Abstract
The enzymatic transesterification of docosahexaenoic acid (DHA) ethyl ester with glycerol was performed with several lipases in a solvent-free system and it involves the initial formation of sn-2 docosahexaenyl monoacylglyceride. This DHA derivative is highly relevant for improving the bioavailability of DHA and it has received increasing interest in the field of nutrition. Three commercial lipases, from Rhizomucor miehei (RML), Alcaligenes sp (QL), and Candida antarctica-fraction B (CALB) were tested. In certain cases (CALB), using an excess of DHA ethyl ester and high temperatures the transesterification reaction continues to the formation of triacylglycerides, but in other cases, sn-2 monoacylglyceride (2-MG) is the unique synthetic product even in the presence of high concentrations of DHA ethyl ester. At low temperatures (e.g. 37°C), RML derivatives synthesize only 2-MG in 15 min. These very mild conditions are very interesting for the thermal oxidative stability of the omega-3 fatty acid and for the thermal stability of the biocatalyst. Using Normal Phase HPLC-ELSD and accurate markers, the formation of the 2-MG was confirmed.
Public Interest Statement
Omega-3 fatty acids (PUFAS) (docosahexaenoic acid (DHA) and eicosapentaenoic acid (EPA)) exhibit interesting health benefits: development of brain, prevention of cardiovascular diseases, etc. They cannot be synthesized by humans but they are present in fish oils. The consumption of fish oil is not common in all countries and cultures. The preparation of food additives highly enriched in PUFAs is very important. These food additives could be supplied with a variety of foods (bread, milk, etc.). Different derivatives of PUFAS have very different bioavailabilities: free acids, ethyl esters, triglycerides, monoglycerides, phospholipids, etc. A number of chemical processes are necessary to transform fish oil into the best derivatives. The use of enzymes to catalyze that synthesis is better than the use of conventional chemical processes. We describe a rapid protocol to produce sn-2 monoglycerides (a very relevant formulation) under mild conditions catalyzed by a lipase from Rhizomucor miehie.
Competing Interests
The authors declare no competing interest.
1. Introduction
Omega-3 polyunsaturated fatty acids (ω-3 PUFAs) have been recognized to have an important role in human health (Chang & Deckelbaum, Citation2013; Houston, Citation2005; Kelly, Gilman, Kim, & Ilich, Citation2013; Kris-Etherton, Harris, & Appel, Citation2002). DHA is an omega-3 fatty acid that is required for the normal development of the mammalian nervous and visual systems and is the most important omega-3 long-chain polyunsaturated fatty acid of the brain phospholipids (Horrocks & Yeo, Citation1999; Makrides, Neumann, Byard, Simmer, & Gibson, Citation1994). DHA is initially provided to developing humans by the mother during pregnancy and the lactation period. It can also provide a pure fatty acid (DHA), ethyl ester derivative (DHA-EE), triglyceride (TG), and sn-2 docosahexaenyl monoacylglyceride (DHA-MG) obtained from fish oil (Lawson & Hughes, Citation1998). sn-2 fatty acid monoacylglycerides have been reported to be more easily absorbed by the intestine than other fatty acid derivatives. In rats, DHA-MG supplementation has been found to generate higher plasma and erythrocyte DHA contents than DHA-EE supplementation (Valenzuela, Nieto, Sanhueza, Nuñez, & Ferrer, Citation2005).
New health benefits of monoacylglycerols or monoacyglycerides (MG) are being investigated (Feltes, de Oliveira, Block, & Ninow, Citation2013). Furthermore, sn-2 monoacylglyceride (2-MG) has key roles in complex metabolic processes that control food intake and in inflammatory processes. In recent years, considerable research has been conducted on 2-MG because its metabolism is involved in the synthesis and degradation of endocannabinoids that resemble other lipid transmitters (D’Addario et al., Citation2014; Massodi, Kuda, Rossmeisl, Flachs, & Kopecky, Citation2015).
Many applications for monoglycerides containing DHA are expected to be developed. They are expected to be incorporated into functional foods, cosmetics, and dietary supplements and to be used as ingredients in pharmaceuticals (Feltes et al., Citation2013).
Furthermore, DHA-MG is a good substrate for the preparation of triglycerides with interesting structures that may have nutritional and pharmacological applications, especially TGs with a long-chain polyunsaturated fatty acid occupying the sn-2 position, such as DHA.
The preparation of DHA-MG has been reported by the following methods: treatment of fish oil with region-specific 1,3 lipases, enzymatic alcoholysis of fish oils and glycerolysis of tuna oil from Pseudomonas fluorescence with lipase AK (Esteban et al., Citation2009; Pawongrat, Xu, & H-Kittikun, Citation2007). The glycerolysis system using an immobilized lipase generates a hydrophobic oil phase, hydrophilic glycerol phase, and solid enzyme phase. One significant drawback of this type of system is the low miscibility of the substrates (glycerol and oil) (Esteban et al., Citation2009). In our laboratory, we also observed the formation of DHA-MG as an interesting byproduct of the transesterification of DHA-EE with glycerol for the preparation of DHA-TG (Moreno-Perez, Luna, Señorans, Guisan, & Fernandez-Lorente, Citation2015).
In this paper, the synthesis of sn-2 DHA-MG through the enzymatic transesterification of DHA-EE with glycerol at low temperatures in solvent-free system is reported. DHA-EE can be easily obtained by ethanolysis of fish oil followed by purification (Moreno-Pérez, Guisan, & Fernandez-Lorente, Citation2014; Salcedo-Sandoval et al., Citation2015). This new synthetic approach was tested for the synthesis of multiple lipases, and yields and reaction rates were compared for the different lipases synthesized. The formation of sn-2 monoglycerides was demonstrated.
2. Materials and methods
2.1. Materials
Triton® X-100 (TX), p-nitrophenyl butyrate (p-NPB), ethanol, and molecular sieves (3A size 1/16 in) were from Sigma Chemical Co. (St. Louis, USA). Docosahexaenoic acid ethyl ester (DHA-EE) was from BiotecBTSA. Sepabeads-C18 was kindly donated by Resindion S.R.L. Lipase form Rhizomucor mihei (RML) was generously donated by Novo Nordisk (Denmark). Lipase from Alcaligenes sp. (QL) was obtained from Meito Sangyo Co., Ltd. (Tokyo, Japan). Other reagents and solvents used were of analytical grade.
2.2. Methods
2.2.1. Determination of hydrolytic activity of different soluble and immobilized lipases
Activity assay was performed by measuring the increase in the absorbance at 348 nm (∈ = 5.150 M−1 cm−1) produced by the released p-nitrophenol (pNP) in the hydrolysis of 0.4 mM p-NPB in 25 mM sodium phosphate at pH 7 and 25°C, using a spectrophotometer with a thermostatized cell and with continuous magnetic stirring. To initialize the reaction, 0.05–0.2 mL of the soluble lipases (blank or supernatant) and their immobilized preparations (suspension) were added to 2.5 mL of substrate solution. Enzymatic activity was calculated as μmols of hydrolyzed p-NPB per minute per mg of enzyme (IU) under the conditions described above.
2.2.2. Immobilization of lipases on sepabeads-C18 resins
The immobilization was performed by adding 6 ml of resin to 36 ml of 10 mM sodium phosphate, pH 7.0 containing 5 mg of each lipase (Palomo et al., Citation2002). In each case, a reference suspension was prepared where the activated support (Sepabeads-C18 resin) was substituted by inert agarose. In all cases, the activity of this reference suspension showed 100% of the activity associated with the supernatant, indicating that no lipase was non-specifically adsorbed to the support. Immobilization yield was defined as the percentage of lipase (determined by the activity p-NPP) immobilized on the different supports.
2.2.3. Drying of lipase derivatives
Organic solvents were dried by incubation overnight in the presence of a high excess of dry molecular sieves: 500 g of molecular sieves (3A size 1/16 in. from Sigma–Aldrich (San Luis, Mo, USA)) per liter of solvent. Then, 5 g of lipase derivative was suspended in 50 ml of dry solvent in the presence of 20 g of dried molecular sieves for 3 h. In this manner, the water filling the pores of the derivatives was dissolved and diluted into the organic solvent and then adsorbed on the sieves.
2.2.4. Enzymatic transesterification of glycerol with DHA-EE
For the typical experiment, glycerol (0.115 g = 0.00124 mol), DHA-EE (6 g = 0.017 mol), and 1 g of derivative lipase were placed in 25-mL glass vials fixed in a shaking thermostated bath. The glass vials were shaken lengthwise at approximately 200 rpm. The esterification was performed under vacuum in order to remove the ethanol formed during the reaction.
To determine the time course of the reaction, the medium was regularly analyzed. Samples of 20 microliters were diluted in 1 mL of hexane and analyzed by chromatographic techniques.
2.2.5. HPLC-ELSD analysis
The HPLC-ELSD analysis was performed using an Agilent 1260 Infinity HPLC system equipped with a ELDS Agilent 385 (Palo Alto, CA, USA) instrument.
The chromatographic separation of triglycerides, diglycerides, and monoglycerides was performed with a silica normal-phase ACE (25 × 4.6 mm i.d., 0.5 μm) column maintained at 30°C using a ternary gradient as follows: 0–3 min, 99.5% A and 0.5% B; at t = 5 min, 70% A and 30% B; at t = 9 min, 70% A and 30% B; at t = 10 min, 56% A, 24% B and 20% C; at t = 16 min, 56% A, 24% B and 20% C; at t = 17 min, 99.5% A and 0.5% B; at t = 20 min, 99.5% A and 0.5% B. Eluent A was isooctane; eluent B was methyl tert-butyl ether; and eluent C was 2-propanol. The flow rate was 2 mL/min. The best signal and resolution were achieved at the following ELSD conditions: evaporator temperature = 35°C, nebulizer temperature = 35°C; and evaporator gas N2 = 1.6 SLM.
2.2.6. Re-use of Sepabeads-RML under optimal conditions
Synthesis of DHA-MG was carried out as described in methods. After 15 min at 37°C, a 98% of sn-2 DHA-MG was obtained. The reaction mixture was filtered, the biocatalyst was dried again (as described above) and it was used in a new reaction cycle. Ten consecutive reaction cycles were performed.
3. Results and discussion
3.1. Transesterification of DHA-EE with different lipase derivatives
The synthetic properties of immobilized CALB are different at different temperatures (Table ). At 37°C, the biocatalyst synthesized DHA-MG with a 90% and a low percentage of 10% DHA-TG. At 50°C, the inverse situation occurs: the reaction synthesizes 82% DHA-TG and 18% DHA-MG is obtained as a byproduct. The main product and the byproduct are very interesting, and they (a monoglyceride and a triglyceride) should be easily separated (e.g. by chromatography, by molecular distillation). At higher temperatures (e.g. 60°C), the reaction is more complex and DHA can undergo thermal oxidation.
Table 1. Effect of temperature on the synthetic yields in monoglycerides and in triglycerides catalyzed by immobilized CALB. Experimental conditions are described in methods
Immobilized QL catalyzed the synthesis of sn-2 DHA-MG at 50°C. At the first stages of the reaction, a 95% yield of MG was obtained. This yield decreased slowly with the simultaneous increase in the synthesis of TG.
However, immobilized RML catalyzed the synthesis of DHA-MG at 37°C with a 98% yield. The product was stable, and it was not modified for 24 h (Table ).
Table 2. Synthetic yields in DHA-TG obtained with different lipases: Rhizomucor miehei (RML), Alcaligenes sp (QL), and Candida antarctica-fraction B (CALB) experimental conditions are described in methods
For all reactions, the synthesized monoglyceride was sn-2 DHA-MG, as shown in Figure . The HPLC analysis of a reaction sample and an external marker of sn-1 DHA-MG showed that the sn-2 DHA-MG peak was intact in the reaction sample. The peak is eluted with a retention time corresponding to monoacylglycerols and it does not correspond to the external marker. Thus, the peak should correspond to the sn-2 regioisomer. Similar results were obtained with the CALB and QL biocatalysts. Although RML specifically generates sn-1,3 isomers when catalyzes hydrolytic reactions (Ifeduba & Akoh, Citation2014), now in the transesterification process, RML specifically generates the sn-2 isomer. The mechanisms of hydrolysis and glycerolysis are different, and in glycerolysis the spatial fixation of free glycerol as the acceptor on the enzyme active center is a key event.
Figure 1. Identification of the synthesized monoacylglycerol.
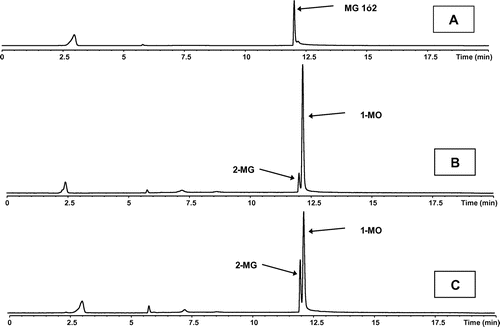
In Table , the behaviors of the three enzymatic biocatalysts are summarized. The three enzymes were all reasonable alternatives for the synthesis of sn-2 DHA-MG.
However, the best results were obtained with immobilized RML, which generated a 98% yield in 15 min at 37°C. This very rapid high yield at low temperatures is very interesting because of the high stability of the biocatalyst and the thermal oxidative stability of the omega-3 fatty acids (Salcedo-Sandoval et al., Citation2015). Other protocols to synthesize DHA-MG have been reported but the experimental conditions are much more drastic. The enzymatic glycerolysis of fish oil in a solvent-free system was feasible, as already stated in the literature for this reaction system. Camino Feltes et al. (Citation2012) have studied all variables proved to be important for MAG production. Temperature proved to be effective in increasing the initial reaction rate for the production of monoacylglycerols. It was possible to produce 25.9% of MAG after 24 h of reaction at 70°C. Fregolente et al. (Citation2008) have reported that lipase CA-IM was the most efficient in producing DG and MG in a solvent-free system through the catalyzed lipase glycerolysis of soybean oil. The maximum production reached, after 24 h of reaction, for DG and MG was 48 and 32%, respectively, at 50°C in solvent free system.
3.2. Re-utilization of immobilized RML
Ten reaction cycles were performed using the same biocatalyst (immobilized RML). In all cases, the reaction rates and synthetic yields were almost identical (98% of product in 15 min), with standard deviations of less than 1%.
4. Conclusions
The synthetic results were notably interesting. Three immobilized lipases were very useful for the production of sn-2 DHA-MG. The best result was obtained with RML adsorbed on a hydrophobic support (SEPABEADS). This biocatalyst catalyzes the synthesis of sn-2 DHA-MG in a solvent-free system and in only 0.25 h at 37°C with a 98% synthetic yield. In addition, this immobilized lipase is very stable. In fact, 10 reaction cycles re-using the same biocatalyst were found to have identical reaction rates and to generate identical yields.
Acknowledgments
We gratefully recognize the Spanish Ministry of Science and Innovation for the “Ramón y Cajal” contract for Dr Fernandez-Lorente and for the FPI contract to Sonia Moreno-Perez. We would like to thank Novozymes and Ramiro Martinez for the generous gift of commercial lipases.
Additional information
Funding
Notes on contributors
Jose M. Guisan
Jose M Guisan is Head of the Laboratory of Enzyme Technology. Enzymes are biological macromolecules are able to catalyze very relevant chemical processes and they exhibit a number of advantages regarding to conventional chemical processes. However, because of their biological origin, enzyme also exhibits important limitations to be used as industrial catalyst: e.g. they are soluble and very unstable. The main goal of our laboratory is the minimization of these limitations by preparing “stone-like” enzymes able to act as “optimal and cost-effective” chemical catalyst. We are very interested in (a) the Biocatalyst Engineering: purification, immobilization, stabilization, and reactivation of very different enzymes (monomeric, multimeric, very large proteins, lipases, etc.) and (b) the design of enzyme processes with interest in all areas of chemical industries: food technology, pharmaceutical and fine chemistry, analytical chemistry (biosensors), energy (production of biodiesel), etc. The present paper deals with the preparation of an optimal lipase catalyst able to produce very relevant functional ingredients in food technology.
References
- Camino Feltes, M. M., Villeneuve, P., Baréa, B., Barouh, N., De Oliveira, J. V., De Oliveira, D., & Ninow, J. L. (2012). Enzymatic production of monoacylglycerols (MAG) and diacylglycerols (DAG) from fish oil in a solvent-free system. JAOCS, 89, 1057–1065.
- Chang, C. L., & Deckelbaum, R. J. (2013). Omega-3 fatty acids. Current Opinion in Lipidology, 24, 345–350.10.1097/MOL.0b013e3283616364
- D’Addario, C., Di Bonaventura, M. M., Pucci, M., Romano, A., Gaetani, S., Ciccocioppo, R., …, Maccarrone, M. (2014). Endocannabinoid signaling and food addiction. Neuroscience & Biobehavioral Reviews, 47, 203–224.
- Esteban, L., del Mar Muñío, M., Robles, A., Hita, E., Jiménez, M. J., González, P. A., …, Molina, E., (2009). Synthesis of 2-monoacylglycerols (2-MAG) by enzymatic alcoholysis of fish oils using different reactor types. Biochemical Engineering Journal, 44, 271–279.10.1016/j.bej.2009.01.004
- Feltes, M. M. C., de Oliveira, D., Block, J. M., & Ninow, J. L. (2013). The production, benefits, and applications of monoacylglycerols and diacylglycerols of nutritional interest. Food and Bioprocess Technology, 6, 17–35.10.1007/s11947-012-0836-3
- Fregolente, P. B., Fregolente, L. V., Pinto, G. M., Batistella, B. C., Wolf-Maciel, M. R., & Maciel Filho, R. (2008). Monoglycerides and diglycerides synthesis in a solvent-free system by lipase-catalyzed glycerolysis. Applied Biochemistry and Biotechnology, 146, 165–172.10.1007/s12010-008-8133-3
- Horrocks, L. A., & Yeo, Y. K. (1999). Health benefits of docosahexaenoic acid (DHA). Pharmacological Research, 40, 211–225.10.1006/phrs.1999.0495
- Houston, M. C. (2005). Nutraceuticals, vitamins, antioxidants, and minerals in the prevention and treatment of hypertension. Progress in Cardiovascular Diseases, 47, 396–449.10.1016/j.pcad.2005.01.004
- Ifeduba, E. A., & Akoh, C. C. (2014). Modification of stearidonic acid soybean oil by immobilized Rhizomucor miehei lipase to incorporate caprylic acid. JAOCS, 91, 953–965.
- Kelly, O. J., Gilman, J. C., Kim, Y., & Ilich, J. Z. (2013). Long-chain polyunsaturated fatty acids may mutually benefit both obesity and osteoporosis. Nutrition Research, 33, 521–533.10.1016/j.nutres.2013.04.012
- Kris-Etherton, P. M., Harris, W. S., & Appel, L. J. (2002). Fish consumption, fish oil, omega-3 fatty acids, and cardiovascular disease. Circulation, 106, 2747–2757.10.1161/01.CIR.0000038493.65177.94
- Lawson, L. D., & Hughes, B. G. (1998). Human absorption of fish oil fatty acids as triacylglycerols, free acids, or ethyl esters. Biochemical and Biophysical Research Communications, 152, 328–335.
- Makrides, M., Neumann, M. A., Byard, R. W., Simmer, K., & Gibson, R. A. (1994). Fatty acid composition of brain, retina, and erythrocytes in breast- and formula-fed infants. American Journal of Clinical Nutrition, 60, 189–194.
- Massodi, M., Kuda, O., Rossmeisl, M., Flachs, P., & Kopecky, J. (2015). Lipid signaling in adipose tissue: Connecting inflammation & metabolism. Biochimica Biophysica Acta. Molecular and Cell Biology of Lipids, 1851, 503–518.
- Moreno-Perez, S., Luna, P., Señorans, F. J., Guisan, J. M., & Fernandez-Lorente, G. (2015). Enzymatic synthesis of triacylglycerols of docosahexaenoic acid: Transesterification of its ethyl esters with glycerol. Food Chemistry, 187, 225–229.10.1016/j.foodchem.2015.04.095
- Moreno-Pérez, S., Guisan, J. M., & Fernandez-Lorente, G. (2014). Selective ethanolysis of fish oil catalyzed by immobilized lipases. JAOCS, 91, 63–69.
- Palomo, J. M., Muñoz, G., Fernández-Lorente, G., Mateo, C., Fernández-Lafuente, R., & Guisán, J. M. (2002). Interfacial adsorption of lipases on very hydrophobic support (octadecyl–Sepabeads): Immobilization, hyperactivation and stabilization of the open form of lipases. Journal of Molecular Catalysis B: Enzymatic, 19–20, 279–286.10.1016/S1381-1177(02)00178-9
- Pawongrat, R., Xu, X., & H-Kittikun, A. (2007). Synthesis of monoacylglycerol rich in polyunsaturated fatty acids from tuna oil with immobilized lipase AK. Food Chemistry, 104, 251–258.10.1016/j.foodchem.2006.11.036
- Salcedo-Sandoval, L., Cofrades, S., Ruiz-Capillas, C., Matalanis, A., McClements, D. J., Decker, E. A., & Jiménez-Colmenero, F. (2015). Oxidative stability of n-3 fatty acids encapsulated in filled hydrogel particles and of pork meat systems containing them. Food Chemistry, 184, 207–213.10.1016/j.foodchem.2015.03.093
- Valenzuela, A., Nieto, S., Sanhueza, J., Nuñez, M. J., & Ferrer, C. (2005). Tissue accretion and milk content of docosahexaenoic acid in female rats after supplementation with different docosahexaenoic acid sources. Annals of Nutrition and Metabolism, 49, 325–332.10.1159/000087337