Abstract
The allotetraploid Brassicales member canola (Brassica napus L.) is an oil-seed crop sought primarily for vegetable oil, animal feed, and biofuel. With the advent of -omics (genomics, transcriptomics, proteomics, and metabolomics) technologies, numerous studies have focused on deciphering the functional genes, proteins, and metabolites in canola. However, the oil-yielding seeds are of commercial interest and only a handful of studies using mass-spectrometry (MS) or spectroscopy based platforms have attempted to characterize and quantify metabolites of the seed. Baring metabolite profiling approaches which study groups of chemicals, metabolomic insights into the seeds are very recent. Canola seeds are enriched in fatty acids, glucosinolates, phenylpropanoids (i.e. sinapoyl cholines), flavonoids, and phytohormones among others as has become evident from MS and spectroscopy-based recent studies. Thus, cataloging of the seed metabolome is essential before gaining further insights into their roles in seed biology and plant physiology in order to further understand the qualitative traits and products obtainable from the seeds.
Public Interest Statement
Brassica napus L., commonly known as canola (rapeseed) belongs to the mustard family of plant kingdom and is an oil-seed crop sought primarily for vegetable oil, animal feed, and biofuel. The oil-yielding seeds are of commercial interest. Hence, recent studies have used tools such as mass-spectrometry or spectroscopy to identify and measure the different small molecules (phytochemicals) in the seeds. Apart from the sulfur-smelling and -containing chemical compound class known as glucosinolates, canola seeds are rich in fatty acids which make them commercially important as an oil crop. In addition, the seeds contain phenolics, flavonoids, sugars, amino acids, and other basic biochemicals usually present in a seed. Thus, cataloging of the seed metabolome is important for understanding its role in seed biology, physiology, and agriculture, before maneuvering the agronomic traits.
Competing Interests
The author declares no competing interest.
1. The evolving-omics world of canola
Oilseed rape or canola (Brassica napus L, family: Brassicaceae) is a recently formed allotetraploid, an important crop plant primarily grown for seed oil production. B. napus (AACC, 2n = 38) is an allopolyploid crossing between its parents Brassica rapa (AA, 2n = 20) and Brassica oleracea (CC, 2n = 18). The oil composition of the two oilseed species is highly similar and their oils are typically mixed and sold as one product, i.e. rapeseed oil. Being a parallel to the model plant Arabidopsis thaliana, canola has enjoyed deriving enormous comparative genomics resources from the later, hence rendered as a model oil crop species for research and development. Accordingly, canola seeds are used for the production of animal feed, vegetable oil, and biodiesel, whereas the canola seed meal as a byproduct contains the highest quality of proteins in terms of nutritive value, antigenicity, and amino acid composition (Pan, Jiang, & Pan, Citation2011). Seed residues left after pressing, i.e. seed meal, is of great interest for industry as animal feed and for human nutrition. However, the meal is enriched in fibers, polyphenols, phytic acid, and other antinutritive principles which decreases the meals market value.
Given that the seed is the key product of most crop species, its development has been intensively investigated across model species and many plant genera (De Smet, Lau, Mayer, & Jürgens, Citation2010; Moles et al., Citation2005; North et al., Citation2010). Moreover, the genetic (North et al., Citation2010), molecular (Finkelstein, Reeves, Ariizumi, & Steber, Citation2008; Holdsworth, Bentsink, & Soppe, Citation2008), and biochemical (Bailly, Citation2004) processes underlying a seed’s life history are somewhat understood to the extent of allowing insights into basic phenomena such as seed dormancy and germination (Koornneef, Bentsink, & Hilhorst, Citation2002). However, the realization of -omics approaches to the understanding of seed development and physiology are limited till date with few exceptions (Joët, Wurtzel, Matsuda, Saito, & Dussert, Citation2012; Miernyk & Johnston, Citation2012; Nambara & Nonogaki, Citation2012). Consistent with the growth and realization of tremendous potentials of -omics research in plant sciences (Provart et al., Citation2015), efforts in cataloging of the available -omics data sets for canola seeds are non-existent. Towards this end, the information on seed genome, transcriptome, proteome, and metabolome are of critical interest.
With the advent of the -omics (genomics, transcriptomics, proteomics, metabolomics) technologies in the past few decades, a lot of studies have focused on the genome and transcriptome of canola to address topics ranging from basic genome structure and ploidy studies to organellar genomes and gene expression studies of plants or plant-organs subjected to a plethora of biotic and abiotic conditions. These studies have focused on small RNAs (Buhtz, Springer, Chappell, Baulcombe, & Kehr, Citation2008; Zhao et al., Citation2012), microRNAs (Zhou, Song, & Yang, Citation2012), single nucleotide polymorphisms (SNPs)(Delourme et al., Citation2013; Raman et al., Citation2014; Trick, Long, Meng, & Bancroft, Citation2009), ploidy status (Gaeta, Pires, Iniguez-Luy, Leon, & Osborn, Citation2007; Harper et al., Citation2012; Higgins, Magusin, Trick, Fraser, & Bancroft, Citation2012; Parkin, Sharpe, Keith, & Lydiate, Citation1995), mitochondrial genome (Handa, Citation2003), genotyping (Bus, Hecht, Huettel, Reinhardt, & Stich, Citation2012), traits (Harper et al., Citation2012), quantitative trait loci (Jiang et al., Citation2014), genome balance (Xiong, Gaeta, & Pires, Citation2011), sub-genomic selection pattern (Qian, Qian, & Snowdon, Citation2014), linkage maps (CitationWang, Lydiate, et al., 2011), and genome structure (Li, Gao, Yang, & Quiros, Citation2003; Parkin et al., Citation2005; Xu, Zhong, Wu, Fang, & Wang, Citation2009). Gene expression studies have been performed in secondary cell-wall-enriched seeds (Jiang & Deyholos, Citation2010), imbibed seeds (Li, Wu, Tsang, & Cutler, Citation2005), responses to fungal pathogen (Yang, Srivastava, Deyholos, & Kav, Citation2007), and cytoplasmic male sterile flowers by microarray analysis (Carlsson et al., Citation2007), among others(Körber et al., Citation2015; Marmagne, Brabant, Thiellement, & Alix, Citation2010).
Similarly, studies in proteomics of B. napus have cataloged proteins expressed in xylem (Kehr, Buhtz, & Giavalisco, Citation2005) and phloem sap (Giavalisco, Kapitza, Kolasa, Buhtz, & Kehr, Citation2006), apoplast during infection (Floerl et al., Citation2008), anthers (Sheoran & Sawhney, Citation2010), leaf, stem and root (Albertin et al., Citation2009), roots in response to infection (Cao et al., Citation2008), boron deficiency (Wang, Wang, Shi, Wang, & Xu, Citation2010), oxalic acid-mediated stress responses (Liang, Strelkov, & Kav, Citation2009), extracellular secretions of roots (Basu et al., Citation2006), response to low phosphorous (Yao, Sun, Xu, Zhang, & Liu, Citation2011) and drought stress (Koh et al., Citation2015), plastids (Jain, Katavic, Agrawal, Guzov, & Thelen, Citation2008), developing embryos and leaves (Demartini, Jain, Agrawal, & Thelen, Citation2011), seed oil bodies (Katavic, Agrawal, Hajduch, Harris, & Thelen, Citation2006), seed filling (Hajduch et al., Citation2006), aged seeds (Yin, He, Gupta, & Yang, Citation2015), germinating seeds (Kubala et al., Citation2015), osmoprimed seeds (Kubala et al., Citation2013), endosperm (Lorenz, Rolletschek, Sunderhaus, & Braun, Citation2014) as well as from single-cell types such as guard cells (Zhu, Dai, McClung, Yan, & Chen, Citation2009; Zhu, Simons, Zhu, Oppenheimer, & Chen, Citation2010; Zhu et al., Citation2012) and mesophyll cells (Zhu et al., Citation2009). Moreover, the recent sequencing of the B. napus genome (Chalhoub et al., Citation2014) and the availability of closely related genome of B. rapa (Wang, Wang, et al., Citation2011) have developed our understanding of the canola growth, development, health, yield among others.
Metabolome is defined as the entire complement of metabolites (i.e. small molecules with molecular weights <2,000 Daltons) obtained from cells, tissues, organs, organisms, fluids, and communities of biological origin. Although, no single platform is capable of covering the diverse chemical classes of small molecules, metabolomic investigations are platform-dependent, and are either MS [gas chromatography-mass spectrometry (GC-MS), liquid chromatography-mass spectrometry (LC-MS), and capillary electrophoresis-mass spectrometry (CE-MS)] or spectroscopy based [nuclear magnetic resonance (NMR), Raman, or Fourier Transform Infrared (FTIR) spectroscopy] (Kim, Choi, & Verpoorte, Citation2011). These approaches may or may not involve chromatographic separations (GC, LC, CE etc.) and are achieved using targeted (Sawada et al., Citation2009) and untargeted (De Vos et al., Citation2007) platforms. Hence, this treatise is to collate all the metabolites found and quantified in canola seeds as a phytoresource for future metabolomics studies.
In contrast, until recently, very little information has been made available on the seed metabolomes from plants such as A. thaliana (Bottcher et al., Citation2008), soybean (Clarke et al., Citation2013; Schmidt et al., Citation2011), pea (Vigeolas et al., Citation2008), tomato (Mounet et al., Citation2007), Nigella species (Farag, Gad, Heiss, & Wessjohann, Citation2014), and rice (Shu, Frank, Shu, & Engel, Citation2008), among others. However, for canola, only a handful attempts (Jahangir et al., Citation2012) were recorded to catalog the seed metabolome, which includes mass-spectrometry (MS)-(Farag, Sharaf Eldin, Kassem, & Abou el Fetouh, Citation2013; Frolov, Henning, Böttcher, Tissier, & Strack, Citation2013) and NMR-based metabolomics of rape seeds (Kortesniemi et al., Citation2015) and seed coats (Jiang et al., Citation2013).
Plant metabolomics applications can range from applications in phytochemistry (Sumner, Mendes, & Dixon, Citation2003), food safety, quality, and traceability (Castro-Puyana & Herrero, Citation2013) to single cell and single cell-type metabolomics studies (Geng et al., Citation2016; Misra, Assmann, & Chen, Citation2014; Misra, Acharya, Granot, Assman, & Chen, Citation2015) and serve as functional genomics tool (Hall et al., Citation2002). These studies can range from explorations of specific classes of chemical compounds using metabolite profiling approaches or widely targeted global approaches. In fact, very recently the normal B. napus guard and mesophyll cell metabolomes from the leaves as well bicarbonate stressed metabolomes (mimicking elevated atmospheric [CO2] response) were cataloged using global high performance liquid chromatography–multiple reaction monitoring- mass spectrometry (HPLC-MRM-MS) and GC-MS approaches (Misra, de Armas, Tong, & Chen, Citation2015). Similarly, previous studies on canola seeds focused on the profiling of smaller groups of compounds such as glucosinolates, phenolics, fatty acids, or sinapoyl cholines using GC-MS, UHPLC-MS, or LC-MS or other chromatographic and chemometric approaches (Farag et al., Citation2013; Frolov et al., Citation2013). With exciting times in metabolomics, the available information is summarized towards the cataloging of the important B. napus seed metabolome.
2. The canola seed metabolome
Till date, only a handful of attempts are recorded which deal with system-wide metabolomic investigations of B. napus seeds (Farag et al., Citation2013; Frolov et al., Citation2013; Jiang et al., Citation2013; Kortesniemi et al., Citation2015; Tan et al., Citation2015). Previous studies used gas-liquid chromatography (GLC) to determine the chemical composition of yellow- and brown-seeded Brassica for quantification of carbohydrates, dietary fiber, and galacto-oligosaccharides in canola seed meal (Simbaya et al., Citation1995; Slominski, Campbell, & Guenter, Citation1994). Remarkably, a sharp increase in metabolomics studies occurred beyond 2012 and the number of studies continues to rise as is evident in current literature. Consequently, the first 13C-NMR based assignment of phytochemicals in seeds coats of B. napus-Sinapis alba hybrids was also performed during this period (Jiang et al., Citation2013). In another recent NMR-based metabolomics study, it was shown that unsaturated fatty acids, sucrose, and sinapine were the most discriminating metabolites between B. napus and B. rapa metabolomes (Kortesniemi et al., Citation2015). This study also recorded the characteristic Fourier transform infrared spectroscopy (FTIR) spectra of critical compounds which provided important leads towards the assured identification of metabolites (classes). However, the most comprehensive metabolomic study of B. napus seeds was performed using non-targeted metabolomic analysis via ultra performance liquid chromatography–quadrupole time-of-flight-mass spectrometry (UPLC-QTOF-MS) where compounds belonging to various chemical classes i.e. oxygenated fatty acids, flavonols, phenolic acids, and sinapoyl choline derivatives were successfully quantified in seeds (Farag et al., Citation2013). In this study, about 20 metabolites were confidently identified in the seeds alongside another four unknown metabolites, as listed in Supplementary Table 1. These metabolites are different from another 44 metabolites which were differentially distributed in root, stem, and inflorescence but were not detected in seeds. Another study in developing canola seeds, where global metabolomics explorations were performed using GC-MS, claimed to detect almost 443 features, but could correctly annotate and quantify 77 metabolites which include a very informative list of amino acids, fatty acids, organic acids, phenolic acids, carbohydrates (sugars) among others (Tan et al., Citation2015). The list is presented as Supplementary Table 1. In another interesting study, to highlight metabolic control, the genotypic differences in carbon partitioning for in vitro cultured developing embryos of oilseed rape resulted in recording of 79 net fluxes, the levels of 77 metabolites, and 26 enzyme activities with specific focus on central metabolism in nine selected germplasm accessions (Schwender et al., Citation2015).
In addition, a lot of information on individual metabolites are derived from focused profiling approaches where the following major metabolite groups have been well characterized and individual metabolites quantified. A list of 160 total metabolites were collected (Supplementary Table 1) which were mapped against different chemical databases to obtain 110 Kyoto Encyclopedia of Genes and Genomes (KEGG) IDs. These KEGG IDs were then connected based on their biochemical interactions and relatedness at STITCH 5.0 available at http://stitch.embl.de/ (Szklarczyk et al., Citation2016) as shown in Figure . For a more classical view of KEGG pathways, the metabolites were also mapped against the KEGG metabolic map (http://www.genome.jp/kegg/mapper.html) as shown in Figure . In fact, with the KEGG IDs provided, users can create both the images in an interactive fashion to add or remove metabolites. It is also to be noted that the network and pathway mapping were based only on the above discussed metabolomics efforts, as not all metabolites (like many flavonoids and sinapines) are mappable in public databases, and it did not take into account each individual metabolite after removing the redundant ones from individual publications. Moreover, the networks and the pathways are used for visual interpretation of the canola seed metabolome for comprehensiveness and not completeness.
Figure 1. Groups of metabolites and their possible relatedness in B. napus seeds as inferred from STITCH 5.0 (Szklarczyk et al., Citation2016) small molecule database mining of total 160 metabolites of which 110 could be assigned to KEGG IDs. For the compound list please refer to Supplementary Table 1.
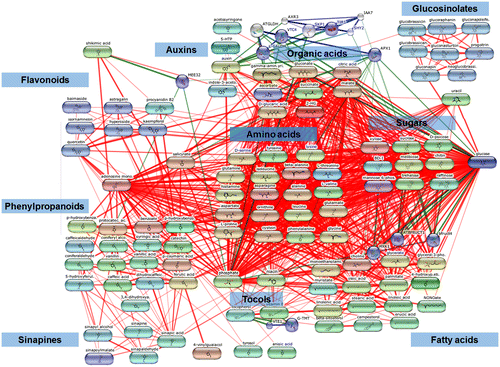
3. Glucosinolates
Glucosinolates are sulfur-containing metabolites characteristic to Brassicales (Fahey, Zalcmann, & Talalay, Citation2001). Biosynthesis of aliphatic and indolic glucosinolates is well understood (Halkier & Du, Citation1997). Glucosinolate contents in the seeds are affected by nutrients such as nitrogen and sulpfur (Zhao, Evans, Bilsborrow, & Syers, Citation1994). Further, glucosinolates and their derivatives were proposed as potential allelochemicals of the crop’s residue (Gardiner, Morra, Eberlein, Brown, & Borek, Citation1999). Eleven glucosinolates were detected and quantified in several B. napus cultivars by HPLC using both photodiode array and electrospray ionization mass spectrometry detection (LC-PAD-ESI/MSn) methods (Velasco, Soengas, Vilar, Cartea, & del Rio, Citation2008; Velasco et al., Citation2011) as shown in Supplementary Table 1. Further, glucosinolate content are known to increase by drought stress in B. napus, while the seed oil content remained unchanged (Jensen et al., Citation1996). These glucosinolates in seeds are involved in protection against pathogens, herbivores, and pests, among others.
4. Flavonoids
Flavonoid diversity in canola is well studied (Auger et al., Citation2010; Velasco et al., Citation2011). Flavonoids such as isorhamnetin, isorhamnetin-3,7-O-β-D-diglucopyranoside, isorhamnetin dihexoside (Auger et al., Citation2010), flavonol glycosides (Auger et al., Citation2010; Yokozawa et al., Citation2002) are reported in canola. Flavonoid extracts from canola seed coat and leaf analyzed using HPLC coupled with diode array detection and mass spectrometry (HPLC-PDA-MS) revealed a total of 22 different flavonoids (Auger et al., Citation2010; Velasco et al., Citation2011). Using HPLC-PDA-ESI (−)-MSn/(high resolution) HRMS analysis, a 91 flavonoids and hydroxycinnamic acid derivatives were detected i.e. 39 kaempferol derivatives, 11 isorhamnetin derivatives, five quercetin derivatives, six flavanols and their oligomers, and 30 hydroxycinnamic acid derivatives of which 78 were tentatively identified, and 24 were detected for the first time in the genus Brassica (Shao et al., Citation2014). Furthermore, analysis of seed coats by LC-UV-MS analysis revealed the presence of quercetin-3-glucoside, [DP2]-B2, epicatechin-(4β-8)-epicatechin, epicatechin, kaempferol-3-O-glucoside-7-O-glucoside, and isorhamnetin-dihexoside (Zhang et al., Citation2013). Although, a lot of flavonoids have been identified and quantified in the canola seeds, the function of individual flavonoid in the seed’s life history is far from established.
5. Phenylpropanoids
Phenylpropanoids (phenolics) constitute a large class of metabolites in plants involved in defense and protection against pathogen attacks, high light intensity and UV rays, wounding, and low nutrient conditions (Dixon & Paiva, Citation1995; Hahlbrock & Scheel, Citation1989; Yu & Jez, Citation2008). In one study, a total phenolic choline ester fractions prepared from canola seeds were analyzed by capillary LC/ESI-QTOF-MS and direct infusion electrospray ionization-Fourier transform ion cyclotron resonance-mass spectrometry (ESI-FTICR-MS) (Böttcher, von Roepenack-Lahaye, Schmidt, Clemens, & Scheel, Citation2009). In addition to the dominating sinapoylcholine, 30 phenolic choline esters were identified based on accurate mass measurements. Some of the compounds identified included substituted hydroxycinnamoyl- and hydroxybenzoylcholines, respective monohexosides, and other oxidative coupling products of phenolic choline esters and monolignol.
Using liquid chromatography-tandem mass spectrometry (LC-MS/MS)-based analysis, sixty-six cell wall-bound phenolics and their derivatives were identified and quantified (Frolov et al., Citation2013). The analysis was performed by an electrospray ionization- liquid chromatography/quadrupole linear ion trap mass spectrometer (ESI-QqLIT) operating in negative ion mode and coupled on-line to an reverse phase ultra performance liquid chromatography (RP-UPLC) system, where the compounds identified are listed in Supplementary Table 1. Although, the functions of the reported common phenylpropanoids are well known, the collection of these unique combination of phenolics alongside a rich repertoire of flavonoids is yet to be deciphered.
6. Sinapines
In addition to the phenylpropanoids (phenolics), a specialized group of phenolics are one of the most abundant classes of metabolites in canola seeds. These particular sinapate esters have kept the research community interested in canola seeds as they accumulate complex patterns of sinapate esters (Baumert et al., Citation2005). Sinapine and sinapic acid can be discriminated from other phenolics in canola seed and meal using HPLC methods developed (Cai & Arntfield, Citation2001). Additionally, 15 canola seed constituents were isolated and identified by a combination of high-field NMR spectroscopy and high resolution-electrospray ionization-mass spectrometry (HR-ESI-MS) which included kaempferol glycoside esters as well as sinapine (sinapoylcholine), sinapoylmalate, and an unusual cyclic spermidine amide. A glucose ester (1,6-di-O-sinapoylglucose), two gentiobiose esters (1-O-caffeoylgentiobiose and 1,2,6′-tri-O-sinapoylgentiobiose), and two kaempferol conjugates [4′-(6-O-sinapoylglucoside)-3,7-di-O-glucoside and 3-O-sophoroside-7-O-(2-O-sinapoylglucoside)] were also reported for the very first time in plants. The phenolic compounds, namely sinapic acid derivatives, i.e. sinapine, and sinapoyl glucose, were quantified using HPLC-DAD and LC-MS platforms (Thiyam, Claudia, Jan, & Alfred, Citation2009). The ethylene precursor, 1-aminocyclopropane-1-carboxylic acid (ACC) and ACC-related compounds, α- and γ-aminobutyric acids, both known to stimulate ethylene production, were measured in the canola seed exudate and tissues using HPLC methods (Penrose & Glick, Citation2001).
Sinapoylcholine (i.e. sinapine) content in seeds can typically vary from 3 to 12 mg/g (Zum Felde, Baumert, Strack, Becker, & Möllers, Citation2007). Furthermore, it was also found that sinapine/sinapic acid content can range from 73 to 80% of the total polyphenol content in the seed meals (Obied et al., Citation2013). Although other classes of metabolites are detectable predominantly in specific organs such as roots and leaves, the study found that sinapoyl cholines are present uniquely in seeds (Farag et al., Citation2013). Not just in canola, but sinapoyl choline esters are known to accumulate abundantly in seeds of glucosinolate-producing plants (Böttcher et al., Citation2009). As sinapoyl choline is antinutritive to human and animals, efforts are ongoing to reduce sinapoyl choline amounts in canola seeds by conventional and molecular breeding approaches to establish rapeseed as a source of food-grade protein. In this study, sinapoyl cholines and their esters were tentatively observed. Nonetheless, the biosynthetic pathways and efforts to manipulate the biosynthesis of sinapine and its derivatives keeps phytochemists interested in canola seed development.
7. Fatty acids
Plant oils are mainly comprised of triacylglycerols (TAGs), a major component of the human diet and widely used in the chemical industry (Biermann, Bornscheuer, Meier, Metzger, & Schäfer, Citation2011); TAGs are predominantly synthesized from glycerol-3-P and fatty acids (FAs) in the endoplasmic reticulum of embryo cells. For an oil-seed crop of global importance to industry, commerce, and human health, the oil constituents, i.e. fatty acids, take the center stage. Canola oil is a major reserved energy, and it is possibly degraded via the β-oxidative pathway to generate energy for development prior to the full establishment of photosynthetic independence in the seedling. Thus, high levels of free fatty acids are a more convenient and economical energy source than sucrose, and are used for lipid biosynthesis and stored in seeds in preparation for germination (Tan et al., Citation2015). Accordingly, C16:0, C18:2, C18:3, C20:1, C22:1, C18:1 fatty acids were identified in developing rape seeds (Norton & Harris, Citation1975) for the aforementioned purpose. The major fatty acids as quantified by GC-MS analyses (Schwender et al., Citation2015) are as follows: palmitic acid (16:0), stearic acid (18:0), cis-vaccenic acid (18:1), oleic acid (18:1), octadecenoic acid (C18:1), eicosenoic acid (C20:1), docosenoic acid (C22:1), linoleic acid (18:2), linolenic acid (18:3), arachidic acid (20:0), gondoic acid (20:1), and erucic acid (22:1). Oleic acid is the most abundant fatty acid in canola seed oil, i.e. up to 57% of total oil (El-Beltagi & Mohamed, Citation2010; Richter, Spangenberg, Kreuzer, & Leiber, Citation2010; Teh & Birch, Citation2013). In addition, analysis of 1H NMR spectra of rapeseeds extract by hierarchical cluster analysis revealed the role of erucic acid in discrimination of rapeseeds (Han et al., Citation2015). The commercially used rapeseed oil, a mixture of oils from B. napus and B. rapa, contains a nutritionally optimal omega-6/omega-3 fatty acid ratio and very little saturated fatty acids (Gunstone, Harwood, & Dijkstra, Citation2007). Typically, in rapeseed oil the most abundant fatty acid is 9-octadecenoic acid (18:1n-9, 60%), followed by 9,12-octadecadienoic acid (18:2n-6, 20%) and 9,12,15-octadecatrienoic acid (18:3n-3, 10%). Further, it was also demonstrated that cultivation in cooler regions would have a beneficial effect on their nutritional quality through increased content of 9,12,15-octadecatrienoic acid (18:3n-3) and other stress-induced antioxidant compounds (Vuorinen et al., Citation2014).
Furthermore, the mean contents of brassicasterol, campesterol, stigmasterol, β-sitosterol, Δ5-avenasterol, and Δ7-stigmatenol were determined as percentages of total phytosterols in canola oil (Hamama, Bhardwaj, & Starner, Citation2003). Although biosynthesized through unrelated pathways, the vitamin E compounds (tocochromenols) are abundant in canola seeds but are known to protect the plant lipids as antioxidants. In addition, higher anti-oxidant activity in seeds is attributed to α-tocopherol present in seeds (El-Beltagi & Mohamed, Citation2010; Tuberoso, Kowalczyk, Sarritzu, & Cabras, Citation2007). A plethora of tocols, i.e. alpha-tocopherol, alpha-tocotrienol, beta-tocopherol, gamma-tocopherol, beta-tocotrienol, gamma-tocotrienol, delta-tocopherol, and delta-tocotrienol, were quantified in canola seed oils (Oraby & Ramadan, Citation2014). These tocols indicate their roles in protection from oxidative stress as well as source of commercial exploits. As fatty acids and the lipophilic constituents are major constituents of canola seed oil, any transgenic manipulation directed towards the seed oil content must take care as to not negatively modify the balance in other metabolic pathways.
8. Other metabolites of importance
Phytohormones are important in seed dehiscence, maturation, germination, and development. Ethylene precursor, 1-aminocyclopropane-1-carboxylic acid (ACC) and ACC-related compounds, α- and γ-aminobutyric acids, both known to stimulate ethylene production, were measured in the canola seed exudate and tissues using HPLC methods (Penrose & Glick, Citation2001). Among the most thoroughly studied classes are abscisic acid (ABA) in relation to imbibition and seed germination. Although, ABA is considered the bioactive form, 7,8, and 9-hydroxy ABA also displayed hormonal activity in B. napus embryos (Jadhav et al., Citation2008). Gibberellins such as GA1 and GA4 and auxins such as indole-3-acetic acid (IAA) were quantified in canola seeds (Walton et al., Citation2012). The ethylene precursor, 1-aminocyclopropane-1-carboxylic acid (ACC) and ACC-related compounds, α- and γ-aminobutyric acids, both known to stimulate ethylene production, were measured in the canola seed exudate and tissues using HPLC methods (Penrose & Glick, Citation2001).
Previous studies have also concluded that the photosynthesis in the silique wall may contribute the driest matter to ripening seeds, with the contents of sucrose, fructose, and glucose specifically affecting the seed oil content (King, Lunn, & Furbank, Citation1997). Sugars like glucose and fructose were identified in developing rape seeds (Norton & Harris, Citation1975). Primary metabolites, for example, 15 common amino acids, were quantified in B. napus seed meal (Jiang et al., Citation2015). Among other classes of metabolites, sugar alcohols such as inositol accumulation patterns in developing canola seeds indicated that early stages of seed development are marked by rapid deployment of inositol into a variety of pathways, such as polar inositol phosphates and non-polar phospholipids (Dong et al., Citation2013). This study also quantified the galactose, galactinol, raffinose, stachyose, and sucrose levels in seeds. Moreover, hydrolysis of inositol-containing phospholipids is known to lead to increased phytate accumulation in seeds of B. napus (Georges et al., Citation2009). Studies have also quantified glucose, gentiobiose, and a polyamine using NMR and HR-ESI-MS based approaches (Baumert et al., Citation2005).
Carotenoids are a large group of secondary compounds, often highly colored, which are derived from isoprenoid precursors, synthesized in plants, have antioxidant function and in higher plants, and are at times are integral parts of the photosynthetic apparatus (Bartley & Scolnik, Citation1995). Carotenoids such as lutein and beta-carotene are prominent in canola seeds (Ravanello, Ke, Alvarez, Huang, & Shewmaker, Citation2003; Shewmaker, Sheehy, Daley, Colburn, & Ke, Citation1999). Moreover, chlorophyll pigments present in canola seed, meal, and crude and degummed oils analyzed by HPLC revealed that chlorophylls a and b, low levels of pheophytin a, and occasionally traces of pheophorbide, and its methyl ester were present in canola seed. Contrastingly, meals and oils contained magnesium-deficient chlorophyll pigments such as pheophorbide a, methylpheophorbide a, pheophytins a and b, and pyropheophytins a and b but not chlorophyll a or b (Endo, Thorsteinson, & Daun, Citation1992).
9. Future prospects
For an oil-crop, the primary challenges are to achieve higher oil yield, reduced glucosinolate, in addition to lowered secondary metabolites of lesser importance. This renders advances in understanding of lipid biology, biochemistry, and lipidomics very important towards the understanding of seed-omics starting from maturation to germination. To this end, newer technologies available to study plant lipidomes are growing at a burgeoning pace which include the successful implementation of in situ lipidomic visualization/imaging as evidenced with numerous successful examples (Horn & Chapman, Citation2012, Citation2014). Additionally, approaches such as shotgun lipidomics (Han & Gross, Citation2005) as well as advances in hydrophobic interaction liquid chromatography-ion trap-time of flight-mass spectrometry (HILIC-IT-ToF-MS) (Okazaki, Kamide, Hirai, & Saito, Citation2013) and ultra-pressure liquid chromatography-high resolution mass spectrometry (UPLC-HRMS) (Hummel et al., Citation2011) have helped technology platforms move forward at a rapid pace to allow better coverage and quantification. The tools above are not only associated with lipidomic explorations, but are also available to widely targeted and untargeted global metabolomic studies. Even more promising, there has been significant development in the amount, accuracy, performance, availability of databases, software, algorithms, webservers, and tools for analyses of lipidomic (Haimi, Uphoff, Hermansson, & Somerharju, Citation2006) and metabolomic (Misra & van der Hooft, Citation2016) datasets.
10. Conclusions
Canola seeds are enriched in fatty acids, glucosinolates, phenylpropanoids (especially sinapoyl cholines), flavonoids, and phytohormones, among others. With advances in epigenetic studies, transcriptomics, proteomics, and metabolomics platforms and technologies, the possibilities of gaining a deeper understanding of the B. napus systems biology is feasible. In addition, the understanding of dynamic changes of seed metabolic flux is a critical step to increase the quantity and quality of seed oil (Tan et al., Citation2015). Thus, flux models of specific pathways would lead to an understanding of C-flow in germinating embryos (Schwender, Ohlrogge, & Shachar-Hill, Citation2003). However, with the seeds of this oil crop playing important roles in oil economy, as future biofuel for industrial growth among other financial roles, approaches and technologies addressing the enhanced oil content in seeds are of prime importance. To achieve this, metabolomics will play a pivotal role in helping integrate the genotype-derived information from genome, transcriptome, and proteome for understanding of regulatory steps leading to increased oil-yield among other improved traits. Moreover, the cataloged metabolites from this review can be integrated into seed-specific or Brassica-specific metabolite databases for future applications.
List of abbreviations | ||
MS | = | Mass-spectrometry |
NMR | = | Nuclear magnetic resonance spectroscopy |
HPLC | = | High performance liquid chromatography |
LC | = | Liquid chromatography |
GC | = | Gas chromatography |
ESI | = | Electron spray ionization |
UHPLC | = | Ultra-high performance liquid chromatography |
CE | = | Capillary electrophoresis |
Q | = | Quadrupole |
ToF | = | Time-of-flight |
FTIR | = | Fourier transform infrared red |
PAD/DAD | = | Photodiode array detector |
MS/MS | = | Tandem mass spectrometry |
Supplementary material
Supplementary material for this article can be accessed here htttp://dx.doi.org/10.1080/23311932.2016.1254420.
Supplementary_Table_1.xlsx
Download MS Excel (34.7 KB)Acknowledgments
The author acknowledges a Postdoctoral Fellowship availed at the affiliated institution.
Additional information
Funding
Notes on contributors
Biswapriya Biswavas Misra
Biswapriya Biswavas Misra, PhD (supporting photograph) is a Postdoctoral Scientist at the Texas Biomedical Research Institute, San Antonio, Texas, USA. His current research interests are metabolomics and genomics towards a holistic understanding of organismal biology. He had obtained a PhD in Plant Biotechnology from Indian Institute of Technology Kharagpur, West Bengal, India followed by Postdoctoral trainings in Plant Genomics from Malaysia and in Metabolomics of Canola from University of Florida, Gainesville, USA. One of his ambitions is to help the metabolomics user community realize the potentials of harnessing plant metabolomics information, tools and methods towards a meaningful utilization of phytochemistry.
References
- Albertin, W., Langella, O., Joets, J., Négroni, L., Zivy, M., Damerval, C., & Thiellement, H. (2009). Comparative proteomics of leaf, stem, and root tissues of synthetic Brassica napus. Proteomics, 9, 793–799.10.1002/pmic.v9:3
- Auger, B., Marnet, N., Gautier, V., Maia-Grondard, A., Leprince, F., Renard, M., … Routaboul, J. M. (2010). A detailed survey of seed coat flavonoids in developing seeds of Brassica napus L. Journal of Agricultural and Food Chemistry, 58, 6246–6256.10.1021/jf903619v
- Bailly, C. (2004). Active oxygen species and antioxidants in seed biology. Seed Science Research, 14, 93–107.10.1079/SSR2004159
- Bartley, G. E., & Scolnik, P. A. (1995). Plant carotenoids: Pigments for photoprotection, visual attraction, and human health. The Plant Cell Online, 7, 1027–1038.10.1105/tpc.7.7.1027
- Basu, U., Francis, J. L., Whittal, R. M., Stephens, J. L., Wang, Y., Zaiane, O. R., … Taylor, G. J. (2006). Extracellular proteomes of Arabidopsis thaliana and Brassica napus roots: Analysis and comparison by MudPIT and LC-MS/MS. Plant and Soil, 286, 357–376.10.1007/s11104-006-9048-9
- Baumert, A., Milkowski, C., Schmidt, J., Nimtz, M., Wray, V., & Strack, D. (2005). Formation of a complex pattern of sinapate esters in Brassica napus seeds, catalyzed by enzymes of a serine carboxypeptidase-like acyltransferase family? Phytochemistry, 66, 1334–1345.10.1016/j.phytochem.2005.02.031
- Biermann, U., Bornscheuer, U., Meier, M. A., Metzger, J. O., & Schäfer, H. J. (2011). Oils and fats as renewable raw materials in chemistry. Angewandte Chemie International Edition, 50, 3854–3871.10.1002/anie.201002767
- Böttcher, C., von Roepenack-Lahaye, E., Schmidt, J., Clemens, S., & Scheel, D. (2009). Analysis of phenolic choline esters from seeds of Arabidopsis thaliana and Brassica napus by capillary liquid chromatography/electrospray-tandem mass spectrometry. Journal of Mass Spectrometry, 44, 466–476.10.1002/jms.1522
- Bottcher, C., von Roepenack-Lahaye, E., Schmidt, J., Schmotz, C., Neumann, S., Scheel, D., & Clemens, S. (2008). Metabolome analysis of biosynthetic mutants reveals a diversity of metabolic changes and allows identification of a large number of new compounds in Arabidopsis. Plant Physiology, 147, 2107–2120.10.1104/pp.108.117754
- Buhtz, A., Springer, F., Chappell, L., Baulcombe, D. C., & Kehr, J. (2008). Identification and characterization of small RNAs from the phloem of Brassica napus. The Plant Journal, 53, 739–749.10.1111/tpj.2008.53.issue-5
- Bus, A., Hecht, J., Huettel, B., Reinhardt, R., & Stich, B. (2012). High-throughput polymorphism detection and genotyping in Brassica napus using next-generation RAD sequencing. BMC Genomics, 13, 281.10.1186/1471-2164-13-281
- Cai, R., & Arntfield, S. D. (2001). A rapid high-performance liquid chromatographic method for the determination of sinapine and sinapic acid in canola seed and meal. Journal of the American Oil Chemists' Society, 78, 903–910.10.1007/s11746-001-0362-4
- Cao, T., Srivastava, S., Rahman, M. H., Kav, N. N., Hotte, N., Deyholos, M. K., & Strelkov, S. E. (2008). Proteome-level changes in the roots of Brassica napus as a result of Plasmodiophora brassicae infection. Plant Science, 174, 97–115.10.1016/j.plantsci.2007.10.002
- Carlsson, J., Lagercrantz, U., Sundström, J., Teixeira, R., Wellmer, F., Meyerowitz, E. M., & Glimelius, K. (2007). Microarray analysis reveals altered expression of a large number of nuclear genes in developing cytoplasmic male sterile Brassica napus flowers. The Plant Journal, 49, 452–462.10.1111/tpj.2007.49.issue-3
- Castro-Puyana, M., & Herrero, M. (2013). Metabolomics approaches based on mass spectrometry for food safety, quality and traceability. TrAC Trends in Analytical Chemistry, 52, 74–87.10.1016/j.trac.2013.05.016
- Chalhoub, B., Denoeud, F., Liu, S., Parkin, I. A., Tang, H., Wang, X., … Corréa, M. (2014). Early allopolyploid evolution in the post-Neolithic Brassica napus oilseed genome. Science, 345, 950–953.10.1126/science.1253435
- Clarke, J. D., Alexander, D. C., Ward, D. P., Ryals, J. A., Mitchell, M. W., Wulff, J. E., & Guo, L. (2013). Assessment of genetically modified soybean in relation to natural variation in the soybean seed metabolome. Scientific Reports, 3.
- Demartini, D. R., Jain, R., Agrawal, G., & Thelen, J. J. (2011). Proteomic comparison of plastids from developing embryos and leaves of Brassica napus. Journal of Proteome Research, 10, 2226–2237.
- De Smet, I., Lau, S., Mayer, U., & Jürgens, G. (2010). Embryogenesis-the humble beginnings of plant life. The Plant Journal, 61, 959–970.10.1111/tpj.2010.61.issue-6
- De Vos, R. C., Moco, S., Lommen, A., Keurentjes, J. J., Bino, R. J., & Hall, R. D. (2007). Untargeted large-scale plant metabolomics using liquid chromatography coupled to mass spectrometry. Nature Protocols, 2, 778–791.10.1038/nprot.2007.95
- Delourme, R., Falentin, C., Fomeju, B. F., Boillot, M., Lassalle, G., André, I., … Pauchon, M. (2013). High-density SNP-based genetic map development and linkage disequilibrium assessment in Brassica napus L. BMC Genomics, 14, 120.10.1186/1471-2164-14-120
- Dixon, R. A., & Paiva, N. L. (1995). Stress-induced phenylpropanoid metabolism. The Plant Cell Online, 7, 1085.10.1105/tpc.7.7.1085
- Dong, J., Yan, W., Bock, C., Nokhrina, K., Keller, W., & Georges, F. (2013). Perturbing the metabolic dynamics of myo-inositol in developing Brassica napus seeds through in vivo methylation impacts its utilization as phytate precursor and affects downstream metabolic pathways. BMC Plant Biology, 13, 84.10.1186/1471-2229-13-84
- El-Beltagi, H. E.-D., & Mohamed, A. A. (2010). Variations in fatty acid composition, glucosinolate profile and some phytochemical contents in selected oil seed rape (Brassica napus L.) cultivars. Grasas Aceites, 61, 143–150.
- Endo, Y., Thorsteinson, C. T., & Daun, J. K. (1992). Characterization of chlorophyll pigments present in canola seed, meal and oil. Journal of the American Oil Chemists' Society, 69, 564–568.10.1007/BF02636109
- Fahey, J. W., Zalcmann, A. T., & Talalay, P. (2001). The chemical diversity and distribution of glucosinolates and isothiocyanates among plants. Phytochemistry, 56, 5–51.10.1016/S0031-9422(00)00316-2
- Farag, M. A., Gad, H. A., Heiss, A. G., & Wessjohann, L. A. (2014). Metabolomics driven analysis of six Nigella species seeds via UPLC-qTOF-MS and GC-MS coupled to chemometrics. Food Chemistry, 151, 333–342.10.1016/j.foodchem.2013.11.032
- Farag, M. A., Sharaf Eldin, M. G., Kassem, H., & Abou el Fetouh, M. (2013). Metabolome classification of Brassica napus L. organs via UPLC-QTOF-PDA-MS and their anti-oxidant potential. Phytochemical Analysis, 24, 277–287.10.1002/pca.v24.3
- Finkelstein, R., Reeves, W., Ariizumi, T., & Steber, C. (2008). Molecular aspects of seed dormancy. Annual Review of Plant Biology, 59, 387–415.10.1146/annurev.arplant.59.032607.092740
- Floerl, S., Druebert, C., Majcherczyk, A., Karlovsky, P., Kues, U., & Polle, A. (2008). Defence reactions in the apoplastic proteome of oilseed rape (Brassica napus var. napus) attenuate Verticillium longisporum growth but not disease symptoms. BMC Plant Biology, 8, 129.10.1186/1471-2229-8-129
- Frolov, A., Henning, A., Böttcher, C., Tissier, A., & Strack, D. (2013). An UPLC-MS/MS method for the simultaneous identification and quantitation of cell wall phenolics in Brassica napus seeds. Journal of Agricultural and Food Chemistry, 61, 1219–1227.10.1021/jf3042648
- Gaeta, R. T., Pires, J. C., Iniguez-Luy, F., Leon, E., & Osborn, T. C. (2007). Genomic changes in resynthesized Brassica napus and their effect on gene expression and phenotype. The Plant Cell Online, 19, 3403–3417.10.1105/tpc.107.054346
- Gardiner, J. B., Morra, M. J., Eberlein, C. V., Brown, P. D., & Borek, V. (1999). Allelochemicals released in soil following incoporation of rapeseed (Brassica napus) green manures. Journal of Agricultural and Food Chemistry, 47, 3387–3842.
- Geng, S., Misra, B. B., Armas, E., Huhman, D. V., Alborn, H. T., Sumner, L. W., & Chen, S. (2016). Jasmonate-mediated stomatal closure under elevated CO2 revealed by time-resolved metabolomics. The Plant Journal. Retrieved from http://onlinelibrary.wiley.com/doi/10.1111/tpj.13296/abstract;jsessionid=9663D586575E1E51189F3EE4C4CF1003.f02t02. doi:10.1111/tpj.13296
- Georges, F., Das, S., Ray, H., Bock, C., Nokhrina, K., Kolla, V. A., & Keller, W. (2009). Over-expression of Brassica napus phosphatidylinositol-phospholipase C2 in canola induces significant changes in gene expression and phytohormone distribution patterns, enhances drought tolerance and promotes early flowering and maturation. Plant, Cell & Environment, 32, 1664–1681.10.1111/pce.2009.32.issue-12
- Giavalisco, P., Kapitza, K., Kolasa, A., Buhtz, A., & Kehr, J. (2006). Towards the proteome of Brassica napus phloem sap. Proteomics, 6, 896–909.10.1002/(ISSN)1615-9861
- Gunstone, F. D., Harwood, J. L., & Dijkstra, A. J. (2007). The lipid handbook with CD-ROM. Boca Raton, FL: CRC Press.
- Hahlbrock, K., & Scheel, D. (1989). Physiology and molecular biology of phenylpropanoid metabolism. Annual Review of Plant Physiology and Plant Molecular Biology, 40, 347–369.10.1146/annurev.pp.40.060189.002023
- Haimi, P., Uphoff, A., Hermansson, M., & Somerharju, P. (2006). Software tools for analysis of mass spectrometric lipidome data. Analytical Chemistry, 78, 8324–8331.10.1021/ac061390w
- Hajduch, M., Casteel, J. E., Hurrelmeyer, K. E., Song, Z., Agrawal, G. K., & Thelen, J. J. (2006). Proteomic analysis of seed filling in Brassica napus. Developmental characterization of metabolic isozymes using high-resolution two-dimensional gel electrophoresis. Plant Physiology, 141, 32–46.10.1104/pp.105.075390
- Halkier, B. A., & Du, L. (1997). The biosynthesis of glucosinolates. Trends in Plant Science, 2, 425–431.10.1016/S1360-1385(97)90026-1
- Hall, R., Beale, M., Fiehn, O., Hardy, N., Sumner, L., & Bino, R. (2002). Plant metabolomics: The missing link in functional genomics strategies. THE PLANT CELL ONLINE, 14, 1437–1440.10.1105/tpc.140720
- Hamama, A. A., Bhardwaj, H. L., & Starner, D. E. (2003). Genotype and growing location effects on phytosterols in canola oil. Journal of the American Oil Chemists' Society, 80, 1121–1126.10.1007/s11746-003-0829-3
- Han, X., & Gross, R. W. (2005). Shotgun lipidomics: Electrospray ionization mass spectrometric analysis and quantitation of cellular lipidomes directly from crude extracts of biological samples. Mass Spectrometry Reviews, 24, 367–412.10.1002/(ISSN)1098-2787
- Han, J., Lu, C., Li, Y., Deng, Z., Fu, B., & Geng, Z. (2015). Discrimination of rapeseeds (Brassica napus L.) based on the content of erucic acid by 1H NMR. European Food Research and Technology, 242, 1–7.
- Handa, H. (2003). The complete nucleotide sequence and RNA editing content of the mitochondrial genome of rapeseed (Brassica napus L.): Comparative analysis of the mitochondrial genomes of rapeseed and Arabidopsis thaliana. Nucleic Acids Research, 31, 5907–5916.10.1093/nar/gkg795
- Harper, A. L., Trick, M., Higgins, J., Fraser, F., Clissold, L., Wells, R., … Bancroft, I. (2012). Associative transcriptomics of traits in the polyploid crop species Brassica napus. Nature Biotechnology, 30, 798–802.10.1038/nbt.2302
- Higgins, J., Magusin, A., Trick, M., Fraser, F., & Bancroft, I. (2012). Use of mRNA-seq to discriminate contributions to the transcriptome from the constituent genomes of the polyploid crop species Brassica napus. BMC Genomics, 13, 247.10.1186/1471-2164-13-247
- Holdsworth, M. J., Bentsink, L., & Soppe, W. J. (2008). Molecular networks regulating Arabidopsis seed maturation, after-ripening, dormancy and germination. New Phytologist, 179, 33–54.10.1111/nph.2008.179.issue-1
- Horn, P. J., & Chapman, K. D. (2012). Lipidomics in tissues, cells and subcellular compartments. The Plant Journal, 70, 69–80.10.1111/j.1365-313X.2011.04868.x
- Horn, P. J., & Chapman, K. D. (2014). Lipidomics in situ: Insights into plant lipid metabolism from high resolution spatial maps of metabolites. Progress in Lipid Research , 54, 32–52.10.1016/j.plipres.2014.01.003
- Hummel, J., Segu, S., Li, Y., Irgang, S., Jueppner, J., & Giavalisco, P. (2011). Ultra performance liquid chromatography and high resolution mass spectrometry for the analysis of plant lipids. Frontiers in Plant Science, 2, 54.
- Jadhav, A. S., Taylor, D. C., Giblin, M., Ferrie, A. M., Ambrose, S. J., Ross, A. R., … Fobert, P. R. (2008). Hormonal regulation of oil accumulation in Brassica seeds: Metabolism and biological activity of ABA, 7′-, 8′- and 9′-hydroxy ABA in microspore derived embryos of B. napus. Phytochemistry, 69, 2678–2688.10.1016/j.phytochem.2008.08.010
- Jahangir, M., Abdel-Farid, I. B., Simoh, S., Kim, H. K., Verpoorte, R., & Choi, Y. H. (2012). Investigation of Brassica biochemical status by NMR-based metabolomics. Acta Horticulturae, 936, 163–172.
- Jain, R., Katavic, V., Agrawal, G. K., Guzov, V. M., & Thelen, J. J. (2008). Purification and proteomic characterization of plastids from Brassica napus developing embryos. Proteomics, 8, 3397–3405.10.1002/pmic.v8:16
- Jensen, C. R., Mogensen, V. O., Mortensen, G., Fieldsend, J. K., Milford, G. F. J., Andersen, M. N., & Thage, J. H. (1996). Seed glucosinolate, oil and protein contents of field-grown rape (Brassica napus L.) affected by soil drying and evaporative demand. Field Crops Research, 47, 93–105.10.1016/0378-4290(96)00026-3
- Jiang, Y., & Deyholos, M. K. (2010). Transcriptome analysis of secondary-wall-enriched seed coat tissues of canola (Brassica napus L.). Plant Cell Reports, 29, 327–342.10.1007/s00299-010-0824-x
- Jiang, C., Shi, J., Li, R., Long, Y., Wang, H., Li, D., … Meng, J. (2014). Quantitative trait loci that control the oil content variation of rapeseed (Brassica napus L.). Theoretical and Applied Genetics, 127, 957–968.10.1007/s00122-014-2271-5
- Jiang, J., Shao, Y., Li, A., Zhang, Y., Wei, C., & Wang, Y. (2013). FT-IR and NMR study of seed coat dissected from different colored progenies of Brassica napus-Sinapis alba hybrids. Journal of Agricultural and Food Chemistry, 93, 898–1902.
- Jiang, J., Wang, Y., Xie, T., Rong, H., Li, A., Fang, Y., & Wang, Y. (2015). Metabolic characteristics in meal of black rapeseed and yellow-seeded progeny of Brassica napus–Sinapis alba hybrids. Molecules, 20, 21204–21213.10.3390/molecules201219761
- Joët, T., Wurtzel, E. T., Matsuda, F., Saito, K., Dussert, S. (2012) Coupled transcript-metabolite profiling: Towards systems biology approaches to unravel regulation of seed secondary metabolism. In Agrawal, G. K., & Rakwal, R. B. (Eds.), Seed development: OMICS technologies toward improvement of seed quality and crop yield (pp. 367–385). Dordrecht: Springer.
- Katavic, V., Agrawal, G. K., Hajduch, M., Harris, S. L., & Thelen, J. J. (2006). Protein and lipid composition analysis of oil bodies from two Brassica napus cultivars. Proteomics, 6, 4586–4598.10.1002/(ISSN)1615-9861
- Kehr, J., Buhtz, A., & Giavalisco, P. (2005). Analysis of xylem sap proteins from Brassica napus. BMC Plant Biology, 5, 11.10.1186/1471-2229-5-11
- Kim, H. K., Choi, Y. H., & Verpoorte, R. (2011). NMR-based plant metabolomics: Where do we stand, where do we go? Trends in Biotechnology, 29, 267–275.10.1016/j.tibtech.2011.02.001
- King, S. P., Lunn, J. E., & Furbank, R. T. (1997). Carbohydrate content and enzyme metabolism in developing canola siliques. Plant Physiology, 114, 153–160.10.1104/pp.114.1.153
- Koh, J., Chen, G., Yoo, M. J., Zhu, N., Dufresne, D., Erickson, J. E., … Chen, S. (2015). Comparative proteomic analysis of Brassica napus in response to drought stress. Journal of Proteome Research, 14, 3068–3081.10.1021/pr501323d
- Koornneef, M., Bentsink, L., & Hilhorst, H. (2002). Seed dormancy and germination. Current Opinion in Plant Biology, 5, 33–36.10.1016/S1369-5266(01)00219-9
- Körber, N., Bus, A., Li, J., Higgins, J., Bancroft, I., Higgins, E. E., … Stich, B. (2015). Seedling development traits in Brassica napus examined by gene expression analysis and association mapping. BMC Plant Biology, 15, 136.10.1186/s12870-015-0496-3
- Kortesniemi, M., Vuorinen, A. L., Sinkkonen, J., Yang, B., Rajala, A., & Kallio, H. (2015). NMR metabolomics of ripened and developing oilseed rape (Brassica napus) and turnip rape (Brassica rapa). Food Chemistry, 172, 63–70.10.1016/j.foodchem.2014.09.040
- Kubala, S., Garnczarska, M., Wojtyla, Ł., Clippe, A., Kosmala, A., Żmieńko, A., … Quinet, M. (2015). Deciphering priming-induced improvement of rapeseed (Brassica napus L.) germination through an integrated transcriptomic and proteomic approach. Plant Science, 231, 94–113.10.1016/j.plantsci.2014.11.008
- Kubala, S., Lechowska, K., Wojtyla, L., Kosmala, A., Quinet, M., Lutts, S., & Garnczarska, M. (2013). Transcriptome and proteome changes accompanying increased vigor of osmoprimed rape (Brassica napus L.) seeds. BioTechnologia. Journal of Biotechnology Computational Biology and Bionanotechnology, 94, 3 pp.
- Li, G., Gao, M., Yang, B., & Quiros, C. F. (2003). Gene for gene alignment between the Brassica and Arabidopsis genomes by direct transcriptome mapping. Theoretical and Applied Genetics, 107, 168–180.
- Li, F., Wu, X., Tsang, E., & Cutler, A. J. (2005). Transcriptional profiling of imbibed Brassica napus seed. Genomics, 86, 718–730.10.1016/j.ygeno.2005.07.006
- Liang, Y., Strelkov, S. E., & Kav, N. N. (2009). Oxalic acid-mediated stress responses in Brassica napus L. Proteomics, 9, 3156–3173.10.1002/pmic.v9:11
- Lorenz, C., Rolletschek, H., Sunderhaus, S., & Braun, H. P. (2014). Brassica napus seed endosperm—Metabolism and signaling in a dead end tissue. Journal of Proteomics, 108, 382–426.10.1016/j.jprot.2014.05.024
- Marmagne, A., Brabant, P., Thiellement, H., & Alix, K. (2010). Analysis of gene expression in resynthesized Brassica napus allotetraploids: Transcriptional changes do not explain differential protein regulation. New Phytologist, 186, 216–227.10.1111/j.1469-8137.2009.03139.x
- Miernyk, J. A., & Johnston, M. L. (2012). Digging deeper into the seed proteome: Prefractionation of total proteins. In Agrawal, G. K., & Rakwal, R. (Eds.), Seed development: OMICS technologies toward improvement of seed quality and crop yield (pp. 265–278). Dordrecht: Springer.
- Misra, B. B., & van der Hooft, J. J. (2016). Updates in metabolomics tools and resources: 2014-2015. Electrophoresis, 37, 86–110.10.1002/elps.v37.1
- Misra, B. B., Acharya, B. R., Granot, D., Assmann, S. M., & Chen, S. (2015). The guard cell metabolome: Functions in stomatal movement and global food security. Front Plant Science, 6, 334.
- Misra, B. B., Assmann, S. M., & Chen, S. (2014). Plant single-cell and single-cell-type metabolomics. Trends in Plant Science, 19, 637–646.10.1016/j.tplants.2014.05.005
- Misra, B. B., de Armas, E., Tong, Z., & Chen, S. (2015). Metabolomic responses of guard cells and mesophyll cells to bicarbonate. PloS One, 10, e0144206.
- Moles, A. T., Ackerly, D. D., Webb, C. O., Tweddle, J. C., Dickie, J. B., & Westoby, M. (2005). A brief history of seed size. Science, 307, 576–580.10.1126/science.1104863
- Mounet, F., Lemaire-Chamley, M., Maucourt, M., Cabasson, C., Giraudel, J. L., Deborde, C., … Rothan, C. (2007). Quantitative metabolic profiles of tomato flesh and seeds during fruit development: Complementary analysis with ANN and PCA. Metabolomics, 3, 273–288.10.1007/s11306-007-0059-1
- Nambara, E., & Nonogaki, H. (2012). Seed biology in the 21st century: Perspectives and new directions. Plant and Cell Physiology, 53, 1–4.10.1093/pcp/pcr184
- North, H., Baud, S., Debeaujon, I., Dubos, C., Dubreucq, B., Grappin, P., … Rajjou, L. (2010). Arabidopsis seed secrets unravelled after a decade of genetic and omics-driven research. The Plant Journal, 61, 971–981.10.1111/tpj.2010.61.issue-6
- Norton, G., & Harris, J. F. (1975). Compositional changes in developing rape seed (Brassica napus L.). Planta, 123, 163–174.10.1007/BF00383865
- Obied, H. K., Song, Y., Foley, S., Loughlin, M., Rehman, A., Mailer, R., … Agboola, S. (2013). Biophenols and antioxidant properties of Australian canola meal. Journal of Agricultural and Food Chemistry, 61, 9176–9184.10.1021/jf4026585
- Okazaki, Y., Kamide, Y., Hirai, M. Y., & Saito, K. (2013). Plant lipidomics based on hydrophilic interaction chromatography coupled to ion trap time-of-flight mass spectrometry. Metabolomics, 9, 121–131.10.1007/s11306-011-0318-z
- Oraby, H. F., & Ramadan, M. F. (2014). Impact of suppressing the caffeic acid O-methyltransferase (COMT) gene on lignin, fiber, and seed oil composition in Brassica napus transgenic plants. European Food Research and Technology, 240, 931–938.
- Pan, M., Jiang, T. S., & Pan, J. L. (2011). Antioxidant activities of rapeseed protein hydrolysates. Food and Bioprocess Technology, 4, 1144–1152.10.1007/s11947-009-0206-y
- Parkin, I. A., Gulden, S. M., Sharpe, A. G., Lukens, L., Trick, M., Osborn, T. C., & Lydiate, D. J. (2005). Segmental structure of the Brassica napus genome based on comparative analysis with Arabidopsis thaliana. Genetics, 171, 765–781.10.1534/genetics.105.042093
- Parkin, I. A. P., Sharpe, A. G., Keith, D. J., & Lydiate, D. J. (1995). Identification of the A and C genomes of amphidiploid Brassica napus (oilseed rape). Genome, 38, 1122–1131.10.1139/g95-149
- Penrose, D. M., & Glick, B. R. (2001). Levels of ACC and related compounds in exudate and extracts of canola seeds treated with ACC deaminase-containing plant growth-promoting bacteria. Canadian Journal of Microbiology, 47, 368–372.10.1139/w01-014
- Provart, N. J., Alonso, J., Assmann, S. M., Bergmann, D., Brady, S. M., Brkljacic, J., … Dangl, J. (2015). 50 years of Arabidopsis research: Highlights and future directions. New Phytologist, 209, 921–944.
- Qian, L., Qian, W., & Snowdon, R. J. (2014). Sub-genomic selection patterns as a signature of breeding in the allopolyploid Brassica napus genome. BMC Genomics, 15, 1170.10.1186/1471-2164-15-1170
- Raman, H., Dalton-Morgan, J., Diffey, S., Raman, R., Alamery, S., Edwards, D., & Batley, J. (2014). SNP markers-based map construction and genome-wide linkage analysis in Brassica napus. Plant Biotechnology Journal, 12, 851–860.10.1111/pbi.2014.12.issue-7
- Ravanello, M. P., Ke, D., Alvarez, J., Huang, B., & Shewmaker, C. K. (2003). Coordinate expression of multiple bacterial carotenoid genes in canola leading to altered carotenoid production. Metabolic Engineering, 5, 255–263.10.1016/j.ymben.2003.08.001
- Richter, E. K., Spangenberg, J. E., Kreuzer, M., & Leiber, F. (2010). Characterization of rapeseed (Brassica napus) oils by bulk C, O, H, and fatty acid C stable isotope analyses. Journal of Agricultural and Food Chemistry, 58, 8048–8055.10.1021/jf101128f
- Sawada, Y., Akiyama, K., Sakata, A., Kuwahara, A., Otsuki, H., Sakurai, T., … Hirai, M. Y. (2009). Widely targeted metabolomics based on large-scale MS/MS data for elucidating metabolite accumulation patterns in plants. Plant and Cell Physiology, 50, 37–47.10.1093/pcp/pcn183
- Schmidt, M. A., Barbazuk, W. B., Sandford, M., May, G., Song, Z., Zhou, W., … Herman, E. M. (2011). Silencing of soybean seed storage proteins results in a rebalanced protein composition preserving seed protein content without major collateral changes in the metabolome and transcriptome. Plant Physiology, 156, 330–345.
- Schwender, J., Hebbelmann, I., Heinzel, N., Tatjana, M. H., Rogers, A., Naik, D., … Borisjuk, L. (2015). Quantitative multilevel analysis of central metabolism in developing oilseeds of Brassica napus during in vitro culture. Plant Physiology, 168, 828–848.
- Schwender, J., Ohlrogge, J. B., & Shachar-Hill, Y. (2003). A flux model of glycolysis and the oxidative pentosephosphate pathway in developing Brassica napus embryos. Journal of Biological Chemistry, 278, 29442–29453.10.1074/jbc.M303432200
- Shao, Y., Jiang, J., Ran, L., Lu, C., Wei, C., & Wang, Y. (2014). Analysis of flavonoids and hydroxycinnamic acid derivatives in rapeseeds (Brassica napus L. var. napus ) by HPLC-PDA–ESI(−)-MS n /HRMS. Journal of Agricultural and Food Chemistry, 62, 2935–2945.10.1021/jf404826u
- Sheoran, I. S., & Sawhney, V. K. (2010). Proteome analysis of the normal and Ogura (ogu) CMS anthers of Brassica napus to identify proteins associated with male sterility. Botany, 88, 217–230.10.1139/B09-085
- Shewmaker, C. K., Sheehy, J. A., Daley, M., Colburn, S., & Ke, D. Y. (1999). Seed-specific overexpression of phytoene synthase: Increase in carotenoids and other metabolic effects. The Plant Journal, 20, 401–412.10.1046/j.1365-313x.1999.00611.x
- Shu, X. L., Frank, T., Shu, Q. Y., & Engel, K. H. (2008). Metabolite profiling of germinating rice seeds. Journal of Agricultural and Food Chemistry, 56, 11612–11620.10.1021/jf802671p
- Simbaya, J., Slominski, B. A., Rakow, G., Campbell, L. D., Downey, R. K., & Bell, J. M. (1995). Quality characteristics of yellow-seeded Brassica seed meals: Protein, carbohydrate, and dietary fiber components. Journal of Agricultural and Food Chemistry, 43, 2062–2066.10.1021/jf00056a020
- Slominski, B. A., Campbell, L. D., & Guenter, W. (1994). Carbohydrates and dietary fiber components of yellow- and brown-seeded canola. Journal of Agricultural and Food Chemistry, 42, 704–707.10.1021/jf00039a020
- Sumner, L. W., Mendes, P., & Dixon, R. A. (2003). Plant metabolomics: Large-scale phytochemistry in the functional genomics era. Phytochemistry, 62, 817–836.10.1016/S0031-9422(02)00708-2
- Szklarczyk, D., Santos, A., von Mering, C., Jensen, L. J., Bork, P., & Kuhn, M. (2016). STITCH 5: Augmenting protein-chemical interaction networks with tissue and affinity data. Nucleic Acids Research, 44, D380–4.
- Tan, H., Xie, Q., Xiang, X., Li, J., Zheng, S., Xu, X., Guo, H., & Ye, W. (2015). Dynamic metabolic profiles and tissue-specific source effects on the metabolome of developing seeds of Brassica napus. PLOS ONE, e0124794.10.1371/journal.pone.0124794
- Teh, S. S., & Birch, J. (2013). Physicochemical and quality characteristics of cold-pressed hemp, flax and canola seed oils. Journal of Food Composition and Analysis, 30, 26–31.10.1016/j.jfca.2013.01.004
- Thiyam, U., Claudia, P., Jan, U., & Alfred, B. (2009). De-oiled rapeseed and a protein isolate: Characterization of sinapic acid derivatives by HPLC-DAD and LC-MS. European Food Research and Technology, 229, 825–831.10.1007/s00217-009-1122-0
- Trick, M., Long, Y., Meng, J., & Bancroft, I. (2009). Single nucleotide polymorphism (SNP) discovery in the polyploid Brassica napus using Solexa transcriptome sequencing. Plant Biotechnology Journal, 7, 334–346.10.1111/pbi.2009.7.issue-4
- Tuberoso, C. I. G., Kowalczyk, A., Sarritzu, E., & Cabras, P. (2007). Determination of antioxidant compounds and antioxidant activity in commercial oilseeds for food use. Food Chemistry, 103, 1494–1501.10.1016/j.foodchem.2006.08.014
- Velasco, P., Francisco, M., Moreno, D. A., Ferreres, F., García-Viguera, C., & Cartea, M. E. (2011). Phytochemical fingerprinting of vegetable Brassica oleracea and Brassica napus by simultaneous identification of glucosinolates and phenolics. Phytochemical Analysis, 22, 144–152.10.1002/pca.v22.2
- Velasco, P., Soengas, P., Vilar, M., Cartea, M. E., & del Rio, M. (2008). Comparison of glucosinolate profiles in leaf and seed tissues of different Brassica napus crops. Journal of the American Society for Horticultural Science, 133, 551–558.
- Vigeolas, H., Chinoy, C., Zuther, E., Blessington, B., Geigenberger, P., & Domoney, C. (2008). Combined metabolomic and genetic approaches reveal a link between the polyamine pathway and albumin 2 in developing pea seeds. Plant Physiology, 146, 74–82.
- Vuorinen, A. L., Kalpio, M., Linderborg, K. M., Kortesniemi, M., Lehto, K., Niemi, J., … Kallio, H. P. (2014). Coordinate changes in gene expression and triacylglycerol composition in the developing seeds of oilseed rape (Brassica napus) and turnip rape (Brassica rapa). Food Chemistry, 145, 664–673.10.1016/j.foodchem.2013.08.108
- Walton, L. J., Kurepin, L. V., Yeung, E. C., Shah, S., Emery, R. N., Reid, D. M., & Pharis, R. P. (2012). Ethylene involvement in silique and seed development of canola, Brassica napus L. Plant Physiology and Biochemistry, 58, 142–150.10.1016/j.plaphy.2012.06.016
- Wang, J., Lydiate, D. J., Parkin, I. A., Falentin, C., Delourme, R., Carion, P. W., & King, G. J. (2011). Integration of linkage maps for the Amphidiploid Brassica napus and comparative mapping with Arabidopsis and Brassica rapa. BMC Genomics, 12, 101.10.1186/1471-2164-12-101
- Wang, Z., Wang, Z., Shi, L., Wang, L., & Xu, F. (2010). Proteomic alterations of Brassica napus root in response to boron deficiency. Plant Molecular Biology, 74, 265–278.10.1007/s11103-010-9671-y
- Wang, X., Wang, H., Wang, J., Sun, R., Wu, J., Liu, S., … Huang, S. (2011). The genome of the mesopolyploid crop species Brassica rapa. Nature Genetics, 43, 1035–1039.10.1038/ng.919
- Xiong, Z., Gaeta, R. T., & Pires, J. C. (2011). Homoeologous shuffling and chromosome compensation maintain genome balance in resynthesized allopolyploid Brassica napus. Proceedings of the National Academy of Sciences, 108, 7908–7913.10.1073/pnas.1014138108
- Xu, Y., Zhong, L., Wu, X., Fang, X., & Wang, J. (2009). Rapid alterations of gene expression and cytosine methylation in newly synthesized Brassica napus allopolyploids. Planta, 229, 471–483.10.1007/s00425-008-0844-8
- Yang, B., Srivastava, S., Deyholos, M. K., & Kav, N. N. (2007). Transcriptional profiling of canola (Brassica napus L.) responses to the fungal pathogen Sclerotinia sclerotiorum. Plant Science, 173, 156–171.10.1016/j.plantsci.2007.04.012
- Yao, Y., Sun, H., Xu, F., Zhang, X., & Liu, S. (2011). Comparative proteome analysis of metabolic changes by low phosphorus stress in two Brassica napus genotypes. Planta, 233, 523–537.10.1007/s00425-010-1311-x
- Yin, X., He, D., Gupta, R., & Yang, P. (2015). Physiological and proteomic analyses on artificially aged Brassica napus seed. Frontiers in Plant Science, 6, 112.
- Yokozawa, T., Kim, H. Y., Cho, E. J., Choi, J. S., & Chung, H. Y. (2002). Antioxidant effects of isorhamnetin 3, 7-di-O-β-D-glucopyranoside isolated from mustard leaf (Brassica juncea) in rats with streptozotocin-induced diabetes. Journal of Agricultural and Food Chemistry, 50, 5490–5495.
- Yu, O., & Jez, J. M. (2008). Nature’s assembly line: Biosynthesis of simple phenylpropanoids and polyketides. The Plant Journal, 54, 750–762.
- Zhang, K., Lu, K., Qu, C., Liang, Y., Wang, R., Chai, Y., & Li, J. (2013). Gene silencing of BnTT10 family genes causes retarded pigmentation and lignin reduction in the seed coat of Brassica napus. PLoS ONE, e61247.10.1371/journal.pone.0061247
- Zhao, F., Evans, E. J., Bilsborrow, P. E., & Syers, J. K. (1994). Influence of nitrogen and sulphur on the glucosinolate profile of rapeseed (Brassica napus L). Journal of the Science of Food and Agriculture, 64, 295–304.10.1002/(ISSN)1097-0010
- Zhao, Y. T., Wang, M., Fu, S. X., Yang, W. C., Qi, C. K., & Wang, X. J. (2012). Small RNA profiling in two Brassica napus cultivars identifies micrornas with oil production- and development-correlated expression and new small RNA classes. Plant Physiology, 158, 813–823.10.1104/pp.111.187666
- Zhou, Z. S., Song, J. B., & Yang, Z. M. (2012). Genome-wide identification of Brassica napus microRNAs and their targets in response to cadmium. Journal of Experimental Botany, 63, 4597–4613.10.1093/jxb/ers136
- Zhu, M., Dai, S., McClung, S., Yan, X., & Chen, S. (2009). Functional differentiation of Brassica napus guard cells and mesophyll cells revealed by comparative proteomics. Molecular & Cellular Proteomics, 8, 752–766.10.1074/mcp.M800343-MCP200
- Zhu, M., Dai, S., Zhu, N., Booy, A., Simons, B., Yi, S., & Chen, S. (2012). Methyl jasmonate responsive proteins in Brassica napus guard cells revealed by iTRAQ-based quantitative proteomics. Journal of Proteome Research, 11, 3728–3742.10.1021/pr300213k
- Zhu, M., Simons, B., Zhu, N., Oppenheimer, D. G., & Chen, S. (2010). Analysis of abscisic acid responsive proteins in Brassica napus guard cells by multiplexed isobaric tagging. Journal of Proteomics, 73, 790–805.10.1016/j.jprot.2009.11.002
- Zum Felde, T., Baumert, A., Strack, D., Becker, H. C., & Möllers, C. (2007). Genetic variation for sinapate ester content in winter rapeseed (Brassica napus L.) and development of NIRS calibration equations. Plant Breeding, 126, 291–296.10.1111/pbr.2007.126.issue-3