Abstract
The morphological changes in giant spherical vesicles comprised of amphilhilic poly(methacrylic acid)-block-poly(methyl methacrylate-random-methacrylic acid), PMAA-b-P(MMA-r-MAA), by incorporation of the 3-sulfopropyl methacrylate potassium salt (SpMA) into the hydrophilic PMAA block chains were investigated. The vesicles were reduced in size as the SpMA ratio increased due to the variation in the critical packing shape by the expansion of the hydrophilic surface area by the incorporation of the more hydrophilic ionic segments. The increase in the SpMA ratio also delayed the transition from the spherical vesicles to a film-like bilayer. The giant vesicles containing the SpMA segments were disrupted into a nonspecific form by the electrostatic interaction with poly(allylamine hydrochloride) at a 2.5 M ratio of the unit for the allylamine hydrochloride (AH) to the SpMA. A large excess of the AH/SpMA above 5.0 caused no disruption, but partial fusion of the vesicles accompanied by a decrease in size.
Public Interest Statement
A great variety of living things inhabit the natural world. They are quite different in form, size, and lifestyle, however, share something in common in a basic unit of their bodies. It’s a cell. A cell is a container enclosed with a thin two-layer membrane formed by surfactants of lipids. The functions of lipid bilayer membrane are closely related not only to essential life activities of living things but also to the origin of life.
A giant vesicle is a micro-sized container with a closed bilayer structure formed by surfactant and is regarded as an artificial model of biomembrane for cells based on similarities in its size and structure. This study demonstrated the maintenance of a giant vesicle structure imitating the way in living cells with the aim of creating biomimetic functions for the giant vesicles comprised of non-natural synthetic polymer surfactants.
1. Introduction
Cytoplasmic membranes have many important roles in essential life processes such as structural maintenance (Branton, Cohen, & Tyler, Citation1981), selective transport (Elston, Wang, & Oster, Citation1998; Nishi & Forgac, Citation2002), endocytosis (Anderson, Brown, & Goldstein, Citation1977; Ford et al., Citation2002; Itoh, Kigawa, Kikuchi, Yokoyama, & Takenawa, Citation2001), and signal transduction (Oancea & Meyer, Citation1998; Rizo & Sudhof, Citation1998). These functions are fulfilled by the membrane proteins embedded or anchored in the lipid bilayer matrix, or attached on the bilayer surface. The integral membrane proteins and lipid-anchored membrane proteins are fixed in the lipid bilayer through the van der Waals interaction between their hydrophobic domains and the hydrocarbon chains of the lipids (Johnson & Cornell, Citation1999; Popot & Engelman, Citation2000). On the other hand, the peripheral membrane proteins located on the bilayer surface are electrostatically bound to the polar head groups of the lipids often via the integral membrane proteins (Branton et al., Citation1981; Hyvonen et al., Citation1995; Kim, Mosior, Chung, Wu, & McLaughlin, Citation1991). While the hydrophilic head groups of the lipids serve as the binders of the membrane proteins to induce their own functions during specific situations on the bilayer, they determine the membrane curvature and cell morphology along with the hydrophobic hydrocarbon chains based on the individual critical packing shape dependent on the optimal hydrophilic surface area and hydrocarbon chain volume (Bigay & Antonny, Citation2012; Cullis & De Kruijff, Citation1979; Israelachvili, Mitchell, & Ninham, Citation1976).
Giant vesicles are regarded as artificial models for cytoplasmic membranes of cells and organelles, such as erythrocytes (Elgsaeter, Stokke, Mikkelsen, & Branton, Citation1986), mitochondria (Frey & Mannella, Citation2000), and chloroplasts (Sadava, Citation1993) based on the similarities in their size and structure. In recent years, it has been demonstrated that the micrometer-sized giant vesicles comprised of amphiphilic poly(methacrylic acid)-block-poly(methyl methacrylate-random-methacrylic acid) diblock copolymer, PMAA-b-P(MMA-r-MAA), provided new biomembrane models based on some similarities concerning the structural properties (Yoshida, Citation2015a) and response to temperature and pH (Yoshida, Citation2013, Citation2015b). The similarities also included the pore formation for ionic compounds passing across the hydrophobic phase of the vesicle bilayer (Yoshida, Citation2015c). It has also been reported that the morphology of the giant vesicles was effectively controlled by manipulating the critical packing shape of the copolymer using the block length (Yoshida, Citation2014a), molar ratio of the monomer units (Yoshida, Citation2014b, Citation2014c), and mixed composition (Yoshida, Citation2015d, Citation2015e), coupled with the physical conditions, such as the copolymer concentration, solvent affinity, and stirring speed during the photopolymerization-induced self-assembly of the copolymers into the vesicles (Yoshida, Citation2015f). The morphologies were varied to spherical, elliptical, worm-like, film-like, and villus-like forms by these factors (Yoshida, Citation2015d, Yoshida, Citation2015e, Yoshida, Citation2015g).
In the present study, the morphological changes in the giant vesicles by incorporation of ionic segments in the hydrophilic PMAA block chain and by the electrostatic interaction between the ionic segments and a polyelectrolyte were investigated. This paper describes the morphology of the giant vesicles evaluated by the incorporation of 3-sulfopropyl methacrylate potassium salt (SpMA) units into the PMAA block chain and by the electrostatic interaction between the SpMA units in the hydrophilic surface of the vesicles and a polyelectrolyte of poly(allylamine hydrochloride) (PAH).
2. Experimental
2.1. Instrumentation
The photopolymerization-induced self-assembly was performed using an Ushio optical modulex BA-H502, an illuminator OPM2–502H with a high-illumination lens UI-OP2SL, and a 500 W super high-pressure UV lamp (USH-500SC2, Ushio Co. Ltd.). 1H NMR measurements were conducted using Jeol ECS400 and ECS500 FT NMR spectrometers. Gel permeation chromatography (GPC) was performed using a Tosoh GPC-8020 instrument equipped with a DP-8020 dual pump, a CO-8020 column oven, and a RI-8020 refractometer. Two gel columns, Tosoh TSK-GEL α-M were used with N,N-dimethylformamide (30 mM LiBr and 60 mM H3PO4) as the eluent at 40°C. Field emission scanning electron microscopy (FE-SEM) measurements were performed using a Hitachi SU8000 scanning electron microscope.
2.2. Materials
Methyl methacrylate (MMA) was passed through a column packed with activated alumina to remove an inhibitor and distilled over calcium hydride. Methacrylic acid (MAA) was distilled under reduced pressure. These purified monomers were degassed with Ar for 15 min with stirring just before use. Methanol (MeOH) was refluxed over magnesium with a small amount of iodine for several hours, then distilled. 4-Methoxy-2,2,6,6-tetramethylpiperidine-1-oxyl (MTEMPO) was prepared as reported previously (Miyazawa, Endo, Shiihashi, & Okawara, Citation1985). SpMA and (4-tert-butylphenyl)diphenylsulfonium triflate (tBuS) were purchased from Sigma–Aldrich and used as received. PAH (Mn = 150,000) and 2,2′–azobis[2-(2-imidazolin-2-yl)propane] (V-61) were purchased from Nittobo and Wako Pure Chemical Industries, respectively and used without further purification. Extrapure N2 gas with over 99.9995 vol% purity and Ar gas with over 99.999 vol% purity were obtained from Taiyo Nippon Sanso Corporation.
2.3. Preparation of P(MAA-r-SpMA) end-capped with MTEMPO
V-61 (22.8 mg, 0.0911 mmol), MTEMPO (18.0 mg, 0.0966 mmol), tBuS (24.0 mg, 0.0512 mmol), MAA (2.010 g, 23.3 mmol), SpMA (58.0 mg, 0.235 mmol), and MeOH (4 mL) were placed in a 30-mL test tube joined to a high vacuum valve. The contents were degassed several times using a freeze–pump–thaw cycle and then charged with N2. The polymerization was carried out at room temperature for 5.5 h with irradiation at 9.3 ampere by reflective light using a mirror with a 500 W high-pressure mercury lamp to avoid any thermal polymerization caused by the direct irradiation (Yoshida, Citation2012). MeOH (11 mL) and distilled water (5 mL) that were degassed by bubbling Ar for 15 min were added to the product under a flow of Ar. After the product was completely dissolved in the aqueous MeOH solution, part of the mixture (ca. 1 mL) was withdrawn to determine monomer conversions and molecular weight of the P(MAA-r-SpMA) prepolymer. The solution was poured into ether (50 mL) to precipitate a polymer. The precipitate was collected by filtration and dried in vacuo for several hours to obtain a polymer (67.2 mg). The conversions of MAA and SpMA were 75% and 82%, respectively, based on 1H NMR. The molecular weight and its distribution of the prepolymer were estimated to be Mn = 21600 and Mw/Mn = 1.73 by GPC based on PMAA standards. The degree of polymerization was DP = 242 based on the molecular weight. The residual P(MAA-r-SpMA) prepolymer solution was subjected to the following polymerization-induced self-assembly.
2.4. Photopolymerization-induced self-assembly of P(MAA-r-SpMA)-b-P(MMA-r-MAA)
The solution of the prepolymer (4 mL containing the MTEMPO-capped P(MAA-r-SpMA) (0.01933 mmol), unreacted MAA (1.163 mmol), and unreacted SpMA (8.317 × 10−3 mmol)), MMA (664.6 mg, 6.638 mmol), and MAA (42.6 mg, 0.495 mmol) were placed in a 30-mL test tube joined to a high vacuum valve under a flow of Ar. The contents were degassed several times using a freeze–pump–thaw cycle and finally charged with N2. The polymerization was carried out for 7 h at room temperature and a 600-rpm stirring speed by UV irradiation at 9.3 ampere using a reflective light from a mirror. Part of the solution (ca. 0.5 mL) was withdrawn to determine the monomer conversions by 1H NMR. A mixed solvent (MeOH/water = 3/1 v/v, 20 mL) was added to the dispersion solution to precipitate aggregates. The aggregates were cleaned with the mixed solvent by a repeated sedimentation–redispersion process. The resulting aggregates were stored in the presence of a small amount of the mixed solvent.
2.5. Reaction of the giant vesicles and PAH
The giant vesicles comprised of P(MAA0.990-r-SpMA0.010)242-b-P(MMA0.841-r-MAA0.159)270 (3.1 mg containing 1.565 × 10−4 mmol of the SpMA unit) were dispersed in an aqueous MeOH solution (0.5 mL, MeOH/water = 3/1 v/v). PAH (14.6 mg, 0.1561 mmol of the allylamine hydrochloride (AH) unit) was dissolved in an aqueous MeOH solution (1 mL, MeOH/water = 3/1 v/v). A PAH solution (10 μL, 1.561 × 10−3 mmol of the AH unit) was added to the vesicle dispersion at room temperature. The mixture was vigorously shaken and stored at room temperature for 7 days to precipitate the vesicles. The precipitates were isolated by decantation and dried in air to subject to FE-SEM.
2.6. SEM observations
The aggregates were dried in air and subjected to the FE-SEM measurements at 1.0 kV without coating. The size distribution of vesicles was estimated as reported previously (Kobayashi, Uyama, Yamamoto, & Matsumoto, Citation1990).
3. Results and discussion
In order to evaluate the morphologies of giant vesicles containing ionic segments in the hydrophilic surface, amphiphilic diblock copolymers consisting of the hydrophilic PMAA block supporting the SpMA ionic units and the hydrophobic P(MMA-r-MAA) block were prepared by photopolymerization-induced self-assembly using the photo-NMP technique (Scheme ). Prior to the synthesis of the diblock copolymer, the P(MAA-r-SpMA) prepolymers end-capped with MTEMPO were prepared by the photo-NMP method in methanol using the V-61 initiator, the MTEMPO mediator, and the tBuS accelerator. The prepolymers with different SpMA contents are listed in Table . The monomer conversions were estimated by 1H NMR (Figure (a)); the SpMA conversion was determined using the signal intensity based on the protons at 4.27 ppm for the ester methylene attached to the oxygen of the unreacted monomer vs. the protons at 4.03–4.19 ppm of the polymerized unit, while the MAA conversion was based on the α-methyl protons at 1.90 ppm of the unreacted monomer vs. the protons at 0.79–2.28 ppm of the polymerized main chains. The molar ratios of the monomer units were calculated using the monomer conversions. The prepolymers contained ca. 1–10 mol% of the SpMA units. The degree of polymerization (DP) was determined on the basis of the molecular weight estimated by GPC based on PMAA standards. A decrease in the molecular weight of the copolymer with an increase in the SpMA content is due to the GPC estimation for the prepolymers with the different SpMA contents. The amphiphilic P(MAA-r-SpMA)-b-P(MMA-r-MAA) diblock copolymers were prepared by the block copolymerization of MMA and MAA employing the MTEMPO-capped P(MAA-r-SpMA) prepolymers. The resulting diblock copolymers are listed in Table . The MMA conversion was estimated by 1H NMR using the ester methyl protons at 3.74 ppm for the unreacted MMA and those at 3.54–3.70 ppm for the polymerized units in the block copolymer. The MAA conversion was determined using the α-methyl protons at 1.90 ppm for the unreacted MAA and 1.92 ppm for MMA on the basis of the MMA conversion (Figure (b)). Some copolymers slightly contained the SpMA units in the P(MMA-r-MAA) blocks due to the remaining unreacted SpMA monomers in the prepolymer solution. The DP of the P(MMA-r-MAA) block was calculated using the monomer conversions and the prepolymer concentration equal to the initial concentration of MTEMPO (Yoshida, Citation2009). The amphiphilic diblock copolymers simultaneously self-assembled into giant vesicles as the block copolymerization proceeded in an aqueous methanol solution (MeOH/water = 3/1 v/v). As shown in the GPC profiles of the block copolymer and prepolymer, the giant vesicles obtained by the photopolymerization-induced self-assembly included no prepolymer due to purification by a repeated sedimentation–redispersion process (Figure ).
Table 1. The P(MAA-r-SpMA) prepolymers end-capped with MTEMPO.
Figure 1. 1H NMR spectra of the P(MAA-r-SpMA) prepolymer (a) Pre-4 and the diblock copolymer (b) BC-41. Solvent: CD3OD-d4/CDCl3 = 3/1 (v/v).
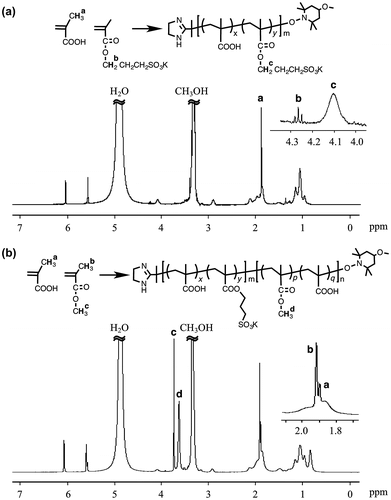
Table 2. The P(MAA-r-SpMA)-b-P(MMA-r-MAA) diblock copolymers
Figure 2. GPC profiles of the P(MAA-r-SpMA) prepolymer (a) Pre-1 and the diblock copolymer (b) BC-11.
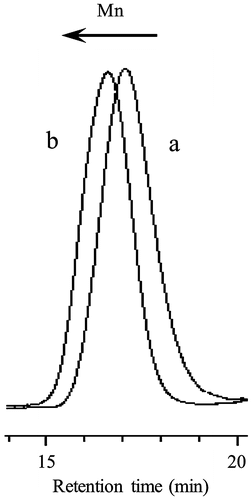
The SEM observations revealed that the morphology of the giant vesicles was dependent on the SpMA contents. Figure shows the FE-SEM images of the giant vesicles formed by the diblock copolymers. Based on the negligible differences in the DP and MMA ratio, the vesicle size (Dn) decreased with an increase in the SpMA content (Table ). This size decrease is accounted for the variation in the critical packing shape of the copolymer; a truncated cone-like critical packing shape forming the spherical vesicles changed into a more sharply truncated cone due to the expansion of the optimal hydrophilic surface area by the incorporation of the more hydrophilic SpMA units into the hydrophilic block chain (Scheme ). This more sharply truncated cone-like shape increased the curvature of the bilayer, resulting in a decreased vesicle size. It was found that the copolymer with the SpMA units over 10 mol% in the hydrophilic block chain produced large unstable vesicles having some holes along with much smaller spherical vesicles. The copolymer with a still more sharply truncated cone-like critical packing shape formed the rims of the holes that require a much higher curvature.
Figure 3. FE-SEM images of the giant vesicles obtained by the diblock copolymers with different SpMA contents; (a) BC-11, (b) BC-21, (c) BC-31 and (d) BC-41.
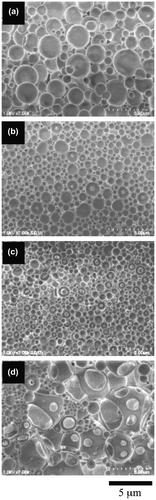
Figure 4. The morphologies of the 1.1-mol% SpMA copolymers with different MMA ratios; (a) BC-11, (b) BC-12 and (c) BC-13.
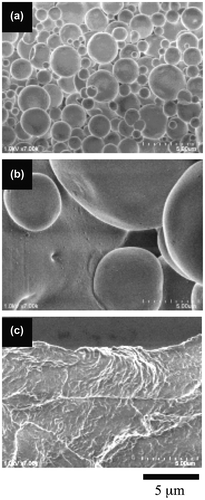
Figure 5. The morphologies of the 3.6-mol% SpMA copolymers with different MMA ratios; (a) BC-21, (b) BC-22 and (c) BC-23.
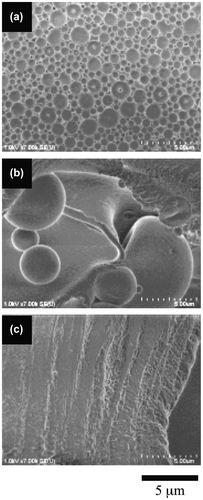
Figure 6. The morphologies of the 5.7-mol% SpMA copolymers with different MMA ratios; (a) BC-31, (b) BC-32 and (c) BC-33.
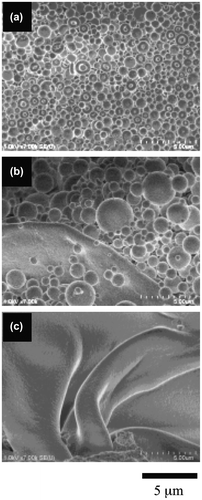
Figure 7. The morphologies of the 11.4-mol% SpMA copolymers with different MMA ratios; (a) BC-41, (b) BC-42, (c) BC-43 and (d) BC-44.
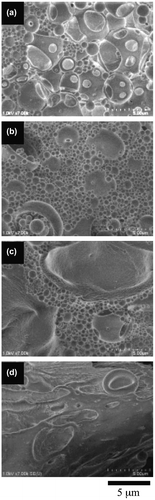
Figure 8. The morphological changes in the spherical vesicles of the 1.1-mol% SpMA copolymer (BC-11) by soaked in PAH solutions of different concentrations; AH/SpMA = (a) 0, (b) 2.5, (c) 5.0 and (d) 10.0.
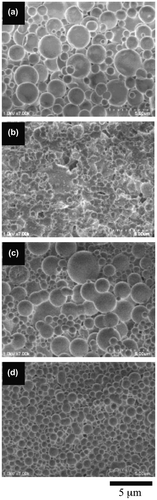
Scheme 1. Preparation of the P(MAA-r-SpMA)-b-P(MMA-r-MAA) diblock copolymers by the photopolymerization-induced self-assembly.
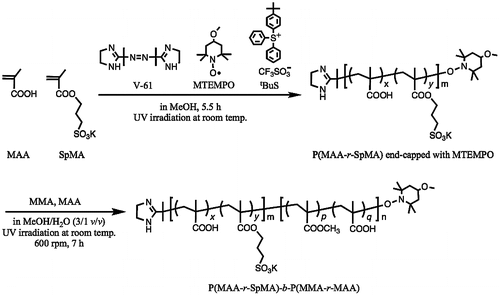
Table 3. Morphologies of the diblock copolymers
Scheme 2. Variation in bilayer curvature based on different critical packing shapes; (a) a truncated cone-like shape and (b) a more sharply truncated cone-like shape.
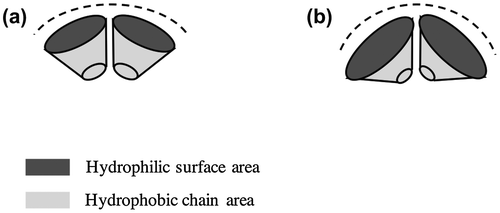
The diblock copolymers produced a difference in the morphological transition from spherical vesicles to a film-like bilayer based on their SpMA contents. Figure shows the variation in the morphology of the copolymer with a 1.1-mol% SpMA content by a decrease in the MMA ratio of the hydrophobic block. The spherical vesicles were changed into very large spherical vesicles by a 5-mol% decrease in the MMA ratio. By a further 5-mol% decrease in the MMA ratio, the large spherical vesicles were transformed into a film-like bilayer. These morphological changes were based on the variation in the critical packing shape from a truncated cone into a cylinder by the expansion of the hydrophobic volume due to a decrease in its hydrophobicity of the P(MMA-r-MAA) block. A similar transformation was observed for the vesicles of the 3.6-mol% SpMA copolymer (Figure ). On the other hand, the vesicles formed by the 5.7-mol% copolymer were still spherical by a 5-mol% decrease in the MMA units, although a flat bilayer was partly observed (Figure ). The vesicles were transformed into a flexible film-like bilayer by a further decrease in the MMA ratio. Furthermore, the vesicles with some holes for the 11.4-mol% SpMA copolymer were changed into many small spherical vesicles along with the much larger vesicles by a 5-mol% decrease in the MMA ratio (Figure ). An ca. 10-mol% decrease in the MMA ratio produced a flexible bilayer bearing the signs of fusion of the vesicles. It was deduced that an increase in the ionic segments in the hydrophilic block chains prevented the transition from the spherical vesicles to a film-like bilayer.
The human erythrocyte membrane maintains its shape by the cytoskeleton of the spectrin network via the peripheral proteins bound to the integral membrane protein and to the polar head groups of the lipids, like phosphatidylinositol and phosphatidylserine through an electrostatic interaction (Branton et al., Citation1981). The morphological changes in the spherical vesicles were investigated by the electrostatic interaction between a PAH polyelectrolyte and the SpMA units in the hydrophilic surface of the vesicles. The spherical vesicles formed by the 1.1-mol% SpMA copolymer were soaked in PAH solutions of different concentrations. The morphological changes in the vesicles by the electrostatic interaction are shown in Figure . The spherical vesicles were disrupted into a nonspecific form in the presence of PAH at a 2.5 M ratio of the unit for the allylamine hydrochloride (AH) to the SpMA. No SpMA-containing vesicles (PMAA216-b-P(MMA0.829-r-MAA0.171)318, Dn = 1.63 μm, Dw/Dn = 2.37) retained their spherical shape by the soaking in the solution with the same PAH concentration, although the vesicle size slightly decreased (Dn = 1.16 μm, Dw/Dn = 2.56). Consequently, the disruption of the SpMA-containing vesicles was caused by the insertion of PAH into the hydrophilic surface of the vesicles through the electrostatic attraction between the sulfonium anion and the allylammonium cation. On the other hand, the vesicles retained their spherical form at a 5.0 AH/SpMA ratio. The average vesicle size slightly decreased (Dn = 1.15 μm) and the size distribution was broadened (Dw/Dn = 2.40) due to the simultaneous formations of much smaller vesicles and partly fused vesicles. Furthermore, still smaller and partly fused vesicles (0.824 μm, Dw/Dn = 1.17) were produced at a 10.0 AH/SpMA ratio. These variations can be accounted because PAH at the low concentration disordered the copolymers forming a vesicle due to too short interval between the AH units interacting with the SpMA units in the hydrophilic surface (Scheme ). On the other hand, PAH at high concentration held the intervals long enough to retain the arrangement of the copolymers in the vesicle. However, PAH at still higher concentration expanded the hydrophilic surface area of the vesicles due to the insertion of the higher hydrophilic chains, resulting in a decrease in size of the vesicles. The expansion of the hydrophilic surface area of the critical packing shape of the copolymer into a more sharply truncated conical shape by PAH also caused a decrease in the vesicular size.
4. Conclusion
The morphology of the giant spherical vesicles comprised of the amphilhilic diblock copolymer was changed by the incorporation of ionic segments into the hydrophilic block chains. An increase in the ionic segment reduced the size of the vesicles. A decrease in the size was due to the expansion of the optimal hydrophilic surface area of the critical packing shape by the incorporation of the more hydrophilic ionic segments. The ionic segments also delayed the transition from the spherical vesicles into a film-like bilayer by a decrease in the MMA ratio of the hydrophobic block. Furthermore, the insertion of the polyelectrolyte into the hydrophilic surface through the electrostatic interaction caused disruption of the vesicles into a nonspecific form. Whereas a small excess of the polyelectrolyte disrupted the vesicles due to a short segment between the units binding the ionic units in the vesicle surface, its large excess retained the spherical shape, accompanied by the partial fusion of the vesicles.
Additional information
Funding
Notes on contributors
Eri Yoshida
Eri Yoshida is an associate professor at Department of Environmental and Life Sciences, Toyohashi University of Technology, Japan. She is the author of over 100 refereed articles and 20 reviews and book chapters and obtained over 20 patents. She is also an editorial board member of 7 international peer-reviewed journals. Yoshida’s research interests include: (a) molecular self-assembly of polymer surfactants, (b) controlled/living radical polymerization, (c) molecular design of functional polymers, and (d) polymer syntheses using supercritical carbon dioxide. The study in this paper concerns the molecular self-assembly of polymers prepared using the photocontrolled/living radical polymerization technique established by the author. She is a member of several professional societies.
References
- Anderson, R. G. W., Brown, M. S., & Goldstein, J. L. (1977). Role of the coated endocytic vesicle in the uptake of receptor-bound low density lipoprotein in human fibroblasts. Cell, 10, 351–364.10.1016/0092-8674(77)90022-8
- Bigay, J., & Antonny, B. (2012). Curvature, lipid packing, and electrostatics of membrane organelles: Defining cellular territories in determining specificity. Cell, 23, 886–895.
- Branton, D., Cohen, C. M., & Tyler, J. (1981). Interaction of cytoskeletal proteins on the human erythrocyte membrane. Cell, 24, 24–32. 10.1016/0092-8674(81)90497-9
- Cullis, P. R., & De Kruijff, B. (1979). Lipid polymorphism and the functional roles of lipids in biological membranes. Biochimica et Biophysica Acta (BBA)–Reviews on Biomembranes, 559, 399–420.10.1016/0304-4157(79)90012-1
- Elgsaeter, A., Stokke, B. T., Mikkelsen, A., & Branton, D. (1986). The molecular basis of erythrocyte shape. Science, 234, 1217–1223.10.1126/science.3775380
- Elston, T., Wang, H., & Oster, G. (1998). Energy transduction in ATP synthase. Nature, 391, 510–513.
- Ford, M. G. J., Mills, I. G., Peter, B. J., Vallis, Y., Praefcke, G. J. K., Evans, P. R., & McMahon, H. T. (2002). Curvature of clathrin-coated pits driven by epsin. Nature, 419, 361–366.10.1038/nature01020
- Frey, T. G., & Mannella, C. A. (2000). The internal structure of mitochondria. TREnds in Biochemical Sciences, 25, 319–324.10.1016/S0968-0004(00)01609-1
- Hyvonen, M., Macias, M. J., Nilges, M., Oschkinat, H., Saraste, M., & Wilmanns, M. (1995). Structure of the binding site for inositol phosphates in a pH domain. EMBO Journal, 14, 4676–4685.
- Israelachvili, J. N., Mitchell, D. J., & Ninham, B. W. (1976). Theory of self-assembly of hydrocarbon amphiphiles into micelles and bilayers. Journal of the Chemical Society, Faraday Transactions II, 72, 1525.10.1039/f29767201525
- Itoh, T., Kigawa, T., Kikuchi, A., Yokoyama, S., & Takenawa, T. (2001). Role of the ENTH domain in phosphatidylinositol-4,5-bisphosphate binding and endocytosis. Science, 291, 1047–1051.10.1126/science.291.5506.1047
- Johnson, J. E., & Cornell, R. B. (1999). Amphitropic proteins: Regulation by reversible membrane interactions (Review). Molecular Membrane Biology, 16, 217–235.10.1080/096876899294544
- Kim, J., Mosior, M., Chung, L. A., Wu, H., & McLaughlin, S. (1991). Binding of peptides with basic residues to membranes containing acidic phospholipids. Biophysical Journal, 60, 135–148.10.1016/S0006-3495(91)82037-9
- Kobayashi, S., Uyama, H., Yamamoto, I., & Matsumoto, Y. (1990). Preparation of monodispersed poly(methyl methacrylate) particle in the size of micron range. Polymer Journal, 22, 759–761.10.1295/polymj.22.759
- Miyazawa, T., Endo, T., Shiihashi, S., & Okawara, M. (1985). Selective oxidation of alcohols by oxoaminium salts (R2N:O+ X−). The Journal of Organic Chemistry, 50, 1332–1334.10.1021/jo00208a047
- Nishi, T., & Forgac, M. (2002). The vacuolar (H+)-atpases—Nature's most versatile proton pumps. Nature Reviews Molecular Cell Biology, 3, 94–103. 10.1038/nrm729
- Oancea, E., & Meyer, T. (1998). Protein kinase C as a molecular machine for decoding calcium and diacylglycerol signals. Cell, 95, 307–318.10.1016/S0092-8674(00)81763-8
- Popot, J. L., & Engelman, D. M. (2000). Helical membrane protein folding, stability, and evolution. Annual Review of Biochemistry, 69, 881–922.10.1146/annurev.biochem.69.1.881
- Rizo, J., & Sudhof, T. C. (1998). C2-domains, structure and function of a universal Ca2+-binding domain. Journal of Biological Chemistry, 273, 15879–15882.10.1074/jbc.273.26.15879
- Sadava, D. E. (1993). Cell biology, organelle structure and function (p. 130). Boston, MA: Jones and Bartlett.
- Yoshida, E. (2009). Photo-living radical polymerization of methyl methacrylate by 2,2,6,6-tetramethylpiperidine-1-oxyl in the presence of a photo-acid generator. Colloid and Polymer Science, 287, 767–772.10.1007/s00396-009-2023-2
- Yoshida, E. (2012). Effects of Illuminance and heat rays on photocontrolld/living radical polymerization mediated by 4methoxy2,2,6,6tetramethylpiperidine1oxyl. ISRN Polymer Science (Article ID: 102186, 6 p.). doi:10.5402/2012/102186
- Yoshida, E. (2013). Giant vesicles prepared by nitroxide-mediated photo-controlled/living radical polymerization-induced self-assembly. Colloid and Polymer Science, 291, 2733–2739.10.1007/s00396-013-3056-0
- Yoshida, E. (2014a). Fission of giant vesicles accompanied by hydrophobic chain growth through polymerization-induced self-assembly. Colloid and Polymer Science, 292, 1463–1468.10.1007/s00396-014-3216-x
- Yoshida, E. (2014b). Morphology control of giant vesicles by manipulating hydrophobic-hydrophilic balance of amphiphilic random block copolymers through polymerization-induced self-assembly. Colloid and Polymer Science, 292, 763–769.10.1007/s00396-013-3154-z
- Yoshida, E. (2014c). Hydrophobic energy estimation for giant vesicle formation by amphiphilic poly(methacrylic acid)-block-poly(alkyl methacrylate-random-mathacrylic acid) random block copolymers. Colloid and Polymer Science, 292, 2555–2561.10.1007/s00396-014-3297-6
- Yoshida, E. (2015a). Morphological changes in polymer giant vesicles by intercalation of a segment copolymer as a sterol model in plasma membrane. Colloid and Polymer Science, 293, 1835–1840.10.1007/s00396-015-3577-9
- Yoshida, E. (2015b). pH response behavior of giant vesicles comprised of amphiphilic poly(methacrylic acid)-block-poly(methyl methacrylate-random-mathacrylic acid). Colloid and Polymer Science, 293, 649–653.10.1007/s00396-014-3482-7
- Yoshida, E. (2015c). Enhanced permeability of rhodamine B into bilayers comprised of amphiphilic random block copolymers by incorporation of ionic segments in the hydrophobic chains. Colloid and Polymer Science, 293, 2437–2443.10.1007/s00396-015-3679-4
- Yoshida, E. (2015d). Morphology control of giant vesicles by composition of mixed amphiphilic random block copolymers of poly(methacrylic acid)-block-poly(methyl methacrylate-random-methacrylic acid). Colloid and Polymer Science, 293, 249–256.10.1007/s00396-014-3403-9
- Yoshida, E. (2015e). Giant vesicles comprised of mixed amphiphilic poly(methacrylic acid)-block-poly(methyl methacrylate-random-methacrylic acid) diblock copolymers. Colloid and Polymer Science, 293, 3641–3648.10.1007/s00396-015-3763-9
- Yoshida, E. (2015f). Morphology transformation of micrometre-sized giant vesicles based on physical conditions for photopolymerisation-induced self-assembly. Supramolecular Chemistry, 27, 274–280.10.1080/10610278.2014.959014
- Yoshida, E. (2015g). Fabrication of microvillus-like structure by photopolymerization-induced self-assembly of an amphiphilic random block copolymer. Colloid and Polymer Science, 293, 1841–1845.doi:10.1007/s00396-015-3600-1