Abstract
In this study, purified thermo- and acid-stable cellulase from the Bacillus licheniformis was used for improving physical and sensory properties of wheat bread. The thermostable cellulase was harvested, purified and characterized from isolated B. licheniformis species. The impact of parameters such as temperature, pH, and metal ions was tested on the purified cellulase enzyme. The purified enzyme was found to be stable at pH 4.0 and also thermally stable for 6 hat 45°C. Purified cellulase was used in preparation of wheat dough followed by the preparation of baked final product wheat bread. The final baked product showed a significant decrease in hardness, adhesiveness and also chewiness. The moisture-holding capacity was significantly increased compared to control. Furthermore, the effect of cellulase in combination with amylase was also studied in preparation of bread. The improvement in sensory parameters such as volume, color, texture, aroma and taste was observed on treating the dough with a combination of cellulase and amylase.
1. Introduction
Cellulose is the most widespread renewable biopolymer on the Earth, generally consisting of the structural part of plant cell walls (Caf et al., Citation2014). The highest amount of cellulose is present in plant stalks, stems and woody pieces of plants (Singhania et al., Citation2010). Cellulose also represents the major part of the fungal and algae cell wall with little presence in the bacterial cell wall. Structurally, cellulose is composed of glucose units joined with β-(1–4) glycosidic bonds, which further get hydrolyzed into soluble sugars by cellulase enzymes (Back & Kwon, Citation2007). Cellulase enzymes are also known for their flexibility and dynamism in terms of its function, structure and independent domains (Harjunpää et al., Citation1999).
Cellulases are a family of enzymes containing three primary components: (1) endoglucanase or endo-β-1, 4-D glucanase (EC 3.2.1.1), (2) exoglucanase or exo-β-1, 4-D glucanase (EC 3.2.1.91), and (3) β-glucosidase (EC 3.2.1.21). These three components catalyze the hydrolysis of cellulose molecules into glucose units by their synergistic effect (Horn et al., Citation2012). Endoglucanases acts on β-1, 4-glycosidic bonds of cellulose molecule chains to form short chains. Each short chain further hydrolyzed into new chain ends and liberates oligosaccharide polymers and glucose by exoglucanase and β-glucosidase enzymes. Endoglucanase and exoglucanase further produce cellobiose and cello-oligosaccharides from cellulose. Finally, β-glucosidases are used to convert cello-oligosaccharides and cellobiose into glucose monomers (Henrissat et al., Citation1998; Lakhundi et al., Citation2015).
In the environment, a wide diversity of microorganisms, including bacteria, fungi, and actinomycetes, produces cellulase enzymes that cover many ecological niches (Karim, Citation2020). Furthermore, cellulase enzyme works as a potential degrader of cellulose molecule present in industrial and agricultural wastes. Cellulase enzymes are produced by many microbes including bacteria and fungi, both of which can be thermophilic or acidophilic (Maijala, Citation2000). Apart from many reports suggesting fungi as a potential enzyme producer, bacteria is also reported as a potential cellulase producer (Nagendran et al., Citation2009). In many laboratories, cellulases are produced by several fungi because of their best enzyme activity, but a number of reports also suggested that bacteria have great potential to produce cellulase enzymes (Nagendran et al., Citation2009).
Currently, bacteria are used extensively for industrial productions of enzymes. Distinct advantages like higher growth rates, multi-enzyme complexes, and potential to thrive in different environments makes them ideal model for industrial enzyme production (Maki et al., Citation2009). Furthermore, bacteria can easily grow in low-cost nutrient sources for production of high cell biomass and secreting enzymes stable under extreme conditions of high or low pH and temperature. Different bacterial species have been reported for cellulase enzyme production such as Bacillus sp. (Soeka, Citation2019), Pseudomonas sp. (Goel et al., Citation2019), Cellulomonas sp., Ruminococcus sp., Micrococcus sp., and Clostridium sp. (Okeke & Lu, Citation2011). Among these, Bacillus species have great potential to produce a diversity of extracellular cellulolytic enzymes isolated from different environments, like soil, composts (Eida et al., Citation2009; Kim et al., Citation2012), forest soil (Kanchanadumkerng et al., Citation2017), mangroves soil, hot springs, agricultural environment, deep-sea sediment, insect or fish gut, and feces. Within fungi, Trichoderma and Aspergillus species are known for cellulase production (Adhyaru et al., Citation2015).
Among this microbial diversity, acidophiles are microorganisms that survives in highly acidic conditions (at pH 3.0–4.0). Mostly, cellulase enzymes from fungi show acidic optima. However, only a few species of bacteria are reported for the production of acidophilic cellulase enzymes. For example, Bacillus or Paenibacillus species have been of specific interest for the production of cellulase enzymes (Azadian et al., Citation2017; Rawat & Tewari, Citation2012). Cellulase enzymes from acidophilic bacteria have attracted great interest because of their potential for a variety of industrial and biotechnological applications in food and feed industry, backing industry, pulp and paper industry, textile and detergent industry, laundry industry, extraction of vegetable dyes, and agriculture(Bhat & Bhat, Citation1997; Zhang & Zhang, Citation2013). The demand for acid-stable cellulase enzymes is increasing rapidly. Many industrial processes needed acidic or extreme pH and great temperature, so if acid-stable cellulase is obtainable, the industry can decrease the cost of pH adjustment and the making process more reasonable.
In the baking industry, hydrolytic enzymes have gained great attention due to their potential effect on improving dough and bread quality. In the last few years, cellulase enzymes have gained great popularity in baking industry (Harada et al., Citation2005). The published report suggests that cellulase enzyme enhances dough rheological characteristics such as stability, flexibility, and extensibility (Yurdugul et al., Citation2012). The key role of cellulase in the baking industry can be hydrolysis of pentosans, which are a small component of wheat flour but play the main role of high water-binding capacity in dough rheology and bread quality. Soluble forms of pentosans are involved in the dough stretchiness, and the hydrolysis of these insoluble pentosans is important to influence the gluten network formation and the improvement of bread elasticity due to the release of water. This further affects the viscosity, proofing capacity and water-binding capacity of dough as well as it improves freshness, aroma, texture, bread volume, anti-staling properties and grain structure of the finished bakery product (Yurdugul et al., Citation2012).
In the present study, the use of a combination of enzymes gives better results than its sole use because of their synergetic effect. The published reports indicates that the combined use of two hydrolytic enzymes like cellulase and amylases displays better bread quality, loaf volume, storage stability and softer crumb production (Kostyuchenko et al., Citation2021). Amylase enzymes used in the baking industry have been reported extensively as anti-staling and standardizing agents of flour. The addition of amylase increases the fermentable sugar level in the dough, thus promoting the fermentation of yeast, which increases crust color and bread flavor (Sanz-Penella et al., Citation2014).
Since in the baking industry, the pH of dough is acidic, acid-stable enzymes are required. In the present study, we describe the purification and characterization of acid-stable cellulase enzyme from Bacillus licheniformis species and its application as a better option for whole wheat bread preparation. Also, the purified cellulase enzyme was combined with the amylase enzyme for better bread preparation.
2. Materials and methods
2.1. Chemicals
All media components used in this research were purchased from Himedia, Mumbai. carboxymethylcellulose (CMC), 3, 5-dinitrosalicylic acid (DNSA), Congo red dye, reagents, and all other chemicals purchased from the SRL were of analytical grade.
2.2. Sample collection
Soil samples were collected from the root of the mangrove plants from Narara Beach at Jamnagar, Gujarat, India. Approximately 5 g of soil sample was taken from the five different locations near to Narara Beach. Soil sample collection was done as per the composite soil sampling technique. Briefly, the collected soil samples were pooled and melded to form a soil sample. All collected samples were transferred to the microbiology laboratory of Marwadi University, Rajkot, and stored at 4°C until further processing.
2.3. Isolation of acidophilic bacteria
Samples were diluted serially and inoculated on modified Norris Media (Norris et al., Citation1996) plate containing (g/l): MgSO4•7 H2O (0.5%), K2HPO4 (0.2%), (NH4)2SO4 (0.4%), NaCl (0.1%), KCl (0.1%), FeSO4•7 H2O (0.1%), yeast extract (10%), and gelatin gellan gum (20%). Plates were adjusted to different pH (3.0, 3.5, and 4.0) by using 0.1N HCl. The plates were incubated at 37°C for 4–6 days, and the plates were showing bacterial colonies with different morphological characteristics. All bacterial colonies were purified and preserved at 4°C for further investigation.
2.4. Screening for enzymatic potential
To study the enzyme-producing ability, the isolates were screened for cellulase enzyme production on the modified Norris media plate containing (g/l): 10% CMC and its pH 4.0 adjusted with 0.1 N HCl. The plates were incubated for 24 and 48 h at 37°C. Then, the plates were stained with Congo red dye (0.1%) for 15–20 min and then the plates were washed using 1 M sodium chloride for 20 min. The results were observed by the formation of a zone of clearance around cellulase-producing colonies. The zone diameters were measured. Furthermore, based on the zone of inhibition, strains were selected for further studies. Isolated strains were further observed for morphological and staining characteristics.
2.5. Molecular identification of cellulase producer isolates
Bacterial genomic DNA was extracted from the studied isolates by using our laboratory’s optimized genomic DNA extraction protocol (unpublished data). Bacterial barcode gene (16S rRNA) was amplified by PCR using universal primer 63F (5ʹ CAGGCCTAACACATGCAAGTC 3ʹ) and 1387 R (5ʹGGGCGGWGTGTACAAGGC 3ʹ) followed by sequencing of the PCR products for the identification of the isolates. The PCR product was purified using a PCR clean-up kit (Nucleo-pore, Genetix Biotech Asia), and purified products were sequenced at Eurofins Genomics, India. The sequenced data were subjected to BLAST (NCBI) for the identification of the isolates.
2.6. Enzyme production by submerged fermentation
Cellulase enzyme production was carried out in liquid Norris broth supplemented with 10% CMC at pH 4.0. The broth was inoculated with an activated culture and incubated in shaking conditions for 8 days at 120 rotation per minute (rpm) at 37°C. The incubated broth was analyzed for enzyme production regularly after 24 h, where the 10 ml of sample was centrifuged for 25 min(min) at 6,500 rpm under cooling conditions at 4°C. The collected upper layer was used as a crude enzyme for an enzyme detection assay.
2.7. Cellulase enzyme assay
Cellulase activity was assayed by using the DNSA method (Miller, Citation1959). Briefly, reaction mixture composed of enzyme solution (0.5 ml) was added to 0.5 ml CMC (1%) prepared in 0.05 M sodium acetate buffer with pH 4.0. The mixture was incubated for 20 min at 37°C followed by adding 1 ml DNSA solution. The enzyme activity was measured at 540 nm using a spectrophotometer. The enzyme unit is expressed as 1 µmol of product liberated in a minute under the standard assay protocol.
2.8. Cellulase enzyme purification
2.8.1. Precipitation and dialysis method
The partial purification of the enzyme was carried out by using the ammonium sulphate precipitation method. The active culture of the isolate was inoculated in the modified Norris broth with pH 3.5 and incubated for 24–48 h at 37°C. After incubation, the broth was centrifuged for 20 min at 6,000 rpm under cooling conditions (4°C). The crude cellulase enzyme was saturated with 80% ammonium sulphate and allowed to precipitate with constant stirring for 24 h at 4°C. The saturated broth was centrifuged for 25 min at 6,500 rpm at 4°C. The supernatant and pellets were separated. Pellets obtained were resuspended in 0.05 M sodium acetate buffer (pH-4.0). Pellets were dialyzed against the same buffer (pH-4.0) using a dialysis membrane at 4°C for 24 h and checked for enzyme activity and protein concentration (Lowry et al., Citation1959).
2.8.2. Purification of cellulase enzyme by size exclusion chromatography
Dialyzed enzyme was purified by using size exclusion chromatography. The partially purified enzyme was loaded on the manually packed column consisting of matrix BioGel P60. The 1 ml fractions were collected up to 40 fractions, followed by quantifying enzyme activity and protein concentration.
2.8.3. Molecular mass determination by SDS-PAGE
SDS-PAGE profiling was done to determine the molecular weight of the purified cellulase enzyme by using the method by Laemmli (Citation1970). SDS-PAGE with a stacking gel (4% polyacrylamide) and a separating gel (12% polyacrylamide) was used, and protein detection was done with Coomassie Brilliant Blue R-250.
2.9. Cellulase characterization
2.9.1. Effect of pH and temperature on cellulase enzyme activity and stability
The effect of pH on cellulase activity was determined using 0.05 M of sodium acetate buffer (3.0–5.0 pH), phosphate buffer (6.0–7.0 pH), and Tris buffer (7.0–8.0 pH). The enzyme was incubated in different pHs of buffers for 20 min at 37°C. The effect of temperature on cellulase enzyme activity was determined by incubating the enzyme at different reaction temperatures: 37°C, 40°C, 50°C, and 60°C for 20 min. After incubation, the enzyme activity was measured at 540 nm by using DNSA method. The pH and temperature stability were determined by incubating the enzyme at a temperature of different temperature for 30-min time intervals till 360 min. After every 30 min, an enzyme assay was performed, and the same protocol was repeated till a downward trend in the enzyme activity was observed. The pH stability was determined using different buffers, and the enzyme activity was measured by incubating the enzyme in the same buffer for 30 min till a downward trend in the enzyme activity is observed.
2.9.2. Effect of metal ions and chelators on cellulase enzyme activity
To determine the effect of metal ions on the cellulase enzyme activity, various metal ions such as MnCl2, ZnCl2, CoCl2, MgCl2, FeCl3, CaCO3, CuSO4, MgSO4, ZnSO4, FeSO4, and MnSO4 and chelators such as EDTA and SDS at a final concentration of 5 and 10 mM of the solution were added to the enzyme assay mixture, and the cellulase enzyme activity assay was performed at 37°C in 0.05 M acetate buffer (pH-4.0). The cellulase enzyme activity without adding metal ions and chelators was defined as a control.
2.9.3. Application of purified cellulase in the baking industry
To study the application of purified cellulase enzymes in bakery processes, wheat flour of good life (MP Wheat Chakki Atta), active dry yeast (Blue Bird), sugar, salt, etc., were purchased from the local market, Rajkot. A cellulase enzyme was produced and purified in our laboratory at Marwadi University, Rajkot.
2.9.4. Dough formation process
The dough was prepared by using wheat flour and the addition of all dry ingredients including salt, active dry yeast, and sugar mixed and, then, following the addition of water and enzyme. Two different dough samples were treated with purified cellulase enzymes and a combination of cellulase end amylase enzymes. One dough sample without an enzyme was used as a control. After mixing, all dough samples were separately kept in a 50 ml beaker and incubated at 32°C for 2 h, and dough rising was observed.
2.9.5. Bread preparation
For bread preparation, active dry yeast (1.05 g) and sugar (1.4 g) are dissolved in lukewarm water (100° to 110° F) and covered container with plastic for 10 min to get yeast foamy or bubbly. The activated form of yeast, salt (0.7 g), amount of enzyme, and oil (1.0 ml) were added to wheat flour (35 g) and then mixed well for 5 min and then kneaded to develop soft and easy dough using water (20 ml). A total of six separate dough samples were prepared: the dough sample was treated with purified cellulase (1 ml) and a combination of cellulase (0.5 ml) and amylase (0.5 ml) enzymes respectively. Dough samples without enzymes were used as a control. Each piece of dough is hand rounded, folded, tucked inside, and shaped in round loaves. Dough samples were placed in a greased bowl, covered with a wet cloth, and allowed to be proofed or fermented for 2 and 24 h at 30°C. After proofing, doughs were pressed lightly by hand to remove air and again folded, kneaded, rounded, and then allowed at room temperature for 30 min for proper rising. After that, dough loaves were baked in the oven at 180°C for 30–40 min. After baking, loaves of bread were removed from the oven and cooled for 2 h at room temperature. Pieces of bread were separately kept in a plastic pouch and stored at 25°C to 27°C. The quality of fresh wheat bread samples was analyzed by measuring bread weight and height.
2.9.6. Textural, physical, and nutritional characteristics of bread
Textural characteristics including hardness (height), adhesiveness, springiness (elasticity), cohesiveness, gumminess, chewiness, and resilience of each bread were analyzed by using the texture analyzer at the Central Institute of Post-Harvest Engineering & Technology (ICAR), Ludhiana 141,004, Punjab. The sensory attributes, including crust, aroma, shape, internal texture, taste, appearance, and general acceptability, were evaluated by a semi-trained 10-member panel, using a 9-point Hedonic scale with 1 representing the least score (dislike extremely) and 9 the highest score (like extremely). Physical attributes of the final product (baked breads) were sent to Gujarat food labs, Ahmedabad, Gujarat, India, for quantification of the proteins, fat, ash content, starch and fiber content.
2.9.7. Statistical analysis
All experiments were conducted in triplicates and the standard deviation was calculated in Microsoft excel. Furthermore, statistical analyses were also done by Duncan’s Multiple Range Test (DMRT) to find the significance of test variables.
3. Results and discussion
3.1. Isolation and morphological characterization
A total of 11 different bacterial strains (AI1 to AI11) were isolated as acidophilic stains from the soil of the mangrove plants of Narara Beach at Jamnagar, Gujarat, India. All isolates were able to grow at pH 4.5. Amongst all, only seven isolates that can grow at pH 4.0 were selected and screened for cellulase enzyme-producing activity, and isolates AI11 were observed as they produced a significant amount of acid-stable cellulase. The colony characteristics and staining behaviors of this isolate revealed that this bacterial strain is white pigmented, rod-shaped, opaque, gram-positive, and motile (Suppl. Fig. S1).
3.2. Molecular identification of bacterial isolate
The primer used for the 16S rRNA gene amplification gave a 1,324-bp length of PCR product, and from the product, we obtained approximately 1,230-bp good sequencing results. The bacterial isolate was identified as B. licheniformis as per the NCBI blast. The GenBank submission number for the submitted sequence of B. licheniformis is OM009286.1
3.3. Cellulase enzyme production and purification
After the production of cellulase in liquid Norris broth, the enzyme activity for the isolated strains B. licheniformis was studied with pH-4.0 at 37°C.
3.4. Purification
Partial purification of the enzyme was carried out by the ammonium sulphate precipitation method. The pellet obtained from the isolated species B. licheniformis at 40% saturation has 3.372 specific activity, 1.347-fold purity, and 1.063% yield (Table ). The results indicate that 40% of precipitated pellets obtained from B. licheniformis show a significant cellulase activity. The partially purified pellets were further dialysed using the dialysis membrane (Himedia LA395) in3 mM sodium acetate buffer (pH-4.0). The dialyzed cellulase enzyme was loaded on the size exclusion column (Biogel p-60), and a fraction of 1 ml was collected and analyzed. The fraction no. 21 of an enzyme from B. licheniformis showed 13.216 specific activity and 5.280-fold purity (Table ).
Table 1. Purification summary of cellulase enzyme from Bacillus licheniformis
3.5. Molecular mass determination by SDS-PAGE
SDS-PAGE of purified enzymes shows a single band. The molecular weight of purified cellulase was 20 kDa after comparing it with the molecular weight marker (Figure ). The similar molecular weight of cellulase is reported in B. licheniformis 380 species (Marco et al., Citation2017). As reported by Furigo and Pereira (Citation2001), low-molecular-weight cellulase enzymes tend to be more stable compared to higher-molecular-weight enzymes. This stability property can be correlated with the presence of disulphide linkages (Furigo & Pereira, Citation2001).
3.6. Characterization of cellulase enzyme
3.6.1. Effect of pH and temperature on cellulase activity
The purified cellulase enzyme was active in a broad pH range (3.0–8.0). The highest enzyme activity (489.40 U/ml) was reported at 37°C temperature with an optimum pH value of 4.0 (Figure ). The lowest cellulase enzyme activity (454.38 U/ml) was observed at pH-8. Similar results were reported with optimum pH-4.5 and pH-5 using Paenibacillus sp. Y2 (Lee et al., Citation2017), Bacillus sp. K-11 (Caf et al., Citation2014), and B. licheniformis JK7 (Seo et al., Citation2013). Furthermore, the pH stability of the cellulase enzyme also displays that the enzyme is stable in a broad pH range (Figure ) (Suppl. Table S1and S2). These results describe that the cellulase enzyme is acid stable and has a good enzymatic potential in acidic conditions. Previous reported papers also indicate that cellulase enzymes from diverse Bacillus sp. are active in a broad pH range and used in different industrial applications (George et al., Citation2001; Lee et al., Citation2008; Mawadza et al., Citation2000). The cellulase activity and stability have direct correlation with the industrial application of the enzyme. The activity and stability at pH 4.0 resembles with most of the hydrolytic enzymes, which are composed of two acid side chains – one protonated and other deprotonated. Changes in the protonation status strongly reduces the catalytic efficiency, resulting it in more stable and active at acidic pH (Harris & Turner, Citation2002).
Figure 2. (a) Effect of pH on purified cellulase enzyme stability. (b) Effect of temperature on purified cellulase stability. (c) Effect of temperature on purified cellulase enzyme. (d) Effect of pH on purified cellulase activity
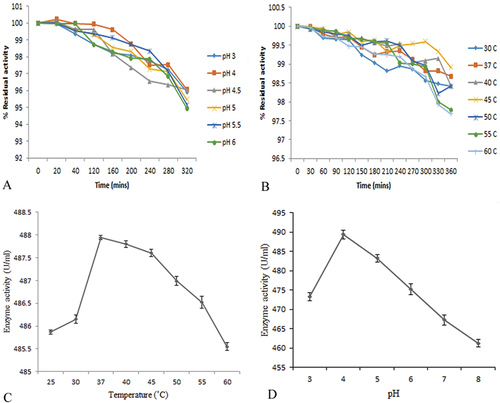
The impact of temperature on cellulase enzyme activity was determined at a different temperature range from 37°C to 60°C. The optimum pH of the purified enzyme is (487.98 U/ml) at 37°C temperature, while the lowest enzyme activity (377.94 U/ml) was observed at 60°C. The results are summarized in Figure . The purified cellulase enzyme activity gradually decreases with an increase in temperature from 37°C to 60°C. A similar study of acid-stable cellulase from Bacillus sp. K-11 was reported (Caf et al., Citation2014). The purified cellulase was stable for more than 5 h (Figure ) (Suppl. Table S3 and S4). The stability at acidic and high thermal conditions indicates the presence of more disulphide bonds in the cellulase composition. The stability and activity at high temperatures can be attributed to changes in physical structure of cell membrane due to which it can increase the extracellular secretion of stable and active enzymes (Sethi et al., Citation2013). Furthermore, similar results were also reported with Bacillus subtilis strain LFSS3, Bacillus strain CH43, and HR68 (Fu et al., Citation2010; Mawadza et al., Citation2000). Also, a few published articles indicate the production of the acidophilic cellulase having stability in the temperature range of 30°C to 40°C (Asha & Sakthivel, Citation2014).
3.6.2. Effect of metal ions and chelators on cellulase activity
To examine the impact of various metal ions and chelators on cellulase enzyme activity, a cellulase enzyme assay was performed with pH 4.0 using the CMC as substrate and the addition of metal ions and chelators at a concentration of 5 and 10 mM. The purified cellulase enzyme from B. licheniformis showed the highest enzyme activity 488.78 U/ml, 389.59 U/ml, and 379.72 U/ml by CuSO4, MgSO4, and MgCl2 at 5 mM concentration (Table ). Using the 10 mM concentration, the enzyme activity increased to 412.87 U/ml and 405.85 U/ml using CuSO4 and MgCl2, respectively. Our findings are similar to the reported study of the acidophilic cellulase EG-PY2 from Paenibacillus sp. Y2 (Rawat & Tewari, Citation2012), Bacillus sonorensis HSC7 (Azadian et al., Citation2017), and Bacillus subtilis cellulase (Rawat & Tewari, Citation2012). Cellulase enzyme activity was enhanced moderately in the presence of metal ions CaCu3, FeCl3, CoCl2, FeSo4, and EDTA, while the cellulase enzyme activity was inhibited by metal ions MnCl2, ZnCl2, ZnSo4, MnSo4, and SDS at 5 and 10 mM concentrations. The results are summarized in Table .
Table 2. Bread attribute testing parameters
Table 3. Nutritional composition analysis of formulated bread samples
Similar results have been reported with metal ions with Zn2+ and Mn2+ inhibiting the cellulase enzyme activity, especially from Bacillus sp. (Cheng et al., Citation2016). The inhibitory effect of Zn2+ions are correlated with the binding site of the enzyme, which has the presence of a thiol group. Also, many studies have reported that metal ion Cu2+ moderately inhibits the cellulase enzyme activity (Bollaín et al., Citation2005; Kanchanadumkerng et al., Citation2017).
3.7. Application in bread preparation
3.7.1. Dough preparation and observation
The dough rising (proofing) was observed and measured by the addition of purified cellulase and a combination of enzymes (cellulase and amylase) in the dough. Several studies reported that dough with cellulase affects proofing capacity, water-binding capacity, bread taste, texture, freshness, and aroma (Yurdugul et al., Citation2012). In the present study, the dough treated with purified cellulase and a combination of cellulase + amylase enzymes resulted in a higher and good rising of dough than the control dough sample. Dough treated with combined enzyme and after incubation showed enhanced dough proofing than dough treated with single cellulase enzyme and control (Figure ). The dough was initially moistened and kept at 2 and 24 h for rising (Figures ). The dough was kept for further observation for its rising and efficient mixing of enzymes with dough (Figures ). The results indicate that the purified cellulase enzyme from B. licheniformis strain and cellulase + amylase combined mixture of enzymes have a great potential for application in the backing industry (Figure ).
3.7.2. Textural characteristics of bread
In the present study, the purified cellulase enzyme and a combination of two different enzymes (cellulase + amylase) were used in bread formation for 2 & 24 h. Each bread sample was analyzed for textural characteristics using a texture analyzer. The important parameters such as hardness, adhesiveness, springiness, cohesiveness, gumminess, chewiness, and resilience were analyzed and measured. The hardness of the bread is the main parameter used to measure bread staling, which demonstrates the force required for bread compression. Bread dough treated with cellulase enzyme showed the lowest value of bread hardness as compared to the control bread sample (without enzyme). However, the addition of combined enzymes (cellulase and amylase) more effectively reduces the hardness of the crumb as compared to the control (Figure ). Adhesiveness is generally assessed during the adhesion or separation of two flat plates or the surface of the sample. According to textural properties, adhesiveness was interrelated with hardness and other characteristics like gumminess and chewiness (Zghal et al., Citation2001). Thus, with increasing hardness, adhesiveness will increase. Adhesiveness was observed to decrease with the addition of purified cellulase and combined (cellulase + amylase) enzymes in bread dough as compared to the control sample. Reduction in hardness and adhesiveness can be correlated with the presence of the miscible or immiscible cell wall material in high concentration, leading to the decrease in hardness and also indirectly affecting the gas-holding capacity of the dough (Rizzello et al., Citation2012). Additionally, the decrease in hardness can also be correlated with the particle size of raw material used for the bread baking (Salmenkallio-Marttila et al., Citation2001).
Primary parameters of texture profile such as springiness and cohesiveness were analyzed. Cohesiveness indicates how long the product can last compared to the performance under the first deformation (Yurdugul et al., Citation2012). In the present findings, there are no significant changes observed in springiness and cohesiveness by the addition of cellulase enzymes. However, the bread dough treated with combined enzymes showed increased values of springiness and cohesiveness than the control. The findings indicate that the combination of amylase and cellulase degrades the carbohydrates and helps in increasing elasticity and its binding efficiency. These results can be directly correlated with the presence of gluten and amylose molecules in the wheat bread. The amylose molecule has the property to reorder and re-associate during the cooling process (Chen et al., Citation2021).
The secondary parameters of texture profiles such as gumminess and chewiness were also analyzed, which mainly depend on the hardness of the bread. Bread treated with cellulase enzyme showed similar values for gumminess and resilience in control and treated samples, while chewiness was enhanced in bread treated with a combination of cellulase and amylase enzymes (Figure ). Published reports indicate that the resilience as an important factor for recovery rate after deformation represents freshness and mechanical strength of the bakery product (Røjel et al., Citation2020).
3.7.3. Bread attributes
As shown in Table , bread treated with cellulase and a combination of amylase resulted in an improved effect on bread attributes such as volume, form symmetry, texture, and color of bread along with its aroma and taste. The bread sample with cellulase increased its scores by 10.2 (volume), 4.15 (symmetry of form), 3.75 (texture), 4.5 (colour), 3.88 (aroma), and 7.19 for the taste of bread in comparison to the control sample. However, bread treated with cellulase and amylase mixture increased higher scores by 13.25 (volume), 4.62 (symmetry of form), 4.10 (texture), 4.65 (colour), 4.01 (aroma), and 8.82 for taste of bread in comparison to control and cellulase-treated bread sample. These results demonstrate that the use of cellulase enzyme and a mixture of cellulase and amylase use in bread preparation leads to better bread quality (Table ). The increase in the symmetry, colour, and texture can strongly depend on the stability of gas cells during the bread-making process. Due to the presence of soluble proteins and sugars, mostly pentosans participate in the improvement of the bread attributes along with their sensory and rheological attributes (Rouillé et al., Citation2005). However, few reports also suggest the antagonist role of sugar and gas cell formation during the bread preparation using different flours (Zghal et al., Citation2001).
Table 4. Effect of metal ions (concentration 5 mM) on enzyme activity (U/ml)
The nutritional composition of wheat flour and bread with cellulase (Formulation-1) and bread with the combination of cellulase with amylase (Formulation-2) is presented in Table . Wheat flour showed the highest content of protein (10.9), fat (1.7), and starch (70) than bread with cellulase and bread with cellulase and amylase. However, bread with cellulase and cellulase with amylase showed the highest contain ash (1.6 & 1.72), insoluble fiber (5.2 & 6.2), and soluble fiber (1.35 & 1.52), respectively, than wheat flour. An increase in the ash content could be due to the higher breakage of the active biomolecules like gluten and carbohydrates present in the wheat dough in comparison to its counter control wheat flour part. Also, the noteworthy increase in the protein, fat, and starch contents can be due to their increase in nutritional quality compared to control wheat flour. The present findings can also be correlated with the previous published reports where plantain flour was used as a substitute for wheat flour for bread preparation (Olaoye et al., Citation2006).
Table 5. Effect of metal ions (concentration: 10 mM) on enzyme activity (U/ml)
4. Conclusion
In the baking industry, hydrolytic enzymes have gained great attention due to their potential effect on improving dough and bread quality. Among the hydrolytic enzymes, a combination of cellulase and amylase was used in the present study as potential bread attribute enhancers. The final baked product displays better aroma, cohesiveness, hardness, and crumbness. The present study has showcased a combination of cellulase and amylase enzymes. Additionally, a combination of other hydrolytic enzymes and their role in the food industry would be interesting for further study.
Acknowledgments
All authors are thankful to the Government of Gujarat for supporting the research conducted in the manuscript. The authors are also thankful to Marwadi University for providing infrastructure for conducting the research.
Disclosure statement
No potential conflict of interest was reported by the authors.
Data availability statement
All authors of the manuscript declare that the raw data will be available on request.
Additional information
Funding
Notes on contributors
Jasmita Chauhan
Ms. Jasmita Chauhan is a PhD research fellow of the Department of Microbiology, Marwadi University. Her research interest includes: Extremophiles and it potential applications in Food and Bakery industries.
Rushit Shukla
Dr. Rushit Shukla is serving as Assistant professor at Department of Microbiology, Christ College, Rajkot, Gujarat, India. Dr. Rushit Shukla research area includes: Actinomycetes, Applied Microbiology, Microbial Molecular Biology and Microbial Diversity.
Ashok Kumar Bishoyi
Dr. Ashok Kumar Bishoyi is serving as Associate Professor at Department of Microbiology, Marwadi University, Rajkot, India. Dr. Ashok Bishoyi research area includes: Molecular marker Analysis, Genetic Diversity Study, Microbial Diversity and Phylogeny, DNA Barcoding and Fingerprinting.
Subhanshu Goyal
Dr. Subhanshu Goyal is serving as Assistant Professor at Department of Mathematics, Marwadi University, Gujarat, India. Dr. Subhanshu Goyal research area includes: Machine learning, Optimization Techniques, Advanced Statistical Analysis, Image Processing and Analysis.
Gaurav Sanghvi
Dr. Gaurav Sanghvi is serving as Associate Professor at Department of Microbiology, Marwadi University, Rajkot, Gujarat, India. His research area includes: Extremophiles, Enzymology, Mycology and Application of enzymes in Food, Pharma and Cosmetic Industries.
References
- Adhyaru, D. N., Bhatt, N. S., & Modi, H. A. (2015). Optimization of upstream and downstream process parameters for cellulase-poor-thermo-solvent-stable xylanase production and extraction by Aspergillus tubingensis FDHN1. Bioresour and Bioprocess, 2(1), 1–17. https://doi.org/10.1186/s40643-014-0029-1
- Asha, B. M., & Sakthivel, N. (2014). Production, purification and characterization of a new cellulase from Bacillus subtilis that exhibit halophilic, alkalophilic and solvent-tolerant properties. Annals of Microbiology, 64(4), 1839–1848. https://doi.org/10.1007/s13213-014-0835-x
- Azadian, F., Badoei-Dalfard, A., Namaki-Shoushtari, A., Karami, Z., & Hassanshahian, M. (2017). Production and characterization of an acido-thermophilic, organic solvent stable cellulase from Bacillus sonorensis HSC7 by conversion of lignocellulosic wastes. Journal of Genetic Engineering & Biotechnology, 15(1), 187–196. https://doi.org/10.1016/j.jgeb.2016.12.005
- Back, S. C., & Kwon, Y. J. (2007). Optimization of the pretreatment of rice straw hemicellulosic hydrolyzates for microbial production of xylitol. Biotechnology and Bioprocess Engineering: BBE, 12(4), 404–409. https://doi.org/10.1007/BF02931063
- Bhat, M. K., & Bhat, S. (1997). Cellulose degrading enzymes and their potential industrial applications. Biotechnology Advances, 15, 583–620. https://doi.org/10.1016/S0734-9750(97)00006-2
- Bollaín, C., Angioloni, A., & Collar, C. (2005). Bread staling assessment of enzyme-supplemented pan breads by dynamic and static deformation measurements. European Food Research & Technology, 220(1), 83–89. https://doi.org/10.1007/s00217-004-1059-2
- Caf, Y., Valipour, E., & Arikan, B. (2014). Study on cold-active and acidophilic cellulase (CMCase) from a novel psychrotrophic isolate Bacillus sp. K-11. International Journal of Current Microbiology and Applied Sciences, 3(5), 16–25.
- Chen, Y., Eder, S., Schubert, S., Gorgerat, S., Boschet, E., Baltensperger, L., Windhab, E. J., Städeli, C., Kuster, S., Fischer, P., & Windhab, E. J. (2021). Influence of amylase addition on bread quality and bread staling. LWT - Food Science & Technology, 1(6), 1143–1150. https://doi.org/10.1021/acsfoodscitech.1c00158
- Cheng, J., Huang, S., & Jiang, H. (2016). Isolation and characterization of a non-specific endoglucanase from a metagenomic library of goat rumen. World Journal of Microbiology & Biotechnology, 32(1), 12. https://doi.org/10.1007/s11274-015-1957-4
- Eida, M. F., Nagaoka, T., Wasaki, J., & Kouno, K. (2009). Isolation and characterization of cellulose-decomposing bacteria inhabiting sawdust and coffee residue composts. Microbes & Environments / JSME, 1202170356. https://doi.org/10.1264/jsme2.ME11299
- Fu, X., Liu, P., Lin, L., Hong, Y., Huang, X., Meng, X., & Liu, Z. (2010). A novel endoglucanase (Cel9p) from a marine bacterium Paenibacillus sp. BME-14. Applied Biochemistry and Biotechnology, 160(6), 1627–1636. https://doi.org/10.1007/s12010-009-8648-2
- Furigo, A., & Pereira, E. B. (2001). Enzimas e suas aplicações: Cinética enzimática. Universi dade Federal de Santa Catarina. Florianópolis, 39(4).
- George, S. P., Ahmad, A., & Rao, M. B. (2001). Studies on carboxymethyl cellulase produced by an alkalothermophilic actinomycete. Bioresource Technology, 77(2), 171–175. https://doi.org/10.1016/S0960-8524(00)00150-4
- Goel, N., Patra, R., Verma, S. K., & Sharma, P. C. (2019). Purification and characterization of cellulase from Pseudomonas sp. isolated from waste dumping site soil J appl. Biotechnology and Bioengineering, 6(3), 118–124. https://doi.org/10.15406/jabb.2019.06.00183
- Harada, O., Lysenko, E. D., Edwards, N. M., & Preston, K. R. (2005). Effects of commercial hydrolytic enzyme additives on Japanese‐style sponge and dough bread properties and processing characteristics. Cereal Chemistry, 82(3), 314–320. https://doi.org/10.1094/CC-82-0314
- Harjunpää, V., Helin, J., Koivula, A., Siika-Aho, M., & Drakenberg, T. (1999). A comparative study of two retaining enzymes of Trichoderma reesei: Transglycosylation of oligosaccharides catalysed by the cellobiohydrolase I, Cel7A, and the β-mannanase, Man5A. FEBS Letters, 443(2), 149–153. https://doi.org/10.1016/S0014-5793(98)01692-5
- Harris, T. K., & Turner, G. J. (2002). Structural basis of perturbed pKa values of catalytic groups in enzyme active sites. IUBMB Life (International Union of Biochemistry and Molecular Biology: Life), 53(2), 85–98. https://doi.org/10.1080/15216540211468
- Henrissat, B., Teeri, T. T., & Warren, R. (1998). A scheme for designating enzymes that hydrolyse the polysaccharides in the cell walls of plants. FEBS Letters, 425, 352–354. https://doi.org/10.1016/S0014-5793(98)00265-8
- Horn, S. J., Vaaje-Kolstad, G., & Westereng, B. (2012). Novel enzymes for the degradation of cellulose. Biotechnology for Biofuels, 5(1), 45. https://doi.org/10.1186/1754-6834-5-45
- Kanchanadumkerng, P., Sakka, M., Sakka, K., & Wiwat, C. (2017). Characterization of endoglucanase from Paenibacillus sp. M33, a novel isolate from a freshwater swamp forest. Journal of Basic Microbiology, 57(2), 121–131. https://doi.org/10.1002/jobm.201600225
- Karim, L. (2020). Stability of acidophile endoglucanase in ionic liquids. International Journal of Innovative Research, 7, 271–280. http://ijiset.com/vol7/v7s2/IJISET_V7_I2_29.pdf
- Kim, Y. K., Lee, S. C., Cho, Y. Y., Oh, H. J., & Ko, Y. I. (2012). Isolation of cellulolytic Bacillus subtilis strains from agricultural environments. ISRN Microbiology, 1–9. https://doi.org/10.5402/2012/650563
- Kostyuchenko, M., Martirosyan, V., Nosova, M., Dremucheva, G., Nevskaya, E., & Savkina, O. (2021). Effects of α-amylase, endo-xylanase and exoprotease combination on dough properties and bread quality. Agronomy Research, 1234–1248. https://doi.org/10.15159/AR.21.067
- Laemmli, U. K. (1970). Cleavage of structural proteins during the assembly of the head of bacteriophage T4. Nature, 227(5259), 680–685. https://doi.org/10.1038/227680a0
- Lakhundi, S., Siddiqui, R., & Khan, N. A. (2015). Cellulose degradation: A therapeutic strategy in the improved treatment of Acanthamoeba infections. Parasites & Vectors, 8(1), 1–16. https://doi.org/10.1186/s13071-015-0642-7
- Lee, Y. J., Kim, B. K., Lee, B. H., Jo, K. I., Lee, N. K., Chung, C. H., Lee, Y. C., & Lee, J. W. (2008). Purification and characterization of cellulase produced by Bacillus amyoliquefaciens DL-3 utilizing rice hull. Bioresource Technology, 99(2), 378–386. https://doi.org/10.1016/j.biortech.2006.12.013
- Lee, J. P., Seo, G. W., An, S. D., & Kim, H. (2017). A cold-active acidophilic endoglucanase of Paenibacillus sp. Y2 isolated from soil in an alpine region. Journal of Applied Biological Chemistry, 60(3), 257–263. https://doi.org/10.3839/jabc.2017.041
- Lowry, O. H., Resebriugh, N. J., Farr, A. L., & Randall, R. J. (1959). Protein measurement with the folin phenol reagent. The Journal of Biological Chemistry, 193(1), 265–275. https://doi.org/10.1016/S0021-9258(19)52451-6
- Maijala, P. (2000). Heterobasidion annosum and wood decay: enzymology of cellulose, hemicellulose, and lignin degradation. [ Dissertation], University of Helsinki.
- Maki, M., Leung, K. T., & Qin, W. (2009). The prospects of cellulase-producing bacteria for the bioconversion of lignocellulosic biomass. International Journal of Biological Sciences, 5, 500. https://doi.org/10.7150/ijbs.5.500
- Marco, É. G., Heck, K., Martos, E. T., & Van Der Sand, S. T. (2017). Purification and characterization of a thermostable alkaline cellulase produced by Bacillus licheniformis 380 isolated from compost. Anais da Academia Brasileira de Ciências, 89(3 suppl), 2359–2370. https://doi.org/10.1590/0001-3765201720170408
- Mawadza, C., Hatti-Kaul, R., Zvauya, R., & Mattiasson, B. (2000). Purification and characterization of cellulases produced by two Bacillus strains. Journal of Biotechnology, 83(3), 177–187. https://doi.org/10.1016/S0168-1656(00)00305-9
- Miller, G. L. (1959). Use of dinitrosalicylic acid reagent for determination of reducing sugar. Analytical Chemistry, 31(3), 426–428. https://doi.org/10.1021/ac60147a030
- Nagendran, S., Hallen-Adams, H. E., Paper, J. M., Aslam, N., & Walton, J. D. (2009). Reduced genomic potential for secreted plant cell-wall-degrading enzymes in the ectomycorrhizal fungus Amanita bisporigera, based on the secretome of Trichoderma reesei. Fungal Genetics & Biology: FG & B, 46, 427–435. https://doi.org/10.1016/j.fgb.2009.02.001
- Norris, P. R., Clark, D. A., Owen, J. P., & Waterhouse, S. (1996). Characteristics of Sulfobacillus acidophilus sp. nov. and other moderately thermophilic mineral-sulphide-oxidizing bacteria. Microbiology (Reading, England), 142(4), 775–783. https://doi.org/10.1099/00221287-142-4-775
- Okeke, B. C., & Lu, J. (2011). Characterization of a defined cellulolytic and xylanolytic bacterial consortium for bioprocessing of cellulose and hemicelluloses. Applied Biochemistry and Biotechnology, 163(7), 869–881. https://doi.org/10.1007/s12010-010-9091-0
- Olaoye, O. A., Onilude, A. A., & Idowu, O. A. (2006). Quality characteristics of bread produced from composite flours of wheat, plantain and soybeans. African Journal of Biotechnology, 5(11). 1102–1106. https://doi.org/10.5897/AJB06.014
- Rawat, R., & Tewari, L. (2012). Purification and characterization of an acidothermophilic cellulase enzyme produced by Bacillus subtilis strain LFS3. Extremophiles, 16(4), 637–644. https://doi.org/10.1007/s00792-012-0463-y
- Rizzello, C. G., Coda, R., Mazzacane, F., Minervini, D., & Gobbetti, M. (2012). Micronized by-products from debranned durum wheat and sourdough fermentation enhanced the nutritional, textural and sensory features of bread. Food Research International, 46(1), 304–313. https://doi.org/10.1016/j.foodres.2011.12.024
- Røjel, N., Kari, J., Sørensen, T. H., Borch, K., & Westh, P. (2020). pH profiles of cellulases depend on the substrate and architecture of the binding region. Biotechnology and Bioengineering, 117(2), 382–391. https://doi.org/10.1002/bit.27206
- Rouillé, J., Valle, G. D., Devaux, M. F., Marion, D., & Dubreil, L. (2005). French bread loaf volume variations and digital image analysis of crumb grain changes induced by the minor components of wheat flour. Cereal Chemistry, 82(1), 20–27. https://doi.org/10.1094/CC-82-0020
- Salmenkallio-Marttila, M., Katina, K., & Autio, K. (2001). Effects of bran fermentation on quality and microstructure of high-fiber wheat bread. Cereal Chemistry, 78(4), 429–435. https://doi.org/10.1094/CCHEM.2001.78.4.429
- Sanz-Penella, J. M., Laparra, J. M., & Haros, M. (2014). Impact of α-amylase during bread making on in vitro kinetics of starch hydrolysis and glycaemic index of enriched bread with bran. Plant Foods for Human Nutrition, 69(3), 216–221. https://doi.org/10.1007/s11130-014-0436-7
- Seo, J. K., Park, T. S., Kwon, I. H., Piao, M. Y., Lee, C. H., & Ha, J. K. (2013). Characterization of cellulolytic and xylanolytic enzymes of Bacillus licheniformis JK7 isolated from the rumen of a native Korean goat. Asian-Australasian Journal of Animal Sciences, 26(1), 50–58. https://doi.org/10.5713/ajas.2012.12506
- Sethi, S., Datta, A., Gupta, B. L., & Gupta, S. (2013). Optimization of cellulase production from bacteria isolated from soil. ISRN Biotechnology, 2013, 1–7. https://doi.org/10.5402/2013/985685
- Singhania, R. R., Sukumaran, R. K., Patel, A. K., Larroche, C., & Pandey, A. (2010). Advancement and comparative profiles in the production technologies using solid-state and submerged fermentation for microbial cellulases. Enzyme and Microbial Technology, 46(7), 541–549. https://doi.org/10.1016/j.enzmictec.2010.03.010
- Soeka, Y. S. (2019). Production and characterization of cellulase from the newly isolated Bacillus subtilis A8 on rice bran and corncob. IOP Conference Series: Earth and Environmental Science, 308(1), 012033. IOP Publishing. https://doi.org/10.1088/1755-1315/308/1/012033
- Yurdugul, S., Pancevska, N. A., Yildiz, G. G., & Bozoglu, F. (2012). The influence of a cellulase bearing enzyme complex from anaerobic fungi on bread staling. Rom Agriculture Research, 29, 2067–5720.
- Zghal, M. C., Scanlon, M. G., & Sapirstein, H. D. (2001). Effects of flour strength, baking absorption and processing conditions on the structure and mechanical properties of bread crumb density by digital image analysis. Cereal Chemistry, 78(1), 1–7. https://doi.org/10.1094/CCHEM.2001.78.1.1
- Zhang, X. Z., & Zhang, Y. H. P. (2013). Cellulases: Characteristics, sources, production, and applications. In Bioprocessing technologies in biorefinery for sustainable production of fuels, chemicals, and polymers (pp. 131–146). John Wiley & Sons, Inc. https://doi.org/10.1002/9781118642047.ch8