Abstract
The current work is focused on the preparation and characterization of a new group of polysulfone-based organoclay nanocomposite as a promising breast anticancer agent. Polysulfone/organoclay nanocomposites which had abbreviations of PSFH1–3 and PSFB1–3 were prepared using a solution casting technique at room temperature using chloroform as a solvent. PSFH1–3 and PSFB1–3 referred to polysulfone-based halloysite and bentonite in their unmodified and modified forms. Chemical modification of both types was carried out using tetraethylammonium chloride and hexadecyltributylphosphonium bromide, respectively. The final products were produced with different compositions based on the variable loading of modified organoclay. The impacts of the modified fillers on the morphological, thermal, and mechanical properties of the nanocomposites were investigated. A pure polysulfone (PSF) membrane was prepared as a reference. The prepared PSF/organoclay nanocomposite membranes (PSFH1–3, PSFB1–3) were characterized with the aid of FT-IR spectroscopy, SEM, X-ray diffraction, and TGA techniques. The results demonstrated that the impact of the organoclay on the main features of the polysulfone was remarkable and that the incorporation of organic cations alters the thermal stability of polysulfone. X-ray diffraction patterns revealed the formation of intercalated clay mineral layers in the PSF matrix but PSFH3 and PSFB2 have an exfoliated structure. A good dispersion of the organoclay mineral particles was detected by SEM images. The chemical structure of the surfactant affected the thermal behavior of organoclays. Moreover, in the tensile tests, the addition of clay caused a decrease in both the tensile strain at the break and the modulus of elasticity in tension for the nanocomposite membranes. The biological screening showed positive effects for PSFB1 and PSFB2, but PSFH3 and PSFB3 have more promising anticancer effects.
The present work is dealing with the preparation and characterization of polysulfone-based organoclay nanocomposite materials via solution casting technique as promising breast anticancer agents. Different compositions of polysulfone and clay are utilized and the impact of such nanofillers on the morphological, thermal as well as mechanical properties has been investigated in detail. The desired products show good antibacterial activities against both Gram-negative as well as Gram-positive types. Moreover, it shows promising results as a breast anticancer agent through in vitro cytotoxic activity against one human cell line.
1. Introduction
Membrane technology is presently overrun in various industrial fields, such as food, chemical, automotive, electronic, and other important industrial processes (Citation1–3). Fouling issues are an obstacle associated with membrane infiltration used in wastewater treatment. This weakness can lead to problems in separation process efficiency and to frequent maintenance of membrane operations (Citation4). Much research has been performed to overcome this problem. Several methods have been developed to modify membranes to improve membrane performance, including the development of composite membranes via interfacial polymerization (Citation5), UV-initiated grafting (Citation6), plasma treatment (Citation7), and the incorporation of nanoparticles or antifouling agents (Citation8,9). In recent years, research has aimed to produce polymer nanocomposite membranes with enhanced performance for different applications. The use of clay in polymer clay nanocomposite (PCN) technology can be considered an important technique because adding very low clay contents (<10 mass% clay) to the polymers enhances several properties, such as the mechanical, thermal, optical, electric, flammability, and barrier properties (Citation3,10,11). Halloysites and bentonites are widely used in many industrial applications. Their natural abundance, low-cost, high cation exchange capacity (CEC), swelling behaviors, adsorption properties, and large surface area are the driving force behind their widespread use (Citation12). Halloysite has the same theoretical chemical composition as kaolinite but has a higher water content. The unit formula for halloysite can be expressed as Al2Si2O5(OH)4·nH2O (Citation13). Smectite clays like montmorillonite (the main constituent of the bentonite) have the chemical formula of bentonite as (Na,Ca)0.33(Al,Mg)2(Si4O10)(OH)2−·nH2O (Citation14).
These hydrophilic clays need to be modified prior to their introduction into most organophilic (hydrophobic) polymer matrices by ion exchange processing. The interlayer accessible compensating cations can be exchanged with a wide variety of hydrated inorganic or organic cations including those of amines or quaternary ammonium salts such as oxonium, sulfonium, and phosphonium ions (Citation15).
Polysulfone (PSF) is one of the most important membrane materials due to its good mechanical, thermal, and chemical stabilities, good oxidation resistance, and durable mechanical strength (Citation16). Several studies have reported that polysulfone nanocomposites increased modulus, tensile strength, and thermal stability and decreased permeability (Citation10,17). However, traditional PSF membranes are very easily attacked by bacteria and cause membrane biofouling. Biofouling results in a quick decrease in membrane flux, so many published works have focused on polysulfone nanocomposite membranes (Citation18,19). The most common methods used in PCN technology are in situ polymerization, melt intercalation, and solution dispersion (Citation20). In the latter method, the clay mineral is exfoliated in single layers in a solvent medium and polymer chains, which are solubilized in this solvent, and are intercalated into these clay mineral layers. The clay mineral platelets are joined by weak Van der Waals forces and can easily be dispersed in the solvent due to the increase in entropy caused by their disorganization. The polymer is then adsorbed onto the delaminated clay mineral layers and, when the solvent is evaporated, the layers are reassembled and filled with polymer chains, forming an intercalated nanocomposite (Citation21–23). Polymer clay nanocomposite properties depend on the nature of their components (-layer silicate, organic cation, and polymer matrix), morphology, and the preparation method. Furthermore, surfactant coverage on the clay’s surface is also an important factor for controlling the morphology of the nanocomposies (Citation10,17,21,24,25).
Polymeric nanoparticles have many advantages, including low toxicity and high stability. Several drugs formulated in polymeric micelles have been used in clinical trial development for the treatment of various cancers. For example, a chitosan and silver nanocomposite were studied with the A549 lung cancer cell line. A significant IC50 value of 29.35 μg ml−1 was obtained. The synergistic effects of chitosan and silver as a composite at a nanometric size exhibited a maximum of 95.56% inhibition at 100 μg ml−1, resulting in potent anticancer activity (Citation26). Another example incorporating chitin nanoparticles with a metallic nanostructure was highly active toward cancerous cells (Citation27). The application of polymer nanocomposites could be used for the development of novel anticancer therapeutics and may provide a potential platform for cancer management. The aim of this work was to prepare the PSF clay nanocomposite membranes with different types of nanoclay. The types of nanoclay, halloysites and bentonites, were modified by alkyl ammonium or phosphonium salt to decrease the hydrophilicity of the mineral clay. Various characteristics of the prepared nanocomposite membranes were studied using FTIR, XRD, TEM, and TGA techniques to investigate the effect of the clay’s surface coverage on the morphology and mechanical properties of these nanocomposites.
2. Experimental
2.1. Materials
Polysulfone pellets were supplied by ACROS ORGANICS CO; the M.W. was 60,000 g/mol. Chloroform served as a polymer solvent. Two types of nanoclay (halloysite and bentonite) were modified by tetraethylammonium chloride and hexadecyltributylphosphonium bromide salts and all of the materials required for the modification were obtained from the Sigma–Aldrich Company. All chemicals were used as received without any further purification.
2.2. Chemical modifications of the clay
The two types of nanoclay were modified by ion exchange reactions between the nanoclay and two different surfactants, namely, tetraethylammonium chloride and hexdecyltributylphosphonium bromide. A salt solution was heated at 50–80°C for one hour. A hot aqueous suspension of the clay (5 g + 400 ml) was added to the salt solution, and the mixture was stirred for 4 h at 70°C. The cation-exchanged silicates were collected by filtration and subsequently washed with a mixture of hot ethanol and distilled water until a silver nitrate (1 M) test indicated the absence of halide anions. The filter cake was dried at room temperature, ground, and further dried at 70–80°C under vacuum for at least 24 h (Citation28–30).
2.3. Synthesis of polysulfone/organoclay nanocomposites
The desired nanocomposite membranes were prepared using a solution dispersion technique in the presence of chloroform as a solvent. Polysulfone was dissolved in a suitable amount of chloroform (25 ml) under constant stirring at room temperature. Then, 5 wt% of an individual organoclay was also dissolved in a small amount of chloroform (5 ml). This solution was sonicated in a water bath until a clear homogenous suspension was obtained. The desired PSF/organoclay composites were obtained by mixing both solutions and stirring the organoclay solution in PSF solution for 15 min. The resulting solution was poured into a glass blade (mold); evaporation of the CH3Cl yielded homogenous films (25 μm thick) (Citation17,24,31,32). Components of our prepared polysulfone/organoclay nanocomposite membranes are illustrated in Table .
Table 1. Components of pure PSF, PSFH1–3, and PSFB1–3
2.4. Antimicrobial evaluation
The antimicrobial activity of the membranes was tested against four standard bacteria strains and one fungus that were obtained from the microbiology laboratory at the King Abdulaziz University Hospital, Jeddah, KSA. These strains included the Gram-positive bacteria Staphylococcus aureus ATCC 29213 and Bacillus subtilis ATCC 6633, the Gram-negative bacteria Escherichia coli ATCC 35218 and Pseudomonas aeruginosa ATCC 27853, and the fungus Candida albicans ATCC 76615. Preliminary screening of the antibacterial and antifungal activities was conducted using an agar diffusion technique as described previously (Citation33). Briefly, Petri dishes (90 mm) were filled with 25-ml Muller-Hinton agar (Oxoid, UK). The 200 μL bacterial cultures (1 × 105 CFU/ml) adjusted with 0.5 McFarland standard Vitek colourimeter were pipetted onto agar plates and then spread using an aseptically sterile swab. The strains were inoculated separately. Membranes with 7.0 mm in diameter were placed on agar plates after being autoclaved for 30 min and DMF, ceftizoxime (30 μg), amoxicillin/clavulanic acid (20/10 μg), and nystatin (10 μg) discs were used as negative and positive controls. The dishes were incubated for 48 h at 37°C. Inhibitory activity was defined as the absence of bacterial growth in the area surrounding the holes. The inhibition zone was measured using a caliper.
2.5. In vitro cytotoxic screening
The samples were tested for their in vitro cytotoxic activity against a human breast cancer cell line (MCF7 ATCC ® HTB-22™) that was purchased from King Fahd Research Center, Jeddah, KSA.
2.5.1. Cells
Stock cultures were grown in T-75 flasks containing 10 ml of RPMI-1640 medium supplemented with glutamine, bicarbonate, and 10% fetal bovine serum (Gibco, USA). The medium was changed every two days and cells were detached using a solution of 0.25% trypsin-EDTA (Gibco, USA) and then plated in 96-well sterile microtiter plates containing 100 μL RPMI per well at densities of 30,000–100,000 cells per well. Each sample was routinely tested at serial twofold dilutions starting at the upper limit of 100 mg/ml. DMF and doxorubicin (IC50 = 0.41 μM) were used as negative and positive controls, respectively. The culture was incubated for 48 h at 37°C in the presence of 5% CO2. The percentage growth inhibition (IC50) was calculated with the following equation: (OD control wells—OD treated wells)/(OD control wells).
2.5.2. Cell fixation
The cultures were washed with phosphate buffered saline prior to fixation to remove serum proteins that commonly cause cell detachment and loss. The culture was then fixed with cold 50% trichloroacetic acid (TCA) (BDH Chemical Ltd., England) and maintained at 4°C for 1 h. Following fixation, the cells were stained with Sulforhodamine B stain.
2.5.3. Dyes
Sulforhodamine B (SRB 0.4% in 1% acetic acid, w/v) (Sigma–Aldrich, USA) was used in this study, as mentioned previously by Skehan et al. (Citation34), for 30 min. After staining, the cells were washed three times with 1% acetic acid solution to remove unbound dye and then air-dried. Bound dye was solubilized with 10 mM Tris buffer (pH 10.5) for 15 min and the optical density (OD) of both treated and untreated cells was read on an automated spectrophotometric plate reader (ELx808, BioTek, Fisher Scientific, USA) at a wavelength of 490 nm.
2.6. Instrumentation
The nanocomposite structure was also studied by Fourier transform infrared spectroscopy (FT-IR) in the region from 4,000 to 400 cm−1. FT-IR spectroscopic analysis was performed using a JASCO model FT-IR 310 spectrophotometer. Wide-angle X-ray diffraction (WAX-RD) patterns of the nanocomposite membranes were obtained using a Rigaku X-ray diffractometer under the following conditions: 40 kV–30 mA; CuKα radiation (λ = 0.154 nm); and at a rate of 0.6°/min in the range from 1.5 to 25° (2θ). To ensure that the polymer chains and no water molecules were intercalated between the clay mineral platelets, the membranes were calcinated in a reducing atmosphere up to 300°C with a heating rate of 5°C/min, maintaining the temperature at 300°C for 5 min before cooling to room temperature. Subsequently, calcinated membrane SAXRD patterns were also obtained. The thermal properties were investigated using a Shimadzu Thermal Analyzer. Polymer samples of approximately 10 mg were heated from 0 to 800°C with a scan rate of 10.0°C/min in an air atmosphere. Scanning electron microscopy (SEM) images were collected using a Quanta 600 FEG with a 20 kV voltage. The surfaces were sputter-coated with gold with a Bal-tec SCD 050 metallizer to make the surface conductive. According to an ASTM D882-02 standard, an Instron Model 5567 tensile strength measuring machine was used at room temperature with a crosshead speed of 50 mm min−1 to determine the tensile strength of the samples.
3. Results and discussion
The present work focuses on the fabrication and characterization of polysulfone-based organoclay nanocomposite materials (PSFH1–3, PSFB1–3) via a solution casting technique as promising breast anticancer agents. Prior to fabrication, organoclays (halloysite and bentonite) were chemically modified by treatment with surfactant. Both types were subject to chemical modification by ammonium and phosphonium surfactant. Different compositions of polysulfone and organoclay were used to investigate the role of these nanofillers on the morphological, thermal, and mechanical properties. The desired products showed good antibacterial activities against both Gram-negative and Gram-positive bacteria. Moreover, the nanocomposites showed promising results as breast anticancer agents due to their in vitro cytotoxic activity against one human cell line.
3.1. Nanocomposite membrane characterization
Various characterization methods were used to identify and characterize pure PSF, PSFH1–3, andPSFB1–3, including FT-IR, XRD, SEM, thermal analysis, and mechanical properties.
FT-IR was used to examine the extent of the interaction between clay and the polymer matrix. The common features of organoclays were clearly observed from the FT-IR spectra Figures and . The FT-IR results showed the appearance of characteristic absorption bands at 3,600 and 1,039 cm−1 due to the –OH stretching of water and Si–O stretching, respectively, and Al–OH at 918 cm−1 (Citation35,36). Furthermore, the characteristic vibration bands of pure PSF were confirmed and assigned at 1,157 cm−1 (symmetric sulfone stretching), 1,240 cm−1 (aromatic ether), 1,323 cm−1 (asymmetric sulfone linkage), 3,000 ≈ 3,100 cm−1 (aromatic CH stretching), and 2,900 cm−1 (aliphatic CH3 stretching) (Citation31). All the peaks observed for PSF nanocomposites remained the same as those for pure PSF. These results also showed that there were no major chemical or structural changes in the PSF nanocomposites due to the presence of organoclay (Citation37). In all of the nanocomposite membranes, the band related to isopropylidene unit methyl group C–H stretching diminished, suggesting that this part of the PSF molecule displayed restricted movement in the nanocomposite structures due to the clay contents, which restricted PSF chain vibration (Citation38). All the bands had high intensities; however, PSFH3 had low intensities, indicating the good interfacial adhesion between PSF and the organoclay. These results revealed that the phosphonium surfactant’s long alkyl chains were more easily and deeply diffused into the halloysite interlayer than with the bentonites which contained obstructive water molecules (Citation35,39). Moreover, no regular behavior was present in relation to the intensities of the PSF bands and the type of clay (Citation17).
XRD was utilized to determine the dispersion of the clay mineral inside the polymer. Figures and show a series of X-ray diffraction patterns for pure PSF and the PSF/clay nanocomposites PSFH1–3 & PSFB1–3, respectively. XRD plots of halloysite show the presence of halloysite (H), kaolinite (K), quartz (Q), and anatase (A). The major phase in bentonite is montmorillonite (M) and other minor phases are kaolinite (K), quartz (Q), and illite (I). Figures and also show a significant shift in the characteristic peaks for halloysite (at 2θ = 12°) and montmorillonite (at 2θ = 6°) upon formation of the composite materials. The peaks shifted to the higher values when mixed with PSF, which confirms that PSF chains are intercalated between the clay layers. More particularly, the halloysite and bentonite clay peaks (2θ = 22°) shifted to lower values when mixed with PSF, which confirms that PSF chains are intercalated between the clay layers. All the other peaks for pure PSF and the PSF/clay nanocomposites appear in the same position (2θ = 18°). Although intercalation was successful with ammonium and phosphonium surfactants, only PSFH2 formed an exfoliation structure, PSFB2 and PSFB3 displayed exfoliation, and PSFH3 displayed intercalation. This was due to the presence of water molecules in the bentonite gallery interlayers, which decreased the cation exchange processing and led to either a lower alkyl phosphonium surfactant packing density or a higher alkyl phosphonium chain conformational disorder within the bentonite layers (Citation14,21,35). These data are in accordance with those found in the FT-IR data.
Figure 5. SEM images for (a) pure polysulfone PSF at magnification (x = 1,000), (b, c, d) PSFH1, PSFH2, and PSFH3 at magnifications (x = 1,000, 5,000) and (e, f, g) PSFB1, PSFB2, and PSFB3 at similar magnifications.
SEM imaging was performed to ascertain the dispersion of the clays in the PSF matrix. Figure (a) shows that the homogenous surface of pure PSF is smooth. The addition of the unmodified clay (PSFH1, and PSFB1) is spread randomly and homogeneously on the whole surface, Figure (b) and (e). Moreover, the clay particles are closer to each other and display poor interfacial adhesion between PSF and platelets due to their incompatibility. Figure (c), (d), (f), and (g) shows that the spaces between the modified clay particles (PSFH2, PSFH3, PSFB2, and PSFB3) are increased due to the presence of alkyl groups. Moreover, the images of (PSFH2, PSFH3, PSFB2, and PSFB3) indicated that the top surfaces of the clay particles are pale due to increase in the hydrophobicity property and the good compatibility between the PSF and organoclay layers (Citation10). Moreover, the clay layers are separated in the continuous polymer matrix by an average distance that depends on modified clay loading.
Thermal analysis was performed to investigate the effects of clay on the thermal stability of the PSF/clay nanocomposite materials. Figure shows the TGA curves for pure PSF and its corresponding nanocomposite membranes. The TGA curves indicate that pure PSF, PSFH1–3, and PSFB1–3 are thermally degraded in three-stage mass loss events between 165 and 760°C. The thermal stability of the nanocomposite membranes is higher than that of pure PSF membrane (Citation17). The first mass loss is observed at approximately 165°C, which can be attributed to the removal of water, leading to a moderate improvement in the nanocomposite thermal stability. A significant increase in the initial degradation temperature is obviously observed. The second decomposition is observed at approximately 470°C, which is due to the extensive weight loss within the range from 470 to 550°C due to polysulfone degradation. After that, there is another range of slow weight loss till the end of the measurements at 760°C. Table shows the nanocomposite degradation, maximum, and final temperatures and weight loss from 10 to 50% for all the samples. The data in Table illustrate a moderate improvement in the thermal stability for all the nanocomposites. For example, at T10, pure PSF has the lowest value (240°C). The order of thermal stability at T10 is as follows: PSF < PSFB3 < PSFB2 ~ PSFH1 < PSFH2 ~ PSFH3 < PSFB1. Therefore, PSFB1 was the highest thermal stability nanocomposite membrane. Even so, PSFB1 also shows the best value at T20, T30, and T40, but its value decreased at T50 and CDTf. PSFB3 had the highest value at CDTf. These behaviors are due to the presence of clay and the nanocomposite morphology that hindered efficient mass transfer. Therefore, the enhancement in the thermal stability could be explained by the barrier properties attributed to the clay mineral layers, which hampered the diffusion of oxygen molecules into the nanocomposites, as well as by the labyrinth effect of these layers dispersed in the PSF matrix, which delayed volatilization (Citation10,17). The nanoclay-filled sample degradation temperatures are significantly shifted to higher temperatures. Additionally, the thermal stability of the modified/clay nanocomposites is better than the unmodified/clay, Figures and . The thermal behavior can be altered due to the low thermal stability of the surfactants (tetraalkyl-ammonium or hexaalkyl-phosphonium), which causes rapid polysulfone degradation, as in the case of PSFB3, or the same reasons cited in the XRD analysis; thus, it is consistent with the XRD and FT-IR results (Citation14).
Table 2. Thermal behavior of pure PSF, PSFH1–3, and PSFB1–3 membranes
The mechanical properties of the organoclay reinforced the PSF nanocomposites (PSFH1–3 - PSFB1–3). The neat PSF matrices were evaluated by tensile studies that included examining the tensile strain at break and the modulus of elasticity in tension. Polymers have a basic elasticity; the addition of nanoparticles made the elastic polymers more brittle due to pinning at the clay interaction sites, as the deforming forces are then transferred to the nanoclay. The tensile strain at break is considered an essential measure of ductility and is related to the clay type. As evident from the test results reported in Table and Figure , pure PSF showed a percentage tensile strain at break of 28.16%, which decreased with the addition of clay. The tensile strain at break of the nanocomposite types (PSFH1–3) was 9.20, 6.88, and 12.02%, respectively. Also, the tensile strain at break of the nanocomposites types (PSFB1–3) showed a large decrease in the tensile strength at break 4.29, 3.19, and 3.45%, respectively. Generally, the presence of clay in nanocomposites makes them more brittle, causing a decrease in the tensile strain at break. In contrast, at 5 wt% clay loading discontinuity in the form of bonding is present because of the poor adherence by the layered silicate to the polymer and the stress transfer at the clay/PSF interface becomes ineffective (Citation10,17). Moreover, comparison of both types of polysulfone nanocomposites showed that PSFH1–3 displayed higher tensile strength at break than PSFB1–3. This decrease in PSFB1–3 nanocomposites could be explained by the presence of obstructive water molecules that weaken the interfacial adhesion between the PSF chains and silicate layers. PSFH3 had the highest value among the nanocomposite membranes. This enhancement is due to the excellent clay matrix interfacial adhesion and its morphology, predominantly intercalated morphology, which was described by the XRD analysis. Pure PSF exhibited an optimum modulus of elasticity in tension of 76.33 MPa, which decreased significantly in the nanocomposite films and is primarily attributed to the presence of silicate layers, which imparted brittleness to the membranes. This phenomenon is probably due to the lack of proper dispersion by the nanocomposite membranes in PSF and the less favorable interaction between the clay and PSF matrix (Citation40).
Table 3. Modulus of elasticity in tension and tensile strain at break of pure PSF, PSFH1–3, and PSFB1–3 nanocomposite membranes
3.2. Antimicrobial properties
The antimicrobial ability of the membranes was tested, and the results are presented in Table . The pure PSF membrane did not exhibit any significant antibacterial effects through many types of research (Citation8,41). Therefore, the pure PSF membrane surface is not capable of killing bacteria and fungi. The PSFB1 and PSFB2 nanocomposite membranes exhibited antibacterial behaviors against both Gram-negative (Pseudomonas aeruginosa) and Gram-positive (Staphylococcus aureus) bacteria, showing zones around the discs. The radial diameters of the inhibiting zones are 10, 8, 9, and 8 mm.
Table 4. Measured inhibition zone in diameter (mm) of pure PSF, PSFH1–3, and PSFB1–3 membranes on some Gram-positive and Gram-negative bacteria and fungus by agar diffusion method
3.3. Anticancer properties
The samples were also tested for their in vitro cytotoxic activity against one human cell line. The IC50 of the PSFH1–3, PSFB1–3 and pure polysulfone membrane samples was 2.9, 2.5, 2, 2.3, 4.5, 2, and 17.2 mg/ml against MCF7 cells, respectively. Figures and demonstrate the effects of PSFH3 and PSFB3 on MCF7 cell viability, which gave the best results compared to the remaining samples. Figure (A), MCF7 cells grown as a control with DMF solvent, shows the characteristic monolayer of carcinoma cells, with standard features, including: increase in nuclear and cytoplasmic pleomorphism and nucleus sizes and cytoplasm ratios, highly irregular shaped cells (tadpole, caudate), irregular nucleus shapes, and hyperchromasia. In contrast, Figure (B), MCF7 cells with a PSFH3 addition, shows a decreased number of breast carcinoma cells and necrotic cells in suspension. Figure (C), MCF7 cells with as PSFB3 addition, shows a decreased number of breast carcinoma cells, cytoplasmic shrinkage, condensed chromatin, and stained fragments of decomposed cancer cells.
Figure 9. Morphological and cytological features of breast cancer cell line (MCF7) (A–C) treated with Dimethyl formamide solution (vehicle control) (A), treated with sample PSFH3 in a concentration of 2 mg/mL (B), and treated with sample PSFB3 in a concentration of 2 mg/ml (C) after 72 h of cell exposure to the investigated samples.
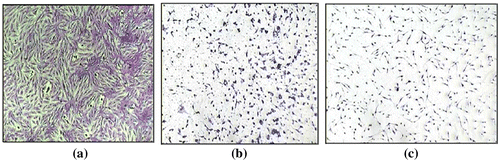
4. Conclusion
PSF/clay nanocomposite membranes have been fabricated using a solution casting technique. Two types of organo/clay are employed as fillers in this study, halloysite and bentonite. The properties of all the products were compared to that of pristine PSF membranes. The FT-IR patterns showed that there were no chemical or structural changes in the PSF/organoclay nanocomposite membranes. The uneven interfacial adhesion between the different membranes enhanced the membranes except for PSFB3. XRD patterns demonstrated that nanocomposite membranes presented both intercalated and exfoliated morphologies. The PSFH3 form showed intercalation in comparison to PSFH1 and PSFH2. The PSFB3 form showed exfoliation in comparison to PSFB1 and PSFB2. SEM images of the surfaces depicted the homogeneity and compatibility between PSF and the organoclay. The thermal stability of the nanocomposite membranes is higher than pure PSF. The order of thermal stability at T10 is as follows: PSF < PSFB3 < PSFB2 ~ PSFH1 < PSFH2 ~ PSFH3 < PSFB1. The tensile strain at break and modulus of elasticity in tension is optimal for pure PSF compared to its nanocomposite membranes. PSFB1 and PSFB2 show good antibacterial against both Gram-negative (P. aeruginosa) and Gram-positive (S. aureus) bacteria. The radial diameters of the inhibition zones were 10, 8, 9, and 8 mm. Thus, both PSFH3 and PSFB3 have promising anticancer activities.
Funding
The authors received no direct funding for this research.
Additional information
Notes on contributors
M.A. Hussein
M.A. Hussein is an associate professor of Polymer Chemistry, Polymer Chemistry Lab, Chemistry Department, Faculty of Science, Assiut University (AU), Egypt. He obtained BSc in Chemistry and PhD in Organic Polymer Synthesis from Assiut University, Egypt. He got a position at Chemistry Department, King Abdulaziz University (KAU), Jeddah, Saudi Arabia in 2010. He got a postdoctoral position in the University of Nice Sophia Antipolis, France and University Sains Malaysia, Malaysia. He visited the school of Industrial technology, University Sains Malaysia and Faculty of Engineering, University of Porto (UP) as visiting researcher two times for each. His research interests are in the area of polymer synthesis, characterization and applications for different fields, polymer composites materials, polymer-doped organic and/or inorganic substances for variable industrial as well as biological interests.
References
- Voicu, S.I.; Stanciu, N.D.; Nechifor, A.C.; Vaireanu, D.-I.; Gheorghe, N. Synthesis and Characterisation of Ionic Conductive Polysulfone Composite Membranes. Romanian J Info. Sci. and Tech. 2009, 12 (3), 410–422.
- Lipnizki, F. Membrane Process Opportunities and Challenges in the Bioethanol Industry. Desalination 2010, 250 (3), 1067–1069.10.1016/j.desal.2009.09.109
- Wang, S.; Hu, Y.; Zong, R.; Tang, Y.; Chen, Z.; Fan, W. Preparation and Characterization of Flame Retardant ABS/Montmorillonite Nanocomposite. Appl. Cla. Sci. 2004, 25 (1–2), 49–55.10.1016/j.clay.2003.08.003
- Van der Bruggen, B.; Mänttäri, M.; Nyström, M. Drawbacks of Applying Nanofiltration and How to Avoid Them: A Review. Sep. and Purifica. Tech. 2008, 63 (2), 251–263.10.1016/j.seppur.2008.05.010
- Mohammad, A.W.; Hilal, N.; Abu Seman, M.N.A. A Study on Producing Composite Nanofiltration Membranes with Optimized Properties. Des. 2003, 158 (1), 73–78.10.1016/S0011-9164(03)00435-1
- Abuhabib, A.A.; Mohammad, A.W.; Hilal, N.; Rahman, R.A.; Shafie, A.H. Nanofiltration Membrane Modification by UV Grafting for Salt Rejection and Fouling Resistance Improvement for Brackish Water Desalination. Des. 2012, 295, 16–25.10.1016/j.desal.2012.03.020
- Kim, E.-S.; Yu, Q.; Deng, B. Plasma Surface Modification of Nanofiltration (NF) Thin-Film Composite (TFC) Membranes to Improve Anti Organic Fouling. Appl. Surf. Sci. 2011, 257 (23), 9863–9871.10.1016/j.apsusc.2011.06.059
- Chung, Y.T.; Mahmoudi, E.; Mohammad, A.W.; Benamor, A.; Johnson, D.; Hilal, N. Development of Polysulfone-Nanohybrid Membranes Using ZnO-GO Composite for Enhanced Antifouling and Antibacterial Control. Des. 2017, 402, 123–132.10.1016/j.desal.2016.09.030
- Ng, L.Y.; Mohammad, A.W.; Leo, C.; Hilal, N. Polymeric Membranes Incorporated with Metal/Metal Oxide Nanoparticles: A Comprehensive Review. Des. 2013, 308, 15–33.10.1016/j.desal.2010.11.033
- Anadão, P.; Sato, L.F.; Wiebeck, H.; Valenzuela-Díaz, F.R. Montmorillonite as a Component of Polysulfone Nanocomposite Membranes. Appl. Cla. Sci. 2010, 48 (1–2), 127–132.10.1016/j.clay.2009.12.011
- Yebra-Rodríguez, A.; Alvarez-Lloret, P.; Cardell, C.; Rodríguez-Navarro, A.B. Crystalline Properties of Injection Molded Polyamide-6 and Polyamide-6/Montmorillonite Nanocomposites. Appl. Cla. Sci. 2009, 43 (1), 91–97.10.1016/j.clay.2008.07.010
- Chen, D.; Zhu, J.X.; Yuan, P.; Yang, S.J.; Chen, T.-H.; He, H.P. Preparation and Characterization of Anion-Cation Surfactants Modified Montmorillonite. J Therm. Anal. and Calor. 2008, 94 (3), 841–848.10.1007/s10973-007-8905-y
- Szczepanik, B.; Słomkiewicz, P.; Garnuszek, M.; Czech, K.; Banaś, D.; Kubala-Kukuś, A.; Stabrawa, I. The Effect of Chemical Modification on the Physico-Chemical Characteristics of Halloysite: FTIR, XRF, and XRD Studies. J Mol. Str. 2015, 1084, 16–22.10.1016/j.molstruc.2014.12.008
- Leite, I.F.; Soares, A.P.S.; Carvalho, L.H.; Raposo, C.M.O.; Malta, O.M.L.; Silva, S.M.L. Characterization of Pristine and Purified Organobentonites. J Therm. Anal. and Calor. 2010, 100 (2), 563–569.10.1007/s10973-009-0265-3
- Salahuddin, N.; Abo-El-Enein, S.A.; Selim, A.; Salah El-Dien, O. Synthesis and Characterization of Polyurethane/Organo-montmorillonite Nanocomposites. Appl. Cla. Sci. 2010, 47 (3–4), 242–248.10.1016/j.clay.2009.10.017
- Yuan, H.G.; Liu, T.Y.; Liu, Y.Y.; Wang, X.L. A Homogeneous Polysulfone Nanofiltration Membrane with Excellent Chlorine Resistance for Removal of Na2SO4 from Brine in Chloralkali Process. Des. 2016, 379, 16–23.10.1016/j.desal.2015.10.006
- Anadão, P.; Montes, R.R.; Larocca, N.M.; Pessan, L.A. Influence of the Clay Content and the Polysulfone Molar Mass on Nanocomposite Membrane Properties. Appl. Surf. Sci. 2013, 275, 110–120.10.1016/j.apsusc.2013.01.102
- Chen, Y.; Zhang, Y.; Liu, J.; Zhang, H.; Wang, K. Preparation and Antibacterial Property of Polyethersulfone Ultrafiltration Hybrid Membrane Containing Halloysite Nanotubes Loaded with Copper Ions. Chem. Eng. J. 2012, 210, 298–308.
- Balta, S.; Sotto, A.; Luis, P.; Benea, L.; Van der Bruggen, B.; Kim, J. A New Outlook on Membrane Enhancement with Nanoparticles: The Alternative of ZnO. J Mem. Sci. 2012, 389, 155–161.10.1016/j.memsci.2011.10.025
- Cong, H.; Radosz, M.; Towler, B.F.; Shen, Y. Polymer–Inorganic Nanocomposite Membranes for Gas Separation. Sep. Pur. Tech. 2007, 55 (3), 281–291.10.1016/j.seppur.2006.12.017
- Sinha Ray, S.; Okamoto, M. Polymer/Layered Silicate Nanocomposites: A Review from Preparation to Processing. Prog. Polym. Sci. 2003, 28 (11), 1539–1641.10.1016/j.progpolymsci.2003.08.002
- Hussain, F.; Hojjati, M.; Okamoto, M.; Gorga, R.E. Review Article: Polymer-matrix Nanocomposites, Processing, Manufacturing, and Application: An Overview. J. Comput. Appl. Math. 2006, 40 (17), 27–32.
- Paul, D.R.; Robeson, L.M. Polymer Nanotechnology. Nanocomposites. Polym. 2008, 49, 3187–3204.
- Monticelli, O.; Bottino, A.; Scandale, I.; Capannelli, G.; Russo, S. Preparation and Properties of Polysulfone–Clay Composite Membranes. J Appl. Polym. Sci. 2007, 103, 3637–3644.10.1002/(ISSN)1097-4628
- Okada, A.; Usuki, A. Twenty Years of Polymer-Clay Nanocomposites. Macromol. Mat. and Eng. 2006, 291 (12), 1449–1476.10.1002/(ISSN)1439-2054
- Arjunan, N.; Kumari, H.L.J.; Singaravelu, C.; Kandasamy, R.; Kandasamy, J. Physicochemical Investigations of Biogenic Chitosan-Silver Nanocomposite as Antimicrobial and Anticancer Agent. Int J Biol Macromol. 2016, 92, 77–87.10.1016/j.ijbiomac.2016.07.003
- Solairaj, D.; Rameshthangam, P.; Arunachalam, G. Anticancer Activity of Silver and Copper Embedded Chitin Nanocomposites Against Human Breast Cancer (MCF-7) Cells. Int J Biol Macromol. 2017, 105, 608–619.10.1016/j.ijbiomac.2017.07.078
- Singla, P. Organic Modification of Clay. Master thesis, School of Chem. and Biochem. Thapan University, 2009, 1–22.
- Ray, S.S. An Overview of Pure and Organically Modified Clays; Elsivier, NY, Clay-containing Polymer Nanocomposites, 2013; pp. 1–24.
- Bhattacharya, S.S.; Aadhar, M. Studies on Preparation and Analysis of Organoclay Nano Particles. Res. J Eng. Sci. 2014, 3 (3), 10–16.
- Voicu, S.I.; Aldea, F.; Radut, M.; Nechifor, G. Nanostructured Polysulfone Composite Membranes. UPB Sci. Bull. Series B 2008, 70 (3), 39–46.
- Sur, G.S.; Sun, H.L.; Lyu, S.G.; Mark, J.E. Synthesis, Structure, Mechanical Properties, and Thermal Stability of Some Polysulfone/Organoclay Nanocomposites. Polym. 2001, 42 (24), 9783–9789.10.1016/S0032-3861(01)00527-4
- Ge, B.; Wang, F.; Sjölund-Karlsson, M.; McDermott, P.F. Antimicrobial Resistance in Campylobacter: Susceptibility Testing Methods and Resistance Trends. J Microbiolog. Meth. 2013, 95 (1), 57–67.10.1016/j.mimet.2013.06.021
- Skehan, P.; Storeng, R.; Scudiero, D.; Monks, A.; McMahon, J.; Vistica, D. New Colorimetric Cytotoxicity Assay for Anticancer-Drug Screening. JNCI: J. National Cancer Inst. 1990, 82 (13), 1107–1112.10.1093/jnci/82.13.1107
- Motawie, A.M.; Madany, M.M.; El-Dakrory, A.Z.; Osman, H.M.; Ismail, E.A.; Badr, M.M. Physico-Chemical Characteristics of Nano-Organo Bentonite Prepared Using Different Organo-Modifiers. Egy. J Petrole. 2014, 23 (3), 331–338.10.1016/j.ejpe.2014.08.009
- Bordeepong, S.; Bhongsuwan, D.; Pungrassami, T.; Bhongsuwan, T. Characterization of Halloysite from Thung Yai District, Nakhon Si Thammarat Province, in Southern Thailand. Songklanakarin J Sci. and Tech. 2011, 33 (5), 599–607.
- Dizman, C.; Ates, S.; Torun, L.; Yagci, Y. Synthesis, Characterization and Photoinduced Curing of Polysulfones with (meth)acrylate Functionalities. Beils. Jof Org. Chem. 2010, 6, 1–7.
- Sharma, B.; Chhibber, R.; Mehta, R. Effect of Surface Treatment of Nanoclay on the Mechanical Properties of Epoxy/Glass Fiber/Clay Nanocomposites. Compos. Interf. 2016, 23 (7), 623–640.10.1080/09276440.2016.1165522
- Ke, Y.C.; Stroeve, P. Polymer-Layered Silicate and Silica Nanocomposites; Elsevier Science, Amsterdam, 2005; pp 211–273.10.1016/B978-044451570-4/50005-7
- Guo, C.; Zhou, L.; Lv, J. Effects of Expandable Graphite and Modified Ammonium Polyphosphate on the Flame-Retardant and Mechanical Properties of Wood Flour-Polypropylene Composites. Polym. and Polym. Compos. 2013, 21 (7), 449–456.
- Xu, Z.; Ye, S.; Zhang, G.; Li, W.; Gao, C.; Shen, C.; Meng, Q. Antimicrobial Polysulfone Blended Ultra Filtration Membranes Prepared with Ag/Cu2O Hybrid Nanowires. J Mem. Sci. 2016, 509, 83–93.10.1016/j.memsci.2016.02.035