Abstract
Environmental distribution of cadmium (Cd) and lead (Pb) were studied in two wetlands (alluvial plain and inland depression) in Ibadan, Nigeria. Mean and median values of variables measured across the wetlands showed that soil properties varied considerably. Hydraulic conditions, soil organic matter (SOM), and particle size distribution contributed to the distribution of heavy metals recorded. Profile distribution of Cd and Pb maintained two patterns: (1) linear distribution pattern, where concentrations of Cd and Pb increase and decrease respectively, with increase in soil depth and (2) middle depth enrichment. Active carbon (AC), SOM, and clay fraction affected the surface and profile distribution of Cd and Pb. Cadmium showed significant correlation (R2 = −0.61* and 0.64*) with AC and SOM, respectively, while Pb had R2 values of 0.77* and 0.57 with SOM and clay content, respectively. The non-residual fractions of the heavy metals increased with increasing metal loading and SOM. This study is useful in assessing chemical changes for Cd and Pb in different wetlands, and their potential release to the environment.
Public Interest Statement
Study on distribution and forms of heavy metals in urban wetlands is important in environmental health assessment. In sub-Sahara Africa, wetlands are important because of their role in ensuring food security in the sub region. These wetlands are used for dry season farming especially vegetable cultivation. Despite the delicate balance that occurs in these lowlands, their utilization in agricultural production is on the increase. In this study, we determined the surface and profile distribution of lead and cadmium and also the forms of these metals in order to determine their potential for plant uptake. It was observed that distribution and availability of these metals were influenced by human activities, moisture conditions, organic matter, and particle size. These wetlands can be a source of heavy metal accumulation in human beings when vegetable cultivated in them are consumed.
Competing Interests
The authors declare no competing interest.
1. Introduction
Within an agro-ecosystem, soil plays a major role in food production, and it stands as the interface between human activities and other parts of the environment. Soil, not only serves as source of essential nutrients for crops but also functions as sink for heavy metal pollutants. This ecological function of soil is most pronounced in wetlands. Wetlands are usually low areas where heavy metals are more easily accumulated due to changes in natural environment and influences of human activities (May & Edwards, Citation2001), and this may also be due to a variety of physicochemical processes such as adsorption, ligand exchange, and sedimentation. In wetlands, heavy metals could exist mainly in water, mud at the bottom, and in plants or other organisms. The occurrence of wetland in Nigeria according to Okusami and Rust (Citation1992) has been associated with three landform types, which are inland depression, alluvial plains, and coastal plains. They are used by peasant farmers in sub-Sahara Africa (SSA) for agricultural production and water sources for domestic consumption as well as dump sites for urban domestic wastes (Binns, Maconachie, & Tanko, Citation2003; Mbabazi et al., Citation2010). Because wetlands are important sources of food and water for human beings in SSA, they also constitute health hazard due to heavy metal deposits. It is therefore pertinent to protect soil resources while detail research studies on the distribution, transfer, and forms of heavy metals in wetlands are of great importance.
It has been documented that the total heavy metal contents in soils cannot determine their availability and toxicity (Clozel, Ruban, Durand, & Conil, Citation2006; Pagnanelli, Moscardini, Giuliano, & Toro, Citation2004). It is the physicochemical forms of any metal that determine its potential availability and toxicity. Thus, the speciation and distribution study of heavy metals in soils has become one of the most important areas in environmental research. The chemical speciation of metal ions in soil involves the fractionation of its total content into exchangeable, acid-extractable, reducible, oxidizable, and residual forms (Christine, Sylvaine, & Michel, Citation2002). The exchangeable and acid-extractable fractions are mobile fractions that are considered to be easily available. The oxidizable and reducible forms will be leached out only under extreme conditions, while the residual fraction is almost inert. The sequential extraction method proposed by Krishnamurti and Naidu (Citation2000) provides information about the distribution of heavy metal associated with different specific solid phases in soils. This contributes to the understanding of the distribution and availability of Cd and Pb in some selected wetlands. Research has shown that the stabilization of heavy metals is being controlled by different physicochemical properties (Cui, Yang, Yang, & Zhang, Citation2009; Ip, Li, Zhang, Wai, & Li, Citation2007). Research by Ip et al. (Citation2007) reported that the presence of organic matter, divalent iron, and clay enhances the presence of heavy metals in soils; while Cui et al. Citation(2009) identified carbonate, phosphate, and Fe/Mn oxides as factors aiding their immobilization and deposition. The processes of decalcification, desalinization, and oxidation of reduced Fe, Mn, and sulfur compounds can induce changes in redox conditions, pH value, mobility of phosphorus, and behavior of Fe and other metals in the soil (Giani, Ahrens, Duntze, & Irmer, Citation2003). Within a given wetland type, these physicochemical properties vary and therefore aid the release and occurrence of heavy metal forms in varying proportion. Previous studies (Ngole, Citation2011; Oluwatosin et al., Citation2010) presented that the accumulation of heavy metals, such as Cd and Pb, is significantly affected by anthropogenic activities. However, the distribution and forms of Cd and Pb in different soil depths in the arrays of wetlands in southwest Nigeria have not been investigated. Understanding Cd and Pb distribution, forms, and accumulation in inland depression and alluvial plain wetlands plays a crucial role in ecological risk assessment. Thus, this study was set out to investigate Cd and Pb distribution in different types of wetlands, their variation with depth, and characterize the geochemical fractions of Cd and Pb in these wetlands.
2. Materials and methods
2.1. Site description
Different types of wetlands, specifically, inland depression and alluvial plain wetlands, were identified within Ibadan metropolis, southwest Nigeria. Two alluvial plain wetlands and one inland depression wetland were selected for this study (Figure ). The alluvial plain wetlands are located at Omi Adio (AP1) (7° 23.8′N and 3° 45.2′E) covering an area of 5.1 ha and Ojoo Barrack (AP2) (7° 26.8′N and 3° 56.6′E) with an area of 3.2 ha, which are drained by Omi and Ajibode rivers, respectively. The inland depression wetland is located within the University of Ibadan (ID) (7° 26.9′N and 3° 53.8′E) with an area of 2.2 ha. The three sites are peri-urban wetlands with a tropical humid climate characterized by high humidity, temperature, and precipitation. The annual precipitation in the area is 1,300 mm, with humidity ranging between 60 and 90%, and minimum and maximum temperatures of 17–21°C and 29–36°C, respectively.
The study sites are characterized by overlaying Precambrian Basement complex rocks consisting of granites, gneisses, and quartzite. The soils of Omi Adio and Ojoo Barrack are classified as Eutric Fluvisol (FAO/UNESCO) and Aeric Fluvaquent (USDA), while the wetland soils of University of Ibadan are classified as Eutric Gleysol (FAO/UNESCO) and Typic Tropaquent (USDA). All the wetland sites receive runoff from adjacent build-up areas where household waste are generated in varying degrees. The wetlands are usually cultivated with vegetable and maize during the dry season (November–April). During this period, the water table might have been lowered, while little or no farming activity takes place during the raining season.
2.2. Soil sample collection and analyses
Soil samples were collected during the dry season in December 2013. Each of the wetland sites was divided into four quadrants representing four sampling plots. From each sampling plot, five random points were selected for soil sampling. Two techniques were used to obtain samples from the plots: (1) a 5 cm by 5 cm core sampler was used to collect undisturbed core samples for bulk density and soil moisture determination, and (2) composite samples were collected from 0–20, 20–40, 40–60, 60–80, and 80–100 cm depths, using a stainless steel soil auger. The composite soil samples were air dried at room temperature and allowed to pass through a 2-mm nylon sieve to remove gravels. The samples were stored in polythene bags prior to soil analysis.
Particle size distribution was determined using a modified Boyoucos hydrometer method as described by (Gee & Or, Citation2002). The undisturbed cores were used to determine soil bulk density (BD) (Grossman & Reinsch, Citation2002). Moisture content was determined using the procedure described by Lowery, Arshad, Lal, and Hickey (Citation1996). Soil pH was determined in distilled water with a pH meter in a 1:1 soil to water suspension ratio after 1 h of equilibration. Exchangeable cations (calcium-Ca, magnesium-Mg, potassium-K, and sodium-Na) were determined by extracting with 1 M NH4OAc (ammonium acetate) solution as described by Okalebo, Gathua, and Woomer (Citation1993). The amounts of exchangeable Na and K in the extract were determined by flame photometry (Jenway PFP 7), while Ca and Mg were measured by atomic absorption spectrophotometry (AAS) (BUCK 211). The effective cation exchange capacity (ECEC) of the soil was determined by addition of the values of exchangeable cations and hydrogen (H+) (Okalebo et al., Citation1993). Soil organic carbon (SOC) was determined by loss on ignition and the SOC content was converted to soil organic matter content (SOM) by multiplying SOC by a Van Bemmelen factor of 1.724. Active carbon (AC) was determined using permanganate oxidation following the procedure of Weil, Islam, Stine, Gruver, and Samson-Liebig (Citation2003). Crystalline iron (Fed) and aluminum (Ald) were determined by dithionite–citrate–bicarbonate extraction (Coffin, Citation1963) and amorphous iron (Feo) and aluminum (Alo) by ammonium oxalate extraction (Schwertmann, Citation1964). The total concentrations of Cd and Pb in the soils were determined by AAS after being digested with aqua regia and HClO4.
2.3. Sequential extraction of soil Cd and Pb
The chemical fractionation technique of Krishnamurti and Naidu (Citation2000) was used in the sequential extraction. The procedure separates heavy metals into seven different fractions as: exchangeable (F1), carbonate bound (F2), metal–organic complex or easily reducible metal bound (F3), organic bound (F4), amorphous Fe–Mn oxide bound (F5), crystalline Fe–Mn oxide bound (F6), and detrital (residual) (F7). The stepwise extraction is presented in Table . Following each successive extraction, the mixture was centrifuged at 14,000 rpm for 30 min. The supernatants were removed with a pipette, filtered with 0.2 μ nucleopore polycarbonate membrane filters, and analyzed for Cd and Pb concentration using AAS. The extraction was done in triplicates and two independent technologists performed the entire process of digestion and analysis while a high level of reproducibility was attained with R2 of 0.95 for Cd and 0.98 for Pb between the two analyses.
Table 1. Procedure for sequential extraction of Cd and Pb in the soils
2.4. Statistical analyses
The data obtained were analyzed using the analysis of variance (ANOVA) with statistical application software (SAS Institute, Citation2002). Significant means were separated using least significance difference (LSD) at 5% significant level of probability. The relationships among soil parameters were evaluated using Pearson correlation analysis to determine whether there are significant correlations among the pairs.
3. Results and discussion
3.1. General properties of soils
Mean and median values of variables measured across the plots revealed that soil properties in the wetlands varied widely. The chemical properties showed more variability when compared with the physical properties except for soil pH (Table ). For example, the coefficient of variation (CV) obtained from exchangeable Ca, Mg, and ECEC exceeded 30%, compared with 10.9, 13.0, and 15.3% for BD, clay, and sand content, respectively (Table ). Also, concentrations of Pb and Cd were highly variable with CVs of 55.6 and 94.5%, respectively. When compared with the study of Aiyelari and Oshunsanya (Citation2008) on wetland soils in Ibadan metropolis, BD measurements and soil texture values measured generally fell within the ranges reported in these studies. In contrast, Ogban and Babalola (Citation2009) reported lower BD values ranging from 0.78 to 1.36 Mg m−3 in some valley bottom soils of southwestern Nigeria. Low BD reported in this study may be a consequent of organic matter content of the soils. Moreover, frequent cultivation of the land made the soil loose and ultimately contributed to the low BD.
Table 2. Descriptive statistics of soil properties sampled to a depth of 20 cm (n = 60)
Although, soil chemical properties in the wetlands generally had high CVs, soil pH measured had a relatively low CV of 5.3% with a range of 5.3–6.6 (Table ). It is evident that the concentration of Pb (ranging from 112.0 to 490.7 mg kg−1) was high in the wetlands, followed by Cd (0.11–4.90 mg kg−1) with a median value of 1.06 mg kg−1. The CV obtained for both Pb and Cd were higher than 50% indicating high variability amongst the wetlands. Oluwatosin, Adeyolanu, Dauda, and Akinbola (Citation2008) reported similar high variability in heavy metal concentration of some valley bottom soils of urban cities in south west Nigeria. These variations could be attributed to the amount of organic matter and clay particles in the wetlands. Also, hydraulic conditions could have played a part in the distribution of Pb and Cd. However, the total extractable heavy metal content in the wetlands cannot predict the availability of the metals for plant uptake. Thus, further investigation was carried out on the samples to understand the chemical speciation of Pb and Cd in the wetlands.
3.2. Surface properties of soils between wetlands
The mean values of some physical and chemical properties of the wetland soils are presented in Table . All wetland soils were dominated by moderate sand content of 570 and 700 g kg−1 for ID and AP2, respectively. The clay and silt contents in the different wetlands varied from 159 to 289 g kg−1 for clay and from 141 to 144 g kg−1 for silt. The soils were slightly acidic with pH values varying from 5.8 to 6.1, with the highest soil pH observed under ID wetland (Table ).
Table 3. Mean surface soil properties (0 – 20 cm) for different wetlands
Figure shows moisture content of the wetlands at various depths. There were significant differences (p ≤ 0.05) in moisture content amongst the wetlands at 0–20 and 20–40 cm depths. The observed moisture content is a reflection of the drainage conditions in the wetlands. The well-drained AP wetlands had lesser moisture content than ID wetland. The improved drainage observed under alluvial plain (AP) wetland soils was enhanced by the higher sand fraction obtained from these soils.
Figure 2. Variation in moisture content with soil depth. Depths with the same letter amongst the wetlands are not significantly different at p ≤ 0.05, ns: not significantly different. AP: alluvial plain wetland; ID: inland depression wetland.
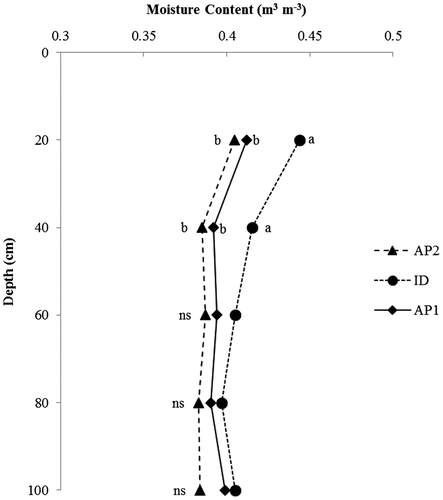
The AC, a measure of the fraction of SOM that is readily available as carbon and energy source for the soil microbial community, was significantly higher (p ≤ 0.01) under the alluvial plain wetlands (AP1 and AP2) than ID, and it was in the order of AP2 > AP1 > ID. This perhaps increased the soil aeration under AP1 and AP2, and thus increased microbial activities, when compared with ID with poorer drainage. Wan, Song, Guo, Wang, and Huang (Citation2008) reported that wetlands with water stagnation or continuous flooding did not favor the growth and multiplication of microbes leading to reduction in enzyme activities. Although, ECEC of the soils were generally low, they were significantly different (p ≤ 0.05) from each other (Table ). The significantly lower values of ECEC under AP1 and AP2 can be attributed to the leaching of exchangeable cations that occurred under the better drained alluvial plain wetland soils.
3.3. Surface distribution of metals in wetlands
The surface distribution of crystalline- Fe and Al (Fed and Ald) and amorphous- Fe and Al (Feo and Alo) in the wetlands is summarized in Table . Generally, Fed and Ald were higher than their Feo and Alo. The higher amount of Fed and Ald in these soils suggested that more amorphous Fe and Al had been transformed to crystalline form. The high tropical soil temperature, prolonged dry season coupled with high precipitation and age of Fe and Al released during weathering may have resulted in more Fed and Ald in the wetland soils (Olaleye, Ogunkunle, & Sahrawat, Citation2000). High temperature causes Al and Fe oxides to dehydrate and subsequently shift from an form to a more crystalline state. In comparison, there were no significant differences in Fe and Al forms among the wetlands, except Fed (Table ). Although, a low value of AC was obtained from ID, the significantly lower values of Fed can be attributed to poor drainage which led to accumulation of organic material (Table ). It has been reported that organic matter hinders the rate of crystallization of iron oxides (Schwertmann, Citation1964). Furthermore, the higher amount of crystalline-Fe (Fed) in the alluvial plain wetlands could also have contributed to the significantly lower values of ECEC in these soils. Hendershot and Lavkulich (Citation1983) reported that iron oxides coatings on clay minerals may decrease the cation exchange capacity of soils.
The mean concentration of Pb and Cd in 0–20 cm soil depth was used to compare the variation in heavy metal distribution in the wetlands. Within the wetlands, there were significant differences (p ≤ 0.05) in the surface distribution of Pb and Cd (Table ) with Pb having the higher concentration in each of the soils. The statistically higher values of Pb and Cd reported under ID could be as a result of the accumulation of organic material in the wetland and higher input of heavy metals resulting from human activities. Retained organic materials in the soil surface enhanced the concentrations of heavy metals by adsorption, complexation, and chelation. A high positive correlation between SOM and concentrations of Pb and Cd (p ≤ 0.05) (Table ) partly explained the metal concentration distribution order of ID > AP2 > AP1 with ascending SOM content of AP1 < AP2 < ID. Du Laing et al. Citation(2007) also established that increasing organic matter enhances metal concentration of floodplain soils. Meanwhile, the relatively higher amount of clay fraction also accounted for the metal distribution in the inland depression wetlands.
Table 4. Pearson correlation matrix among Pb and Cd concentrations and soil properties in wetlandsTable Footnotea
3.4. Profile distribution of metals in wetlands
The linear relationships between metal concentrations and soil depth are identified by the high values of the correlation coefficient (R2 ranges from 0.69 to 0.91, Figures and ). Results showed that the distribution of Pb and Cd varied significantly with sampling depth. Similar reports by Du Laing, Meers, et al. (Citation2009) indicated that the concentrations of some metals (Cd, Ni, and Pb) are significantly affected by depth. Generally, Pb concentrations in the top layers (0–20 cm) of the wetlands were much higher than those in the deeper layers except for ID, where Pb concentration (383.2 mg kg−1) in the 0–20 cm depth was lower than the 20–40 cm (391.1 mg kg−1) and 40–60 cm (384.7 mg kg−1) depths (Figure ). This distribution pattern suggests a middle depth enrichment of Pb under ID resulting probably from metal enrichment during deposition. The reduction in Pb concentration with depth can be ascribed to the decrease in SOM with depth in the wetlands (Ferraz & Lourençlo, Citation2000). Although, Pb showed a positive correlation with clay content in the 0–20 cm depth (Table ), there was a reduction in Pb concentration with depth despite the slight increase in clay content with depth. This indicates that the mobility of Pb in these wetlands was strongly controlled by the presence of organic complexes which was lower at deeper depths. In contrast to the reduction of Pb down the profile under alluvial plain wetlands (AP1 and AP2), the distribution of Cd increased with depth under AP1 and AP2 but showed a similar trend with Pb under ID (Figure ). The mixed distribution pattern of Cd amongst the wetlands may be attributed to redox-induced changes, resulting from the improved drainage in the alluvial plain wetlands. In these wetlands, processes such as metal binding capacity of humic materials, insoluble metal sulfide formation, and changes in Fe/Mn-oxyhydroxides are inhibited (Du Laing, Rinklebe, et al., Citation2009) thereby enhancing the mobility of Cd in the alluvial plain wetlands. This view is corroborated by Vandecasteele, Du Laing, and Tack (Citation2007), who reported that Cd is more mobile and readily available for plant uptake in oxidized soils with good drainage system when compared to reduced conditions.
3.5. Chemical fractions of Pb and Cd in the wetlands
Geochemical fractions of Pb and Cd in the wetlands from the sequential extraction are shown in Figures and . The concentration of Pb associated with different fractions in the alluvial plain wetlands was in the order of organic > metal–organic complex > carbonate > crystalline Fe–Mn oxide > amorphous Fe–Mn oxide > exchangeable > detrital. In contrast, the order under inland depression wetland was organic > carbonate > metal–organic complex > amorphous Fe–Mn oxide > crystalline Fe–Mn oxide > exchangeable > detrital. In all the wetlands, Pb was mostly concentrated in the organic bound fraction (Figure ). The concentration of organic bound Pb (Figure ) accounted for over 50% of the total Pb content in all the wetland soils. The affinity of Pb to organic material explains the high values of organic bound Pb observed in the wetlands (Du Laing et al., Citation2007). Lead tends to undergo complexation reaction with strong binding sites which are probably offered in organic matter. Apart from the organic bound Pb, lead had specific binding sites in the different solid phases within the soils of the wetlands. The Pb loading might have contributed to the exchangeable fraction (2.2 to 3.3% of the total Pb) observed in all the wetlands since Pb occupied the weaker binding sites with its increasing concentration in the soils (Figure ). The least concentration of Pb form in all the wetlands was observed under detrital (residual) fractions (Figure ). The observed concentration of the inert (residual) fraction of Pb (detrital, amorphous Fe–Mn and crystalline Fe–Mn oxide fractions) in all the wetlands is close to the concentrations of Pb in the continental crust (12.5 mg kg−1) and worldwide shale (20.0 mg kg−1). Therefore, it is possible to suggest that the high concentration of Pb in the non-residual (readily available) fractions in the studied wetlands were probably as a result of anthropogenic activities.
Figure 5. Lead concentration in each geochemical fraction of the wetland soils (0–20 cm). AP: alluvial plain wetland; ID: inland depression wetland; Concentrations with the same letter within the same geochemical fraction are not significantly different at p ≤ 0.05.
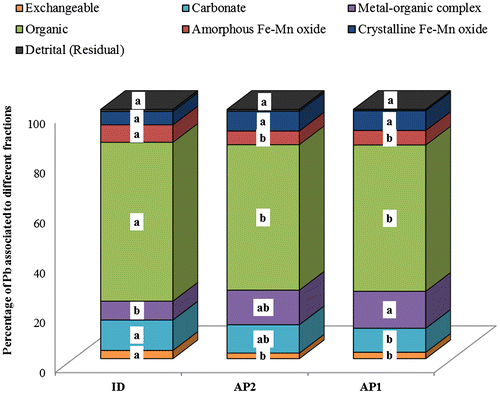
Figure 6. Cadmium concentration in each geochemical fraction of the wetland soils (0–20 cm). AP: alluvial plain wetland; ID: inland depression wetland; Concentrations with the same letter within the same geochemical fraction are not significantly different at p ≤ 0.05.
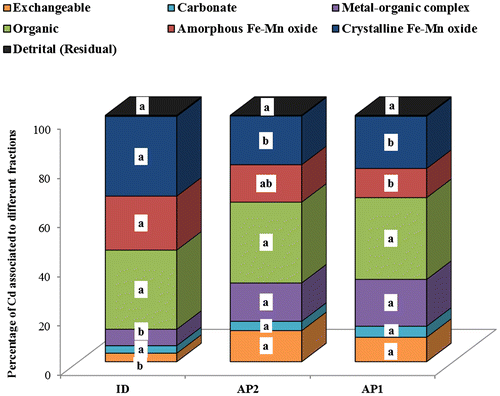
Figure shows the geochemical fractions of Cd in the different wetlands. The concentration of water-soluble exchangeable fraction differ significantly (p ≤ 0.05) between the wetlands, and it varied from 0.11 mg kg−1 (ID) to 0.42 mg kg−1 (AP2). Approximately 38–52% of the total Cd was associated with organic matter. The values of Cd obtained from sequential extraction suggest that it prefers to form complexes with organic matter in the wetland soils. The apparent stability and lability of Cd complexes in the soils is found to be dependent on Cd loading. It was observed that Cd prefers to form thermodynamically strong complexes (especially with organic matter) at low Cd loading. However, the concentration of thermodynamically weak complexes (exchangeable and carbonate bound) gradually increases with the increasing concentrations of Cd in the soils (Ferraz & Lourençlo, Citation2000). The detrital fraction of Cd in the studied wetlands was less than 1% of the total metal contents. The high concentration of Cd in the readily available fraction may lead to huge potential risk for environmental sustainability and human health. With the amount of Cd in these fractions, there is enough Cd for accumulation from the soil matrix into plant tissues.
4. Conclusions
Surface and profile distributions of Cd and Pb in the three wetlands showed that there were accumulation and mobility of these metals, which vary with soil depths. The surface distribution was influenced by anthropogenic activities, hydraulic conditions, AC, SOM, and particle size distribution. The observed profile distribution patterns in the wetlands were linear distribution and middle enrichment. The linear distribution showed an increase in Cd and decrease in Pb concentration under AP wetland with increasing depth, while the middle enrichment distribution had concentration of metals at a depth higher than values obtained from layers above and below it under ID wetland. These patterns are primarily related to SOM, hydraulic conditions, and depositional history. Concentrations of non-residual fraction of Cd and Pb (which are good indicators of their bioavailability in a system) may increase with increasing Cd and Pb input in these soils due to their affinity to complex with organic matter. These Cd and Pb complexes can easily be leached out into water bodies or taken up into plant tissues of crops that are cultivated in these wetlands. Therefore, the need to monitor the uptake of these metals by plants in order to reduce the potential risk to human health becomes important.
Additional information
Funding
Notes on contributors
Ayodele O. Adelana
Ayodele O Adelana is a research scientist and holds a master’s degree in soil physics with emphases on urban soils. He has conducted studies on soil quality and soil conservation.
Gabriel A. Oluwatosin
Gabriel A Oluwatosin is a research professor and a land evaluation expert who has published in various international and local journals on various aspect of soil science.
Celinah Agunbiade
Celinah Agunbiade is a graduate student.
Kayode S. Are
Kayode S Are is a research scientist specializing in soil conservation. He has conducted studies on remediation of polluted and erosion affected soils.
Olateju D. Adeyolanu
Olateju D Adeyolanu is a soil survey expert with emphases on soil quality research. This study is very important in the area of risk assessment because wetlands are put to intensive cultivation, especially for vegetables in Nigeria. If these soils are contaminated with heavy metals, there is a risk of transfer to humans when such areas are cultivated.
References
- Aiyelari, E. A., & Oshunsanya, S. O. (2008). Preliminary studies of soil erosion in a valley bottom in Ibadan under some tillage practices. Global Journal of Agricultural Sciences, 7, 221–228.
- Binns, J. A., Maconachie, R. A., & Tanko, A. I. (2003). Water, land and health in urban and peri-urban food production: The case of Kano, Nigeria. Land Degradation and Development, 14, 431–444.10.1002/(ISSN)1099-145X
- Christine, G., Sylvaine, T., & Michel, A. (2002). Fractionation studies of trace elements in contaminated soils and sediments: A review of sequential extraction procedures. Trends in Analytical Chemistry, 21, 451–467.
- Clozel, B., Ruban, V., Durand, C., & Conil, P. (2006). Origin and mobility of heavy metals in contaminated sediments from retention and infiltration ponds. Applied Geochemistry, 21, 1781–1798.10.1016/j.apgeochem.2006.06.017
- Coffin, D. E. (1963). A method for determination of free iron oxides in soils and clays. Canadian Journal of Soil Science, 43, 9–17.
- Cui, B., Yang, Q., Yang, Z., & Zhang, K. (2009). Evaluating the ecological performance of wetland restoration in the Yellow River Delta, China. Ecological Engineering, 35, 1090–1103.10.1016/j.ecoleng.2009.03.022
- Du Laing, G., Vandecasteele, B., De Grauwe, P., Moors, W., Lesage, E., Meers, E., …, Verloo, M. G. (2007). Factors affecting metal concentrations in the upper sediment layer of intertidal reedbeds along the river Scheldt. Journal of Environmental Monitoring, 9, 449–455.10.1039/b618772b
- Du Laing, G., Meers, E., Dewispelaere, M., Rinklebe, J., Vandecasteele, B., Verloo, M. G., & Tack, F. M. G. (2009). Effect of water table level on metal mobility at different depths in wetland soils of the Scheldt estuary (Belgium). Water Air Soil Pollution, 202, 353–367.10.1007/s11270-009-9982-2
- Du Laing, G., Rinklebe, J., Vandecasteele, B., Meers, E., & Tack, F. M. G. (2009). Trace metal behaviour in estuarine and riverine floodplain soils and sediments: A review. Science of The Total Environment, 407, 3972–3985.10.1016/j.scitotenv.2008.07.025
- Ferraz, M. C. M., & Lourençlo, J. C. N. (2000). The influence of organic matter content of contaminated soils on the leaching rate of heavy metals. Environmental Progress, 19, 53–58.10.1002/(ISSN)1547-5921
- Gee, G. W., & Or, D. (2002). Particle size analysis. In J. H. Dane & G. C. Topp (Eds.), Methods of soil analysis, Part 4, physical methods (pp. 255–294). Madison, WI: Soil Science Society of America.
- Giani, L., Ahrens, V., Duntze, O., & Irmer, S. K. (2003). Geo-pedogenesis of salic fluvisols on the North Sea Island of Spiekeroog. Journal of Plant Nutrition and Soil Science, 166, 370–378.10.1002/jpln.200390057
- Grossman, R. B., & Reinsch, T. G. (2002). Bulk density and linear extensibility: Core method. In J. H. Dane & G. C. Topp (Eds.), Methods of Soil Analysis, Part 4, Physical Methods (pp. 208–228). Madison, WI: Soil Science Society of America.
- Hendershot, W. H., & Lavkulich, L. M. (1983). Effect of sesquioxide coating on surface charge of standard minerals and soil samples. Soil Science Society of America Journal, 47, 1252–1260.10.2136/sssaj1983.03615995004700060037x
- Ip, C. C. M., Li, X., Zhang, G., Wai, O. W. H., & Li, Y. (2007). Trace metal distribution in sediments of the Pearl River Estuary and the surrounding coastal area, South China. Environmental Pollution, 147, 311–323.10.1016/j.envpol.2006.06.028
- Krishnamurti, G. S. R., & Naidu, R. (2000). Speciation and phytoavailability of cadmium in selected surface soils of South Australia. Australian Journal of Soil Research, 38, 991–1004.10.1071/SR99129
- Lowery, B., Arshad, M.A., Lal, R., & Hickey, W.J. 1996. Soil water parameters and soil quality. In: J.W. Doran & A.J. Jones (Eds.), Methods for assessing soil quality, (pp. 143–157). Madison, WI: Soil Science Society of America Special Publication 49.
- May, P. A., & Edwards, G. S. (2001). Comparison of heavy metal accumulation in a natural wetland and constructed wetlands receiving acid mine drainage. Ecological Engineering, 16, 487–500.
- Mbabazi, J., Bakyayita, G., Wasswa, J., Muwanga, A., Twinomuhwezi, H., & Kwetegyeka, J. (2010). Variations in the contents of heavy metals in arable soils of a major urban wetland inlet drainage system of Lake Victoria, Uganda. Lakes & reservoirs: Research and management, 15, 89–99.
- Ngole, V. M. (2011). Using soil heavy metal enrichment and mobility factors to determine potential uptake by vegetables. Plant Soil Environment, 57, 75–80.
- Ogban, P. I., & Babalola, O. (2009). Characteristics, classification and management of inland valley bottom soils for crop production in sub-humid southwestern Nigeria. Journal of Tropical Agriculture, Food, Environment and Extension, 8, 1–13.
- Okalebo, J. R., Gathua, K. W., & Woomer, P. L. (1993). Laboratory methods of soil and plant analysis: A working manual. Nairobi: TSBF-UNESCO, EPZ.
- Okusami, T. A., & Rust, R. H. (1992). Occurrence, characteristics and classification of some hydromorphic soils from south Nigeria. In J. M. Kimble (Ed.), Characterization, classification and utilization of wet soils (pp. 185–197). USDA, Soil Conservation Service: Proceedings VIII ISCOM.
- Olaleye, A. O., Ogunkunle, A. O., & Sahrawat, K. L. (2000). Forms and pedogenic distribution of extractable iron in selected wetland soils in Nigeria. Communications in Soil Science and Plant Analysis, 31, 923–941.10.1080/00103620009370488
- Oluwatosin, G. A., Adeyolanu, O. D., Dauda, O. T., & Akinbola, G. E. (2008). Level and geochemical fractions of Pb, Cd and Zn in valley bottom soils of some urban cities in south west Nigeria. African Journal of Biotechnology, 7, 3455–3465.
- Oluwatosin, G. A., Adeoyolanu, O. D., Ojo, A. O., Are, K. S., Dauda, T. O., & Aduramigba-Modupe, V. O. (2010). Heavy metal uptake and accumulation by edible leafy vegetable (Amaranthus hybridus L.) grown on urban valley bottom soils in southwestern Nigeria. Soil and Sediment Contamination: An International Journal, 19, 1–20.
- Pagnanelli, F., Moscardini, E., Giuliano, V., & Toro, L. (2004). Sequential extraction of heavy metals in river sediments of an abandoned pyrite mining area: Pollution detection and affinity series. Environmental Pollution, 132, 189–201.10.1016/j.envpol.2004.05.002
- SAS Institute. 2002. SAS/STAT user’s guide, version 8.2. Cary, NC: SAS Institute .
- Schwertmann, U. (1964). The differentiation of iron oxide in soils by a photochemical extraction with acid ammonium oxalate. Zeitschrift für Pflanzenern. Bodenkunde, 105, 194–202.10.1002/(ISSN)1522-2624
- Vandecasteele, B., Du Laing, G., & Tack, F. M. G. (2007). Effect of submergence-emergence sequence and organic matter or aluminosilicate amendment on metal uptake by woody wetland plant species from contaminated sediments. Environmental Pollution, 145, 329–338.10.1016/j.envpol.2006.03.003
- Wan, Z., Song, C., Guo, Y., Wang, L., & Huang, J. (2008). Effects of water gradients on soil enzyme activity and active organic carbon composition under Carex lasiocarpa marsh. Acta Ecologica Sinica, 28, 5980–5986.
- Weil, R. R., Islam, K. R., Stine, M. A., Gruver, J. B., & Samson-Liebig, S. E. (2003). Estimating active carbon for soil quality assessment: A simplified method for laboratory and field use. American Journal of Alternative Agriculture, 18, 1–15.