with reduced clay and silt. Case Studies in Construction Materials, 12, e00326. https://doi.org/10.1016/j.cscm.2019.e00326
- Mohammed, A., Hughes, T. G., & Mustapha, A. (2011). The effect of scale on the structural behaviour of masonry under compression. Construction and Building Materials, 25(1), 303–307. https://doi.org/10.1016/j.conbuildmat.2010.06.025
- Moraes, M. J. B., Moraes, J. C. B., Tashima, M. M., Akasaki, J. L., Soriano, L., Borrachero, M. V., & Payá, J. (2019). Production of bamboo leaf ash by auto-combustion for pozzolanic and sustainable use in cementitious matrices. Construction and Building Materials, 208, 369–380. https://doi.org/10.1016/j.conbuildmat.2019.03.007
- Muntohar, A. S. (2011). Engineering characteristics of the compressed-stabilized earth brick. Construction and Building Materials, 25(11), 4215–4220. https://doi.org/10.1016/j.conbuildmat.2011.04.061
- Neville, A. M. (2000). Properties of Concrete (4th ed.) (low-price ed.). Pearson Education Asia Publ. produced by Longman Malaysia.
- Nnochiri, E. S., & Adetayo, O. A. (2019). Geotechnical properties of lateritic soil stabilized with Corn Cob Ash. ACTA TECHNICA CORVINIENSIS – Bulletin of Engineering, XII(1), 73–76. https://doi.org/10.20944/preprints201811.0100.v1
- Obianyo, I. I., Anosike-francis, E. N., Odochi, G., Geng, Y., Jin, R., Peter, A., & Soboyejo, A. B. O. (2020). Multivariate regression models for predicting the compressive strength of bone ash stabilized lateritic soil for sustainable building. Construction and Building Materials, 263, 120677. https://doi.org/10.1016/j.conbuildmat.2020.120677
- Obianyo, I. I., Onwualu, A. P., & Soboyejo, A. B. O. (2020). Mechanical behaviour of lateritic soil stabilized with bone ash and hydrated lime for sustainable building applications. Case Studies in Construction Materials, 12, e00331. https://doi.org/10.1016/j.cscm.2020.e00331
- Ojo, E. B., Mustapha, K., Teixeira, R. S., & Savastano, H. (2019). Development of unfired earthen building materials using muscovite rich soils and Alkali activators. Case Studies in Construction Materials, 11, e00262. https://doi.org/10.1016/j.cscm.2019.e00262
- Okeyinka, O. M., Olutoge, F. A., & Okunlola, L. O. (2018). Durability Performance of Cow-Bone Ash (CBA) Blended Cement Concrete in Aggressive Environment. International Journal of Scientific and Research Publication, 8(12), 10–13. https://doi.org/10.29322/IJSRP.8.12.2018.p8408
- Ola, S. A. (2013). Geotechnical properties and behaviour of some stabilized Nigerian lateritic soils. Quarterly Journal of Engineering Geology and Hydrogeology. https://doi.org/10.1144/GSL.QJEG.1978.011.02.04
- Oluremi, J. R., Adedokun, S. I., & Osuolale, O. M. (2012). Stabilization of poor lateritic soils with coconut Husk Ash. International Journal of Engineering Research & Technology, 1(8), 1–9.
- Oluwatuyi, O. E., Adeola, B. O., Alhassan, E. A., Nnochiri, E. S., Modupe, A. E., Elemile, O. O., Obayanju, T., & Akerele, G. (2018). Ameliorating effect of milled eggshell on cement stabilized lateritic soil for highway construction. Case Studies in Construction Materials, 9, e00191. https://doi.org/10.1016/j.cscm.2018.e00191
- Omoniyi, T. E. (2019). Potential of oil palm (Elaeisguineensis) empty fruit bunch fibres cement composites for building applications. Agricultural Engineering, 153–163.
- Onakunle, O., Omole, D. O., & Ogbiye, A. S. (2020). Stabilization of lateritic soil from Agbara Nigeria with ceramic waste dust stabilization of lateritic soil from Agbara Nigeria with ceramic waste dust. Cogent Engineering, 6(1). https://doi.org/10.1080/23311916.2019.1710087
- Onyelowe, K. C. (2017a). Local nanostructured ashes synthesized by incineration, pulverization and spectrophotometric characterization of solid wastes ashes for use as admixtures in soil stabilization. The International Journal of Sustainable Construction Engineering Technology, 7(2), 50–64.
- Onyelowe, K. C. (2017b). Nanosized Palm Bunch Ash (NPBA) stabilisation of lateritic soil for construction purposes. International Journal of Geotechnical Engineering, (April), 1–9. https://doi.org/10.1080/19386362.2017.1322797
- Otunyo, A. W., & Chukuigwe, C. C. (2018). Investigation of the impact of palm bunch ash on the stabilization of poor lateritic soil. Nigerian Journal of Technology, 37(3), 600–604. https://doi.org/10.4314/njt.v37i3.6
- Pacheco-Torgal, F., & Jalali, S. (2011). Eco-Efficient construction and building materials. Construction Management and Economics, 31(11), 1164–1165. https://doi.org/10.1080/01446193.2013.833665
- Pourkhorshidi, A. R., Najimi, M., Parhizkar, T., Jafarpour, F., & Hillemeier, B. (2010). Cement & concrete composites applicability of the standard specifications of ASTM C618 for evaluation of natural Pozzolans. Cement and Concrete Composites, 32(10), 794–800. https://doi.org/10.1016/j.cemconcomp.2010.08.007
- (Rahmouni, E. Z. A., Tebbal, N., & Omri, I. Y. (2020). Effect of curing temperature in the alkali-activated brick waste and glass powder mortar and their influence of mechanical resistances RICON19 - REMINE International Conference on Valorization of mining and industrial wastes into construction materials by alkali-activation, KnE Eng, 49–61. https://doi.org/10.18502/keg.v5i4.6794.
- Rao, S. M., & Shivananda, P. (2005). Role of curing temperature in progress of lime-soil reactions. Geotechnical and Geological Engineering, 23, 79–85. https://doi.org/10.1007/s10706-003-3157-5
- Rimal, S., Poudel, R. K., & Gautam, D. (2019). Experimental study on properties of natural soils treated with cement Kiln Dust. Case Studies in Construction Materials, 10, e00223. https://doi.org/10.1016/j.cscm.2019.e00223
- Sadh, P. K., Duhan, S., & Duhan, J. S. (2018). Agro-industrial wastes and their utilization using solid state fermentation: A review. Bioresources and Bioprocessing, 5(1), 1–15. https://doi.org/10.1186/s40643-017-0187-z
- Segura, J., Pelà, L., Roca, P., & Cabané, A. (2019). Experimental analysis of the size effect on the compressive behaviour of cylindrical samples core-drilled from existing brick masonry. Construction and Building Materials, 228, 116759. https://doi.org/10.1016/j.conbuildmat.2019.116759
- Series, I. O. P. C., & Science, M. (2019). Stabilization of lateritic soil with cement – Oil palm empty fruit bunch ash blend for California bearing ratio base course requirement. IOP Conference Series: Materials Science and Engineering Papers, 640, 1–11. https://doi.org/10.1088/1757-899X/640/1/012085
- Udoetok, I. (2012). Characterization of Ash Made from Oil Palm Empty Fruit Bunches (OEFB) characterization of ash made from Oil Palm Empty Fruit Bunches (Oefb). International Journal of Environmental Science, 3(1), 518–524. https://doi.org/10.6088/ijes.2012030131033
- Wi, K., Lee, H. S., Lim, S., Song, H., Hussin, M. W., & Ismail, M. A. (2018). Use of an agricultural by-product, nano sized palm oil fuel ash as a supplementary cementitious material. Construction and Building Materials, 183, 139–149. https://doi.org/10.1016/j.conbuildmat.2018.06.156