ABSTRACT
Prostate cancer (PC) is a commonly diagnosed malignancy in men and is associated with high mortality rates. Current treatments for PC include surgery, chemotherapy, and radiation therapy. However, recent advances in targeted delivery systems have yielded promising new approaches to PC treatment. As PC epithelial cells express high levels of prostate-specific membrane antigen (PSMA) on the cell surface, new drug conjugates focused on PSMA targeting have been developed. microRNAs (miRNAs) are small noncoding RNAs that regulate posttranscriptional gene expression in cells and show excellent possibilities for use in developing new therapeutics for PC. PSMA-targeted therapies based on a miRNA payload and that selectively target PC cells enhances therapeutic efficacy without eliciting damage to normal surrounding tissue. This review discusses the rationale for utilizing miRNAs to target PSMA, revealing their potential in therapeutic approaches to PC treatment. Different delivery systems for miRNAs and challenges to miRNA therapy are also explored.
Introduction
Prostate cancer (PC) is one of the leading causes of mortality in male cancer patients worldwide.Citation1 Effective PC management remains an ongoing challenge, with androgen deprivation therapy (ADT) the current standard PC treatment.Citation2 Although this course of treatment improves the survival and quality of life for individuals with PC, most men eventually progress to metastatic castration-resistant PC (mCRPC).Citation3 Advanced PC (including castration-sensitive and castration-resistant disease) is commonly managed with androgen axis – targeted therapies, such as abiraterone acetate and enzalutamide, docetaxel-based chemotherapy, or radium Ra-223 dichloride.Citation4 Unfortunately, only approximately 50% of patients with advanced disease respond favorably to these therapies, with the other 50% developing resistance and exhibiting survival rates of only 5% − 30%.Citation2 There are many reasons for treatment failure: Factors such as a mutation in the androgen receptor (AR) drug-binding domain, tumor heterogeneity, and vascular permeability all negatively affect efficient drug delivery to tumor sites. Moreover, many currently used drugs exhibit limited specificity and often produce deleterious effects on healthy peripheral tissues.Citation5,Citation6 Therefore, targeted drug delivery holds immense potential to improve cancer treatment by selectively providing effective therapies at tumor sites. Ideally, these therapies not only specifically recognize tumors but also target survival pathways that the tumor has leveraged to achieve drug resistance.
PC cells within prostate tumors express many tumor-associated antigens that can be potentially targeted for cancer diagnosis, treatment, and selective drug delivery.Citation7,Citation8 Prostate-specific membrane antigen (PSMA), a type II transmembrane protein found predominantly on the surface of prostate epithelial cells, is among these,,Citation9–11 PSMA is expressed on the epithelium of nearly all PCs, and its increased expression correlates with progression to castration resistance and metastatic disease.Citation12–14 The cytoplasmic domain of PSMA contains a motif that signals the internalization of PSMA via clathrin-coated pits,Citation15,Citation16 and clinical technologies utilize this pathway to enhance the delivery of radiopharmaceuticals into the tumor, with Citation17Ga-PSMA-11 PET/CT and 177Lu-PSMA-617Citation18–20 leading the way. Studies with both antibody-drug conjugates (ADCs) and small-molecule drug conjugates (SMDCs) have demonstrated encouraging results.Citation21–27 thus highlighting the continued interest in PSMA in biomedical, translational medicine, and pharmaceutical fields.Citation28
microRNAs (miRNAs) are conserved 21–25-nucleotide-long noncoding molecules that play essential roles in regulating gene expression and participate in various biological processes,Citation29 including roles in cancer Citation30,Citation31 by functioning as a tumor suppressor Citation32 or as an onco-miRNA that represses the expression tumor suppressor genes such as p53.Citation32 Each miRNA has the potential to target many genes. By using a single microRNA to silence multiple genes, several signaling pathways can be simultaneously regulated, which may minimize compensatory mechanisms that cause therapeutic resistance. Therefore, manipulating cellular miRNA levels with modified oligonucleotides that mimic or inhibit miRNA function has led to the extensive research and development of miRNAs as therapeutics.Citation33 Loss-of-function approaches have led to superior research results, as they reveal processes dependent on physiological miRNA levels; in contrast, exogenous miRNA added to the system can lead to repressed activity of targeted mRNAs in nonphysiological contexts since miRNA – target interaction is highly concentration dependent. The expression of many miRNAs is tissue-specific and altered in different diseases, including PC. These alterations can significantly affect tumor cell growth and survival.Citation34,Citation35 Furthermore, miRNAs are considered valuable diagnostic biomarkers and potential therapeutic targets in cancer;Citation36 for example, miRNA-15a, miRNA-21, miRNA-34a, miRNA-153, and miRNA-17 have been connected with PC pathogenesis.Citation37,Citation38 Therefore, miRNAs that perturb human disease pathways are potentially powerful candidates for therapeutic intervention against various pathological conditions, including PC.
Although the understanding of miRNA biology has grown exponentially since its discovery in 1993 by Lee and colleagues,Citation39 a more comprehensive assessment of the strengths and limitations of miRNA-based approached for PC therapy is still necessary. In this review, we discuss potential therapeutic strategies of targeting PSMA to deliver specific miRNA payloads exclusively to PC tumors as well as provide insight into various delivery systems for miRNAs and the challenges to using these systems for therapy.
miRNA biogenesis and mechanisms of action
miRNA biogenesis is a complex process that begins with nuclear transcription mediated by RNA polymerase II forming a primary transcript known as primary miRNA (pri-miRNA).Citation40 Pri-miRNAs contain a unique hairpin stem‒loop structure and a single-stranded sequence of differing lengths that can potentially harbor hundreds of kilobases.Citation41 The nuclear complex contains the ribonuclease III enzyme Drosha as well as a double-stranded RNA (dsRNA)-binding protein (DiGeorge syndrome critical region 8 protein (DGCR8)), which facilitate Drosha removal of approximately 11 bp from each side of the hairpin stem of a pri-miRNA, resulting in precursor miRNA (pre-miRNA).Citation41,Citation42 A pre-miRNA is an approximately 70-nucleotide stem‒loop structure that is transported from the nucleus to the cytoplasm by exportin-5 (×PO5), a Ran-GTP-dependent dsRNA-binding protein.Citation43 Once in the cytoplasm, the pre-miRNA is processed by the ribonuclease III enzyme Dicer to form a mature, 22-nucleotide miRNA duplex.Citation44 The mature miRNA is then incorporated into the miRNA-induced silencing complex (RISC),Citation45,Citation46 through which it regulates gene expression through translational repression mediated by mRNA deregulation ().Citation45
Figure 1. miRNA biogenesis and mechanisms of action in posttranscriptional gene regulation. miRNA biogenesis begins with miRNA transcription from DNA via the action of RNA polymerase II to generate primary hairpin miRNA (pri-miRNA). Then, pri-miRNA is cleaved by the RNase III drosha and its binding partner DiGeorge syndrome critical region gene 8 (DGCR8), which recognizes the hairpin structures in pri-miRNA and processes them to form precursor miRNA (pre-miRNA). The resulting pre-miRNA is exported to the cytoplasm by Exportin-5, a Ran-GTP-dependent dsRNA-binding protein. In the cytoplasm, another RNase III enzyme, dicer, further processes pre-miRNA, cleaving its hairpin and thus producing a mature miRNA duplex. Then, one strand is loaded into an argonaute (AGO) family member to form the miRNA-induced silencing complex (RISC) that recognizes the mRNA target via sequence complementarity, resulting in mRNA degradation or translation inhibition.
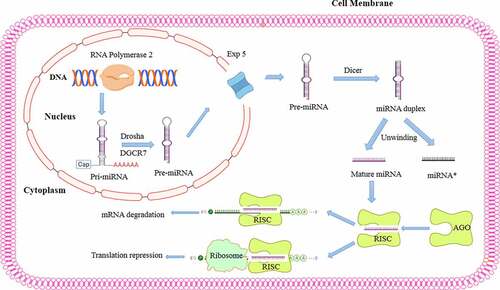
miRnas in PC progression
miRNAs play crucial roles in critical cellular processes such as cell proliferation, differentiation, cell cycle progression, apoptosis, angiogenesis, the epithelial-mesenchymal transition (EMT), and metastasis during cancer progression.Citation47 Hao et al. showed that miRNA-101 inhibited PC cell proliferation by inhibiting cyclooxygenase-2 (COX-2) gene expression, inhibiting the activation of the COX-2/PGE2/EGFR pathway, which mediates cell proliferation during inflammation.Citation48 COX-2 is an inducible isozyme of COX, a key enzyme in converting arachidonic acid to prostaglandins and other eicosanoids. COX-2 is highly expressed in several human cancers and cancer cell lines, including PC tumor cells, and activates the PGE2/EGFR pathway, leading to cell proliferation via extracellular signal-regulated kinase 2 (ERK2) activation.Citation49,Citation50 A study by Zhu et al. demonstrated that miRNA-136 suppressed PC cell proliferation and invasion by targeting mitogen-activated protein kinase 4 (MAP2K4a) in vitro 51. MAP2K4a can increase androgen receptor expression/activation and promote PC tumor progression via noncanonical activation of AKT.Citation51 Moreover, Wang et al. showed that miRNA-182 upregulation increased the expression of important regulators of cell cycle progression, namely c-MYC and cyclin D1, leading to uncontrolled proliferation of the LNCaP and PC3 immortalized human PC cell lines.Citation52 As miRNAs control the expression of cell cycle-related genes, identifying critical miRNAs involved in the cell cycle can lead to better treatment opportunities for cancers, including PC.Citation53 For instance, miRNA-193a functions as a tumor suppressor, and its expression is lower in PC tissues compared to that in benign prostatic hyperplasia.Citation54 In addition, Liu et al. demonstrated that miRNA-193a overexpression inhibited cell growth by targeting cyclin D1 and promoting G1-phase cell cycle arrest in the DU-145 immortalized human PC cell line as well as in PC3 cells.
Apoptosis is a complex process that involves many signaling pathways that can be modulated by miRNAs. Ma et al. showed that miRNA-143 decreases the proliferation and induces the apoptosis of LNCaP cells by suppressing the expression of the integral outer mitochondrial membrane protein BCL2, which inhibits cell death.Citation55 A study by Ostadrahimi et al. demonstrated that miRNA-185, miRNA-30c, and miRNA-1266 were downregulated in PC tissues compared to healthy control tissues,Citation56 resulting in antiapoptotic BCL2 and BCL2-XL gene upregulation and a reduced apoptosis rate.Citation56
Among the crucial outcomes of cancer cell progression to metastatic phenotype acquisition is the EMT.Citation57 Several miRNAs have been suggested to regulate the expression of genes involved in the EMT, and a reduction in their expression leads to cancer invasion and metastasis.Citation58 For example, miRNA-200b targets the zinc-finger E-box-binding homeobox 1 and 2 genes (ZEB1 and ZEB2), Bim1, and E-cadherin. ZEB1/ZEB2 directly bind to the E-box in the promoter of the adhesion molecule E-cadherin, recruiting transcriptional corepressors and inducing the EMT in PC.Citation59–62 miRNA-200b action is crucial for cells to maintain their epithelial phenotype and prevent the EMT and tumor metastasis.Citation63 Yu et al. showed that administration of miRNA-200b downregulated the expression of ZEB1 and ZEB2 in PC3 cells and reversed the EMT, attenuating EMT phenotype acquisition.Citation63 A study by Gandellini et al. demonstrated that miRNA-205 plays a vital role in the EMT by targeting integrin-β4, laminin, and matrix metalloproteinase 2 (MMP2), which are necessary for interactions between the PC cell cytoskeleton and the extracellular matrix.Citation17,Citation64,Citation65 A decrease or loss in miRNA-205 expression results in increased cell proliferation and invasion and changes to prostate cell characteristics, moving them toward a mesenchymal phenotype.Citation66 The essential miRNAs involved in PC pathogenesis are summarized in .
Targeted systems for miRNA delivery
Primary PC results in a highly vascular tumor derived from proliferating prostatic epithelial cells.Citation67 Tumor vascularity significantly affects tumor growth and drug responsiveness because it influences tumor blood flow, oxygenation, and the permeability of chemotherapeutic drugs into the tumor.Citation68,Citation69 Successfully targeted drug delivery systems are small (from 10 to 100 nm in diameter), remain stable in the circulation, accumulate in leaky tumor vasculature via the enhanced permeability and retention (EPR) effect, and enable targeted delivery of specific-ligand-modified drugs and drug carriers to areas with limited access.Citation70,Citation71 Targeting specific cancer cells is a crucial characteristic of drug delivery systems as targeting enhances therapeutic efficacy without eliciting damage to normal surrounding tissue or causing a bystander effect.Citation72 Recent advances in drug delivery systems have suggested promising miRNA-based approaches for the delivery and treatment of different diseases, including PC.Citation73 These advances can be broadly classified into two categories: passive and active targeting approaches.
Passive targeting
Passive targeting exploits the biological characteristics of tumorous and normal tissue to deliver a drug to the target site, where it can exert a therapeutic effect. Tumor growth and metastasis depend on angiogenesis to provide an adequate supply of oxygen and nutrients to the tumor and to remove waste products.Citation74 However, this new tumor vasculature is often defective and leaky, hindering the delivery and effectiveness of systemically administered therapeutic cancer drugs to the tumor.Citation75
Many limitations have prevented miRNAs from becoming optimal candidates for this type of delivery, including the stability of the miRNA in the circulation, its ability to accumulate in pathological sites with differing vascular permeability and nonspecific distribution, and most importantly, the fact that one miRNA has the potential to target many different mRNAs leading to a nonspecific effect.Citation76,Citation77 These challenges to miRNA usage for targeted drug delivery and some possible solutions are highlighted in .
Table 1. Challenges to miRNA usage for targeted drug delivery and possible solutions.
Active targeting
Active targeting affects cancer cells through direct interactions between ligands and target molecules that are overly abundant on the surface of cancer cells, allowing the carriers to distinguish targeted cells from healthy cells.Citation78 The drug carriers are internalized into the cell via receptor-mediated endocytosis, and then, the payload is released.Citation79 This active ligand-specific targeting is particularly suitable for miRNA-mediated drug delivery applications. The most common active targeting carriers for miRNAs are generated from peptides, antibodies, aptamers, and nanoparticles, which help miRNAs specifically target tumor cells.Citation80 In summary, active targeting is a precise mechanism for targeting tumor cells that reduces the need for a high number of miRNAs, which is required for passive targeting, and thus prevents unwanted side effects.Citation81
PSMA is overly abundant on the surface of PC epithelial cells and thus has been used as a successful target for PC management.Citation82 Interestingly, PSMA is expressed on the surface of endothelial cells in the tumor neovasculature in many other types of cancers, including breast, lung, gastric, colorectal, pancreatic and renal cell carcinoma, and bladder cancers. Therefore, using PSMA to carry therapeutic miRNA payloads may be broadly applicable to cancers in addition to PC.Citation83 We discuss the most common active targeting PSMA-based carriers used for miRNA in PC.
PSMA-targeting peptides and proteins as miRNA carriers
Peptide- and protein-based carriers have been broadly used for miRNA delivery because of the ability of the positive charged amino acids to interact with negatively charged nucleotides.Citation84 For instance, Jin et al. developed a novel combinatorial phage biopanning procedure to identify PSMA-specific-targeting peptides as carriers for targeted drug delivery to PC cells.Citation85 They reported that a novel PSMA-specific-targeting peptide named GTI, on the basis of its amino acid sequence, exhibited high binding affinity and selectivity for PSMA and PSMA-positive PC cells. Specifically, GTI mediated the internalization of the apoptotic KLA peptide into PSMA-positive LNCaP cells and induced cell death. Moreover, FAM-labeled GTI displayed high and specific tumor uptake in nude mice bearing human PC xenografts. It can be employed as a PSMA-specific ligand.Citation85 Although this system may be an excellent tool for PC diagnosis and targeted drug delivery to PC, to date, no study on the use of this system for miRNA delivery via PSMA targeting in PC has been reported.
Anti-PSMA antibodies as miRNA carriers
Antibody-based approaches have been widely used to target tumor cells via active targeting with specific drug carriers in cancer.Citation86 Henry et al. used MLN2704, an antibody-chemotherapeutic conjugate consisting of a monoclonal antibody specific to PSMA conjugated to the drug maytansinoid 1 (DM1), which has microtubule-depolymerizing activity. After MLN2704 binds to PSMA through its specific antibody, MLN2704-PSMA is internalized, and DM1 is released into the cells, leading to cancer cell death. The Henry et al. study demonstrated that MLN2704 showed antitumor activity in an animal model of PC, whereas an unconjugated antibody showed no antitumor activity and DM1 alone showed weak tumor-suppressing activity in vivo .Citation87 Rege et al. focused on designing and generating an amphipathic fusion peptide to destroy PC cells. Amphipathic lytic peptides exert cytotoxic effects on PC cells via depolarization of mitochondrial membranes and the induction of apoptosis.Citation88 This group used PSMA-targeted peptides and antibodies against PSMA to precisely deliver cytotoxic amphipathic lytic peptides to PSMA-positive LNCaP cells. The results showed that, compared to the peptides, the antibodies more efficiently targeted the PC cells. Additionally, the group compared the cytotoxic activity of fusion peptides and antibody conjugates and found that treatment with fusion peptides induced oncotic/necrotic death in LNCaP cells; moreover, treatment with the antibody conjugates caused apoptotic death in these cells.Citation88 Several anti-PSMA monoclonal antibodies with cytotoxic agents have been introduced for radioimmunotherapy application to target PSMA-expressing cells.Citation89 For example, Behe et al. used the 177Lu-labeled anti-PSMA monoclonal antibody 3F11 to target PC cells in a mouse xenograft model. Their results indicated that 177Lu-labeled anti-PSMA 3F11 showed high specificity and affinity for a xenograft mouse model, making it a potential candidate for radioimmunotherapeutic applications in PC.Citation90 However, the literature on the use of this system for miRNA delivery in PC treatment is rare.
PSMA-directed aptamers as miRNA carriers
Aptamers are short single-stranded DNA or RNA oligonucleotides with a unique three-dimensional structure that enables its selective binding to specific receptors or protein targets,Citation91 making them excellent drug delivery platforms.Citation92 Conjugation of aptamers to miRNAs is a new method to deliver miRNAs precisely to PC cells.Citation93 Dassie et al. developed an RNA aptamer (A9 g) that selectively inhibited PSMA enzyme activity and functioned as a smart drug for PC treatment. Because PSMA activity plays a crucial role in PC progression, this group showed that PC tumor treatment with the A9 g aptamer in a murine model significantly reduced cell migration and invasion in vitro and metastasis to bone in vivo.Citation94 Wu et al. showed that targeting PC with miRNA-15a and miRNA-16-1 (potent tumor suppressors in PC) through the RNA aptamer A10–3.2, which specifically targets PC cells with PSMA residing on their surface, was beneficial for the selective killing of PC cells in vitro.Citation95 Another study by Ye et al. revealed that aptamers in a compound with hyperbranched polyamidoamine (HPAA) and polyethylene glycol (PEG) and used for targeting PSMA-positive LNCaP cells via miRNA-133a-3p delivery facilitated miRNA-133a-3p delivery into LNCaP cells and showed excellent cytotoxicity in these cells. Furthermore, in an in vivo mouse model of PC, systemic injection of the APT-HPAA-PEG/miRNA-133a-3p compound inhibited tumor growth and prolonged animal survival.Citation96
PSMA nanoparticles as miRNA carriers
Nanoparticles (NPs) are essential carriers in cancer prevention and therapy because they can be generated with unique sizes and shapes that enable them to deliver miRNAs and other chemotherapeutic agents.Citation97 Silica, gold, and iron oxide NPs have been primarily used for miRNA delivery in cancer treatment.Citation98 Luo et al. conjugated a PSMA-targeting ligand named PSMA-1 to gold NPs (AuNPs) and found that these PSMA-1-AuNPs showed greater uptake by PSMA-expressing PC3 cells compared to cells lacking PSMA receptors. As gold can increase radiotherapy sensitization, significantly enhanced radiotherapy efficacy was observed with these PSMA-targeting AuNPs.Citation99 Additionally Binzel et al. reported that NPs containing an anti-PSMA RNA aptamer as the targeting ligand and carrying anti-miRNA-17 or anti-miRNA-21 (two common oncogenes) suppressed miRNA oncogenic activity in PC, showing significant knockdown of miRNA-17 and miRNA-21 and upregulation of phosphatase and tensin homolog (PTEN), a negative regulator of tumor growth in both in vitro and in vivo models of PC.Citation100 Saniee et al. developed a docetaxel-loaded NP consisting of poly(lactic-co-glycolic acid) polyethylene glycol (PLGA-PEG) conjugated with a urea-based anti-PSMA ligand named glutamate-urea-lysine (Glu-urea-Lys) to deliver docetaxel for PC treatment. The uptake of these NPs by PSMA-positive LNCaP and PSMA-negative PC3 cells was analyzed. The results showed that docetaxel uptake was more efficient in the PSMA-positive cells when compared to the control. In addition, this group showed that compared to that of PSMA-targeted NP-carried drugs, the toxicity of untargeted NP-carried drugs was reduced by more than 70%. Finally, the NPs specifically targeting PSMA-positive PC cells showed enhanced the antitumor efficacy mediated via docetaxel.Citation101
Challenges to miRNA therapy
Similar to other treatment strategies, challenges and limitations have been identified in using miRNAs in cancer treatment.Citation102 The first three challenges to miRNA therapy are caused by 1) nucleases quickly degrading naked miRNAs in the circulatory system,Citation103 2) miRNAs quickly cleared through the kidney,Citation102 and 3) naked miRNAs frequently inducing immune responses and being eliminated from the circulation by macrophages, thus requiring high-dose administration, which subsequently leads to toxicity.Citation104,Citation105 To address these three challenges, several different approaches have been employed to alter the miRNA surface charge through structural chemical modifications:Citation106 1) locked nucleic acid (LNA) modification, 2) ribose 2′-OH group modification, 3) peptide nucleic acid (PNA) modification, and 4) backbone modification.Citation106
LNA antisense oligonucleotides have been the most extensively studied miRNA structural modifications and have been demonstrated to enhance endonuclease resistance and increase biodistribution and to exhibit a lower toxicity profile than unmodified miRNA.Citation107 The most common groups used to as a substitution for 2′-OH are 2′-O-methyl, 2′-O-methoxyethyl, and 2′-O-fluoro groups. Substitution with these chemical groups enhanced stability, increased binding affinity, and increased the effectiveness of miRNA inhibition in vivo.Citation108 PNAs are uncharged oligonucleotide analogs. A phosphodiester backbone replacement with PNAs produces N-(2-aminoethyl)-glycine units. PNA recognizes single-stranded nucleic acids with extremely high affinity and sequence selectivity. Although uncharged, PNAs increase oligonucleotide stability, making them suitable for therapeutic approaches.Citation109 Backbone modification is another strategy in which one of the critical atoms in an oligonucleotide is replaced to create a more stable oligonucleotide with therapeutic applications. The most widely used backbone-modified oligonucleotides are generated by replacing one oxygen atom with a sulfur atom. This modification has been shown to enhance nuclease resistance. However, modified oligonucleotides exhibit a short circulation half-life and low binding affinity.Citation110
The fourth challenge in miRNA therapy is low penetration through the cell membrane and the ECM. miRNAs are hydrophilic and therefore cannot cross cell membranes, despite their negative charge.Citation111 Different approaches to help miRNAs cross the cell membrane include active targeting via peptides and conjugation with lipid-soluble compounds such as cholesterol.Citation112 For example, Fabani et al. designed anti-miRNA-122 conjugated to penetratin, a cell-penetrating peptide (also known as a protein transduction domain), enabling delivery of miRNAs through the cell membrane in vitro.Citation113
The fifth challenge in miRNA therapy is endosomal escape and intracellular localization.Citation114 To enhance endosomal release, conjugating peptides and probes have been developed. For example, Xie et al. used chloroquine-containing 2-(dimethylamino)ethyl methacrylate (DMAEMA) copolymers to enhance miRNA delivery by increasing the endosomal escape rate. Their results showed that miRNA delivery efficiency was increased by using chloroquine-DMAEMA copolymers in breast cancer cells.Citation115
The sixth challenge involves miRNA targeting of multiple mRNAs and the subsequent toxicity caused by off-target gene silencing. miRNAs inhibit the expression of target genes by imperfect base pairing with target mRNA, allowing a single miRNA to regulate the expression of multiple genes, acting as a potent multidrug. For example, the tumor-suppressing miRNA miR-34 can downregulate genes involved in cell proliferation (c-MYC, androgen receptor), angiogenesis (VEGF), apoptosis inhibition (BCL2), and the immune response (PD-L1), resulting in a potent antitumor response.Citation116,Citation117 Localized use of miRNAs and active targeting delivery systems can reduce off-target gene silencing and the number of possible side effects.Citation118 Furthermore, miRNAs may face obstacles in reaching their targets due to competitors that interfere with their functions. Increasing evidence suggests that competitive endogenous RNAs (ceRNAs) can prevent the downregulation of mRNA targets by binding through their own miRNA-binding sites.Citation119 These ceRNAs need to be blocked before miRNA targeted therapy can exert the maximum effect. We present a summary of the most critical challenges to miRNA therapy and potential solutions to overcome these challenges through new drug delivery technologies in .
Conclusions and future perspectives
During the past decade, advancements in studies on miRNA functions and their essential roles in cancer have led to many possibilities for miRNA therapeutic applications. To date, many different strategies have been studied to find a suitable method for miRNA delivery in cancer treatment.Citation120 Nevertheless, the specific properties of miRNAs and various physiological obstacles remain the main limitations of in vivo miRNA delivery.Citation121 However, new opportunities and discoveries being presented by targeted miRNA research are increasing the possibility of using miRNA therapy for cancer treatment.Citation122 In the attempts to use miRNA in cancer treatment, PSMA is being tested as a reliable and specific target for developing a targeted delivery system with potential application in PC, one of the most common cancers worldwide.Citation95,Citation96 As targeted miRNA therapy systems mediated through PSMA may increase the efficacy and prevent toxic effects on other human cells, new treatment strategies for using PSMA in PC treatment will be valuable for further studies. Furthermore, future works are needed for designing and optimizing an effective delivery system for miRNAs targeting PSMA and potential combinations of these therapies, along with other therapeutic strategies, for the long-term treatment of PC.
Acknowledgments
The authors acknowledge Kyle Guzy for editing of the manuscript as well as the financial support from NIH NCI 5R21CA256382.
Disclosure statement
No potential conflicts of interest were disclosed.
Data availability statement
The data that support the findings of this study are available from the corresponding author upon reasonable request.
Additional information
Funding
References
- Mohler J, Bahnson RR, Boston B, Busby JE, D’Amico A, Eastham JA, Enke CA, George D, Horwitz EM, Huben RP, et al. Prostate cancer. Journal of the National Comprehensive Cancer Network. 2010;8(2):162–10. doi:10.6004/jnccn.2010.0012.
- Whitburn J, Rao SR, Morris EV, Tabata S, Hirayama A, Soga T, Edwards JR, Kaya Z, Palmer C, Hamdy FC, et al. Metabolic profiling of prostate cancer in skeletal microenvironments identifies G6PD as a key mediator of growth and survival. Sci Adv. 2022;8(8):eabf9096. doi:10.1126/sciadv.abf9096.
- Alibhai SMH, Breunis H, Feng G, Timilshina N, Hansen A, Warde P, Gregg R, Joshua A, Fleshner N, Tomlinson G, et al. Association of chemotherapy, enzalutamide, abiraterone, and radium 223 with cognitive function in older men with metastatic castration-resistant prostate cancer. JAMA Netw Open. 2021;4(7):e2114694. doi:10.1001/jamanetworkopen.2021.14694.
- McAllister MJ, Underwood MA, Leung HY, Edwards J. A review on the interactions between the tumor microenvironment and androgen receptor signaling in prostate cancer. Transl Res. 2019;206:91–106. doi:10.1016/j.trsl.2018.11.004.
- Pal SK, Twardowski P, Sartor O. Critical appraisal of cabazitaxel in the management of advanced prostate cancer. Clin Interv Aging. 2010;5:395–402. doi:10.2147/CIA.S14570.
- Cereda V, Formica V, Massimiani G, Tosetto L, Roselli M. Targeting metastatic castration-resistant prostate cancer: mechanisms of progression and novel early therapeutic approaches. Expert Opin Investig Drugs. 2014;23(4):469–487. doi:10.1517/13543784.2014.885950.
- Erdmann K, Kaulke K, Thomae C, Huebner D, Sergon M, Froehner M, Wirth MP, Fuessel S. Elevated expression of prostate cancer-associated genes is linked to down-regulation of microRnas. BMC Cancer. 2014;14(1):1–14. doi:10.1186/1471-2407-14-82.
- Fuessel S, Sickert D, Meye A, Klenk U, Schmidt U, Schmitz M, Rost A-K, Weigle B, Kiessling A, Wirth MPet al. Multiple tumor marker analyses (PSA, hK2, PSCA, trp-p8) in primary prostate cancers using quantitative RT-PCR. Int J Oncol. 2003;23:221–228. doi:10.3892/ijo.23.1.221.
- Caromile LA, Shapiro LH. PSMA redirects MAPK to PI3K-AKT signaling to promote prostate cancer progression. Molecular & Cellular Oncology. 2017;4:e1321168. doi:10.1080/23723556.2017.1321168.
- Pinto JT, Suffoletto BP, Berzin TM, Qiao CH, Lin S, Tong WP, May F, Mukherjee B, Heston WD. Prostate-specific membrane antigen: a novel folate hydrolase in human prostatic carcinoma cells. Clin Cancer Res. 1996;2:1445–1451.
- Carter RE, Feldman AR, Coyle JT. Prostate-specific membrane antigen is a hydrolase with substrate and pharmacologic characteristics of a neuropeptidase. Proc Natl Acad Sci U S A. 1996;93:749–753. doi:10.1073/pnas.93.2.749.
- Ghosh A, Heston WD. Tumor target prostate specific membrane antigen (PSMA) and its regulation in prostate cancer. J Cell Biochem. 2004;91(3):528–539. doi:10.1002/jcb.10661.
- Murphy GP, Su S, Jarisch J, Kenny GM. Serum levels of PSMA. The Prostate. 2000;42:318–319. doi:10.1002/(SICI)1097-0045(20000301)42:4<318:AID-PROS10>3.0.CO. 2-L [pii].
- Bacich DJ, Pinto JT, Tong WP, Heston WD. Cloning, expression, genomic localization, and enzymatic activities of the mouse homolog of prostate-specific membrane antigen/NAALADase/folate hydrolase. Mamm Genome. 2001;12(2):117–123. doi:10.1007/s003350010240.
- Rajasekaran AK, Anilkumar G, Christiansen JJ. Is prostate-specific membrane antigen a multifunctional protein? Am J Physiol Cell Physiol. 2005;288(5):C975–981. doi:10.1152/ajpcell.00506.2004.
- Liu H, Rajasekaran AK, Moy P, Xia Y, Kim S, Navarro V, Rahmati R, Bander NH. Constitutive and antibody-induced internalization of prostate-specific membrane antigen. Cancer Res. 1998;58:4055–4060.
- Li S, Luo W. Matrix metalloproteinase 2 contributes to aggressive phenotype, epithelial-mesenchymal transition and poor outcome in nasopharyngeal carcinoma. Onco Targets Ther. 2019;12:5701. doi:10.2147/OTT.S202280.
- Chatalic KL, Heskamp S, Konijnenberg M, Molkenboer-Kuenen JDM, Franssen GM, Groningen MCCV, Schottelius M, Wester H-J, van Weerden WM, Boerman OC, et al. Towards personalized treatment of prostate cancer: pSMA I&T, a promising prostate-specific membrane antigen-targeted theranostic agent. Theranostics. 2016;6(6):849–861. doi:10.7150/thno.14744.
- Ganguly T, Dannoon S, Hopkins MR, Murphy S, Cahaya H, Blecha JE, Jivan S, Drake CR, Barinka C, Jones EF, et al. A high-affinity [18F]-labeled phosphoramidate peptidomimetic PSMA-targeted inhibitor for PET imaging of prostate cancer. Nucl Med Biol. 2015;42(10):780–787. doi:10.1016/j.nucmedbio.2015.06.003.
- Haberkorn U, Eder M, Kopka K, Babich JW, Eisenhut M. New strategies in prostate cancer: prostate-specific membrane antigen (psma) ligands for diagnosis and therapy. Clin Cancer Res. 2016;22(1):9–15. doi:10.1158/1078-0432.ccr-15-0820.
- Choy CJ, Ling X, Geruntho JJ, Beyer SK, Latoche JD, Langton-Webster B, Anderson CJ, Berkman CE. 177lu-labeled phosphoramidate-based psma inhibitors: the effect of an albumin binder on biodistribution and therapeutic efficacy in prostate tumor-bearing mice. Theranostics. 2017;7(7):1928–1939. doi:10.7150/thno.18719.
- Ling X, Latoche JD, Choy CJ, Kurland BF, Laymon CM, Wu Y, Salamacha N, Shen D, Geruntho JJ, Rigatti LH, et al. Preclinical dosimetry, imaging, and targeted radionuclide therapy studies of lu-177-labeled albumin-binding, psma-targeted CTT1403. Mol Imaging Biol. 2019;22(2):274–284. doi:10.1007/s11307-019-01404-8.
- Nedrow-Byers JR, Moore AL, Ganguly T, Hopkins MR, Fulton MD, Benny PD, Berkman CE. PSMA-targeted SPECT agents: mode of binding effect on in vitro performance. The Prostate. 2013;73(4):355–362. doi:10.1002/pros.22575.
- Nedrow-Byers JR, Jabbes M, Jewett C, Ganguly T, He H, Liu T, Benny P, Bryan JN, Berkman CE. A phosphoramidate-based prostate-specific membrane antigen-targeted SPECT agent. The Prostate. 2012;72(8):904–912. doi:10.1002/pros.21493.
- Liu T, Wu LY, Kazak M, Berkman CE. Cell-surface labeling and internalization by a fluorescent inhibitor of prostate-specific membrane antigen. The Prostate. 2008;68:955–964. doi:10.1002/pros.20753.
- Choy CJ, Geruntho JJ, Davis AL, Berkman CE. Tunable pH-sensitive linker for controlled release. Bioconjug Chem. 2016;27:824–830. doi:10.1021/acs.bioconjchem.6b00027.
- Choy CJ, Ley CR, Davis AL, Backer BS, Geruntho JJ, Clowers BH, Berkman CE. Second-generation tunable ph-sensitive phosphoramidate-based linkers for controlled release. Bioconjug Chem. 2016;27(9):2206–2213. doi:10.1021/acs.bioconjchem.6b00422.
- Dassie JP, et al. Targeted inhibition of prostate cancer metastases with an RNA aptamer to prostate-specific membrane antigen.
- Romero-Cordoba SL, Salido-Guadarrama I, Rodriguez-Dorantes M, Hidalgo-Miranda A. miRNA biogenesis: biological impact in the development of cancer. Cancer Biology & Therapy. 2014;15:1444–1455. doi:10.4161/15384047.2014.955442.
- Volinia S, Calin GA, Liu C-G, Ambs S, Cimmino A, Petrocca F, Visone R, Iorio M, Roldo C, Ferracin M, et al. A microRNA expression signature of human solid tumors defines cancer gene targets. Proceedings of the National Academy of Sciences. 2006;103(7):2257–2261. doi:10.1073/pnas.0510565103.
- Borralho PM, Simões AES, Gomes SE, Lima RT, Carvalho T, Ferreira DMS, Vasconcelos MH, Castro RE, Rodrigues CMP. miR-143 overexpression impairs growth of human colon carcinoma xenografts in mice with induction of apoptosis and inhibition of proliferation. PloS One. 2011;6(8):e23787. doi:10.1371/journal.pone.0023787.
- Gambari R, Brognara E, Spandidos DA, Fabbri E. Targeting oncomiRnas and mimicking tumor suppressor miRnas: new trends in the development of miRNA therapeutic strategies in oncology. Int J Oncol. 2016;49(1):5–32. doi:10.3892/ijo.2016.3503.
- Garzon R, Marcucci G, Croce CM. Targeting microRnas in cancer: rationale, strategies and challenges. Nat Rev Drug Discov. 2010;9(10):775–789. doi:10.1038/nrd3179.
- Yarahmadi A, Shahrokhi SZ, Mostafavi-Pour Z, Azarpira N. MicroRnas in diabetic nephropathy: from molecular mechanisms to new therapeutic targets of treatment. Biochem Pharmacol. 2021;189:114301. doi:10.1016/j.bcp.2020.114301.
- Wang H, Jiang Y, Peng H, Chen Y, Zhu P, Huang Y. Recent progress in microRNA delivery for cancer therapy by non-viral synthetic vectors. Adv Drug Deliv Rev. 2015;81:142–160. doi:10.1016/j.addr.2014.10.031.
- Bader A, Brown D, Stoudemire J, Lammers P. Developing therapeutic microRnas for cancer. Gene Ther. 2011;18:1121–1126. doi:10.1038/gt.2011.79.
- Sharma N, Baruah MM. The microRNA signatures: aberrantly expressed miRnas in prostate cancer. Clin Transl Oncol. 2019;21:126–144. doi:10.1007/s12094-018-1910-8.
- Chen W-Y, Liu S-Y, Chang Y-S, Juan Yin J, Yeh H-L, Mouhieddine TH, Hadadeh O, Abou-Kheir W, Liu Y-N. MicroRNA-34a regulates WNT/TCF7 signaling and inhibits bone metastasis in ras-activated prostate cancer. Oncotarget. 2015;6(1):441. doi:10.18632/oncotarget.2690.
- Lee RC, Feinbaum RL, Ambros V. The C. elegans heterochronic gene lin-4 encodes small RNAs with antisense complementarity to lin-14. Cell. 1993;75:843–854. doi:10.1016/0092-8674(93)90529-y.
- Lee Y, Kim M, Han J, Yeom K-H, Lee S, Baek SH, Kim VN. MicroRNA genes are transcribed by RNA polymerase II. Embo J. 2004;23(20):4051–4060. doi:10.1038/sj.emboj.7600385.
- Han J, Lee Y, Yeom K-H, Kim Y-K, Jin H, Kim VN. The drosha-DGCR8 complex in primary microRNA processing. Genes & Development. 2004;18(24):3016–3027. doi:10.1101/gad.1262504.
- Guo Z, Li B, Tian P, Li D, Zhang Y, Li Q, Fan T, Yue J, Guo Y. DGCR8 expression is altered in children with congenital heart defects. Clinica Chimica Acta. 2019;495:25–28. doi:10.1016/j.cca.2019.03.1619.
- Zeng Y, Cullen BR. Structural requirements for pre-microRNA binding and nuclear export by exportin 5. Nucleic Acids Res. 2004;32(16):4776–4785. doi:10.1093/nar/gkh824.
- Flores-Jasso CF, Arenas-Huertero C, Reyes JL, Contreras-Cubas C, Covarrubias A, Vaca L. First step in pre-miRnas processing by human dicer. Acta Pharmacol Sin. 2009;30(8):1177–1185. doi:10.1038/aps.2009.108.
- Yoda M, Kawamata T, Paroo Z, Ye X, Iwasaki S, Liu Q, Tomari Y. ATP-dependent human RISC assembly pathways. Nature Structural & Molecular Biology. 2010;17(1):17–23. doi:10.1038/nsmb.1733.
- Meijer HA, Smith EM, Bushell M. Regulation of miRNA strand selection: follow the leader? Biochem Soc Trans. 2014;42:1135–1140. doi:10.1042/BST20140142.
- Cochetti G. Role of miRNAs in prostate cancer: Do we really know everything? Urologic oncology: seminars and original investigations. 2020 Jul;38(7):623–635. doi:10.1016/jurolonc.2020.03.007. PubMed ID: 32284256.
- Hao Y, Gu X, Zhao Y, Greene S, Sha W, Smoot DT, Califano J, Wu T-C, Pang X. Enforced expression of miR-101 inhibits prostate cancer cell growth by modulating the COX-2 pathway in vivo. Cancer Prevention Research. 2011;4(7):1073–1083. doi:10.1158/1940-6207.CAPR-10-0333.
- Zhu Y, Shao S, Pan H, Cheng Z, Rui X. MicroRNA‑136 inhibits prostate cancer cell proliferation and invasion by directly targeting mitogen‑activated protein kinase kinase 4. Mol Med Rep. 2018;17:4803–4810. doi:10.3892/mmr.2018.8417.
- Liu C-Y, Su S-H, Chang T-H, Hsieh M-L, Chang Y-H, Pang JST, Chuang C-K. Promoter gene methylation regulates clooxygenase-2 expression in androgen-dependent and independent prostate cancer cells. World J Oncol. 2022;13(3):107–116. doi:10.14740/wjon1478.
- Shen T, Wang W, Zhou W, Coleman I, Cai Q, Dong B, Ittmann MM, Creighton CJ, Bian Y, Meng Y, et al. MAPK4 promotes prostate cancer by concerted activation of androgen receptor and AKT. J Clin Invest. 2021;131(4). doi:10.1172/JCI135465.
- Ji X, Lu Y, Tian H, Meng X, Wei M, Cho WC. Chemoresistance mechanisms of breast cancer and their countermeasures. Biomedicine & Pharmacotherapy. 2019;114:108800. doi:10.1016/j.biopha.2019.108800.
- Yu Z, Baserga R, Chen L, Wang C, Lisanti MP, Pestell RG. microRNA, cell cycle, and human breast cancer. Am J Pathol. 2010;176(3):1058–1064. doi:10.2353/ajpath.2010.090664.
- Liu Y, Xu X, Xu X, Li S, Liang Z, Hu Z, Wu J, Zhu Y, Jin X, Wang X, et al. MicroRna‑193a‑3p inhibits cell proliferation in prostate cancer by targeting cyclin D1. Oncol Lett. 2017;14:5121–5128. doi:10.3892/ol.2017.6865.
- Ma Z, Luo Y, Qiu M. miR-143 induces the apoptosis of prostate cancer LNCap cells by suppressing Bcl-2 expression. Medical Science Monitor: international Medical Journal of Experimental and Clinical Research. 2017;23:359. doi:10.12659/MSM.899719.
- Ostadrahimi S, Fayaz S, Parvizhamidi M, Abedi‑valugerdi M, Hassan M, Kadivar M, Teimoori‑toolabi L, Asgari M, Shahrokh H, Abolhasani M, et al. Downregulation of miR‑1266‑5P, miR‑185‑5P and miR‑30c‑2 in prostatic cancer tissue and cell lines. Oncol Lett. 2018;15:8157–8164. doi:10.3892/ol.2018.8336.
- Derynck R, Weinberg RA. EMT and cancer: more than meets the eye. Dev Cell. 2019;49:313–316. doi:10.1016/j.devcel.2019.04.026.
- Zaravinos A. The regulatory role of microRnas in EMT and cancer. J Oncol. 2015;2015. doi:10.1155/2015/865816.
- Zhang P, Sun Y, Ma L. ZEB1: at the crossroads of epithelial-mesenchymal transition, metastasis and therapy resistance. Cell Cycle. 2015;14:481–487. doi:10.1080/15384101.2015.1006048.
- Li H. The EMT regulator ZEB2 is a novel dependency of human and murine acute myeloid leukemia. Blood, the Journal of the American Society of Hematology. 2017;129(4):497–508. doi:10.1182/blood-2016-05-714493.
- Fardi M, Alivand M, Baradaran B, Farshdousti Hagh M, Solali S. The crucial role of ZEB2: from development to epithelial‐to‐mesenchymal transition and cancer complexity. J Cell Physiol. 2019;234:14783–14799. doi:10.1002/jcp.28277.
- Sommariva M, Gagliano N. E-cadherin in pancreatic ductal adenocarcinoma: a multifaceted actor during EMT. Cells. 2020;9:1040. doi:10.3390/cells9041040.
- Yu J, LU Y, CUI DI, LI E, ZHU Y, ZHAO Y, ZHAO F, XIA S. miR-200b suppresses cell proliferation, migration and enhances chemosensitivity in prostate cancer by regulating Bmi-1. Oncol Rep. 2014;31(2):910–918. doi:10.3892/or.2013.2897.
- Scott LE, Weinberg SH, Lemmon CA. Mechanochemical signaling of the extracellular matrix in epithelial-mesenchymal transition. Frontiers in Cell and Developmental Biology. 2019;7:135. doi:10.3389/fcell.2019.00135.
- Giannelli G, Bergamini C, Fransvea E, Sgarra C, Antonaci S. Laminin-5 with transforming growth factor-β1 induces epithelial to mesenchymal transition in hepatocellular carcinoma. Gastroenterology. 2005;129:1375–1383. doi:10.1053/j.gastro.2005.09.055.
- Gandellini P, Profumo V, Casamichele A, Fenderico N, Borrelli S, Petrovich G, Santilli G, Callari M, Colecchia M, Pozzi S, et al. miR-205 regulates basement membrane deposition in human prostate: implications for cancer development. Cell Death & Differentiation. 2012;19(11):1750–1760. doi:10.1038/cdd.2012.56.
- Bostwick DG. The pathology of early prostate cancer. CA Cancer J Clin. 1989;39(6):376–393. doi:10.3322/canjclin.39.6.376.
- Hasan J, Byers R, Jayson GC. Intra-tumoural microvessel density in human solid tumours. Br J Cancer. 2002;86(10):1566–1577. doi:10.1038/sj.bjc.6600315.
- Sharma S, Sharma MC, Sarkar C. Morphology of angiogenesis in human cancer: a conceptual overview, histoprognostic perspective and significance of neoangiogenesis. Histopathology. 2005;46:481–489. doi:10.1111/j.1365-2559.2005.02142.x.
- Torchilin V. Tumor delivery of macromolecular drugs based on the EPR effect. Adv Drug Deliv Rev. 2011;63:131–135. doi:10.1016/j.addr.2010.03.011.
- Au J-S, Yeung BZ, Wientjes MG, Lu Z, Wientjes MG. Delivery of cancer therapeutics to extracellular and intracellular targets: determinants, barriers, challenges and opportunities. Adv Drug Deliv Rev. 2016;97:280–301. doi:10.1016/j.addr.2015.12.002.
- Craig V, Tzankov A, Flori M, Schmid CA, Bader AG, Müller A. Systemic microRNA-34a delivery induces apoptosis and abrogates growth of diffuse large B-cell lymphoma in vivo. Leukemia. 2012;26(11):2421–2424. doi:10.1038/leu.2012.110.
- Zang X, Zhang X, Zhao X, Hu H, Qiao M, Deng Y, Chen D. Targeted delivery of miRNA 155 to tumor associated macrophages for tumor immunotherapy. Mol Pharm. 2019;16(4):1714–1722. doi:10.1021/acs.molpharmaceut.9b00065.
- Folkman J. Angiogenesis in cancer, vascular, rheumatoid and other disease. Nat Med. 1995;1(1):27–31. doi:10.1038/nm0195-27.
- Carmeliet P, Jain RK. Angiogenesis in cancer and other diseases. Nature. 2000;407:249–257. doi:10.1038/35025220.
- Zhang L, Liao Y, Tang L. MicroRNA-34 family: a potential tumor suppressor and therapeutic candidate in cancer. Journal of Experimental & Clinical Cancer Research. 2019;38:1–13.
- Jain RK. Barriers to drug delivery in solid tumors. Sci Am. 1994;271(1):58–65. doi:10.1038/scientificamerican0794-58.
- Bertrand N, Wu J, Xu X, Kamaly N, Farokhzad OC. Cancer nanotechnology: the impact of passive and active targeting in the era of modern cancer biology. Adv Drug Deliv Rev. 2014;66:2–25. doi:10.1016/j.addr.2013.11.009.
- Farokhzad OC, Langer R. Impact of nanotechnology on drug delivery. ACS Nano. 2009;3:16–20. doi:10.1021/nn900002m.
- Bae YH. Drug targeting and tumor heterogeneity. J Control Release. 2009;133(1). doi:10.1016/j.jconrel.2008.09.074.
- Hayes J, Peruzzi PP, Lawler S. MicroRnas in cancer: biomarkers, functions and therapy. Trends Mol Med. 2014;20:460–469. doi:10.1016/j.molmed.2014.06.005.
- Caromile LA, Dortche K, Rahman MM, Grant CL, Stoddard C, Ferrer FA, Shapiro LH. PSMA redirects cell survival signaling from the MAPK to the PI3K-AKT pathways to promote the progression of prostate cancer. Sci Signal. 2017;10(470). doi:10.1126/scisignal.aag3326.
- Nguyen DP, Xiong PL, Liu H, Pan S, Leconet W, Navarro V, Guo M, Moy J, Kim S, Ramirez-Fort MK, et al. Induction of PSMA and internalization of an anti-PSMA mAb in the vascular compartment. Mol Cancer Res. 2016;14(11):1045–1053. doi:10.1158/1541-7786.MCR-16-0193.
- Singh S, Grossniklaus HE, Kang SJ, Edelhauser HF, Ambati BK, Kompella UB. Intravenous transferrin, RGD peptide and dual-targeted nanoparticles enhance anti-VEGF intraceptor gene delivery to laser-induced CNV. Gene Ther. 2009;16(5):645–659. doi:10.1038/gt.2008.185.
- Jin W, Qin B, Chen Z, Liu H, Barve A, Cheng K. Discovery of PSMA-specific peptide ligands for targeted drug delivery. Int J Pharm. 2016;513(1–2):138–147. doi:10.1016/j.ijpharm.2016.08.048.
- Zhao Z, Ukidve A, Kim J, Mitragotri S. Targeting strategies for tissue-specific drug delivery. Cell. 2020;181:151–167. doi:10.1016/j.cell.2020.02.001.
- Henry MD, Wen S, Silva MD, Chandra S, Milton M, Worland PJ. A prostate-specific membrane antigen-targeted monoclonal antibody–chemotherapeutic conjugate designed for the treatment of prostate cancer. Cancer Res. 2004;64(21):7995–8001. doi:10.1158/0008-5472.CAN-04-1722.
- Rege K, Patel SJ, Megeed Z, Yarmush ML. Amphipathic peptide-based fusion peptides and immunoconjugates for the targeted ablation of prostate cancer cells. Cancer Res. 2007;67:6368–6375. doi:10.1158/0008-5472.CAN-06-3658.
- Tykvart J, Navrátil V, Sedlák F, Corey E, Colombatti M, Fracasso G, Koukolík F, Bařinka C, Šácha P, Konvalinka J. Comparative analysis of monoclonal antibodies against prostate‐specific membrane antigen (PSMA). The Prostate. 2014;74(16):1674–1690. doi:10.1002/pros.22887.
- Behe M, Alt K, Deininger F, Bühler P, Wetterauer U, Weber WA, Elsässer-Beile U, Wolf P. In vivo testing of 177lu-labelled anti-PSMA antibody as a new radioimmunotherapeutic agent against prostate cancer. In Vivo. 2011;25:55–59.
- Keefe AD, Pai S, Ellington A. Aptamers as therapeutics. Nature reviews Drug discovery. 2010;9:537–550. doi:10.1038/nrd3141.
- Kruspe S, Mittelberger F, Szameit K, Hahn U. Aptamers as drug delivery vehicles. ChemMedchem. 2014;9:1998–2011. doi:10.1002/cmdc.201402163.
- Esposito CL, Cerchia L, Catuogno S, De Vita G, Dassie JP, Santamaria G, Swiderski P, Condorelli G, Giangrande PH, de Franciscis V et al. Multifunctional aptamer-miRNA conjugates for targeted cancer therapy. Molecular Therapy. 2014;22(6):1151–1163. doi:10.1038/mt.2014.5.
- Dassie JP, Hernandez LI, Thomas GS, Long ME, Rockey WM, Howell CA, Chen Y, Hernandez FJ, Liu XY, Wilson ME, et al. Targeted inhibition of prostate cancer metastases with an RNA aptamer to prostate-specific membrane antigen. Molecular Therapy. 2014;22(11):1910–1922. doi:10.1038/mt.2014.117.
- Wu X . Second-generation aptamer-conjugated PSMA-targeted delivery system for prostate cancer therapy. Int J Nanomedicine. 2011;6:1747. doi:10.2147/IJN.S23747.
- Ye Y, Zhang L, Dai Y, Wang Z, Li C, Peng Y, Ma D, He P. PSMA-Targeting reduction-cleavable hyperbranched polyamide-amine gene delivery system to treat the bone metastases of prostate cancer. molecular Therapy. 2020;15:7173. doi:10.2147/IJN.S268398.
- Mehlhorn H. 2016. Nanoparticles in the fight against parasites. In: Melhorn H, editor. Parasitology Research MonographsSwitzerland. 1st ed. pp. 1–14. Springer Cham: Nanoparticles - Definitions. doi: 10.1007/978-3-319-25292-6.
- Bhattacharyya S, Kudgus RA, Bhattacharya R, Mukherjee P. Inorganic nanoparticles in cancer therapy. Pharm Res. 2011;28:237–259. doi:10.1007/s11095-010-0318-0.
- Luo D, Wang X, Zeng S, Ramamurthy G, Burda C, Basilion JP. Prostate-specific membrane antigen targeted gold nanoparticles for prostate cancer radiotherapy: does size matter for targeted particles? Chemical Science. 2019;10(35):8119–8128. doi:10.1039/C9SC02290B.
- Binzel DW, Shu Y, Li H, Sun M, Zhang Q, Shu D, Guo B, Guo P. Specific delivery of miRNA for high efficient inhibition of prostate cancer by RNA nanotechnology. Molecular Therapy. 2016;24(7):1267–1277. doi:10.1038/mt.2016.85.
- Saniee F, Shabani Ravari N, Goodarzi N, Amini M, Atyabi F, Saeedian Moghadam E, Dinarvand R. Glutamate-urea-based PSMA-targeted PLGA nanoparticles for prostate cancer delivery of docetaxel. Pharm Dev Technol. 2021;26(4):381–389. doi:10.1080/10837450.2021.1875238.
- Chen Y, Gao D-Y, Huang L. In vivo delivery of miRnas for cancer therapy: challenges and strategies. Adv Drug Deliv Rev. 2015;81:128–141. doi:10.1016/j.addr.2014.05.009.
- Segal M, Slack F. Challenges identifying efficacious miRNA therapeutics for cancer. Expert Opinion on Drug Discovery. 2020;15(9):987–991. doi:10.1080/17460441.2020.1765770.
- Cortez MA, Anfossi S, Ramapriyan R, Menon H, Atalar SC, Aliru M, Welsh J, Calin GA. Role of miRnas in immune responses and immunotherapy in cancer. Genes Chromosomes Cancer. 2019;58(4):244–253. doi:10.1002/gcc.22725.
- Curtale G. MiRnas at the crossroads between innate immunity and cancer: focus on macrophages. Cells. 2018;7(12). doi:10.3390/cells7020012.
- Pereira DM, Rodrigues PM, Borralho PM, Rodrigues CM. Delivering the promise of miRNA cancer therapeutics. Drug Discov Today. 2013;18:282–289. doi:10.1016/j.drudis.2012.10.002.
- Stenvang J, Silahtaroglu AN, Lindow M, Elmen J, Kauppinen S. The utility of LNA in microRNA-based cancer diagnostics and therapeutics. Seminars in cancer biology. 2008;18(2):89–102. doi:10.1016/j.semcancer.2008.01.004.
- Davis S, Lollo B, Freier S, Esau C. Improved targeting of miRNA with antisense oligonucleotides. Nucleic Acids Res. 2006;34:2294–2304. doi:10.1093/nar/gkl183.
- Sharma C, Awasthi SK. Versatility of peptide nucleic acids (PNA s): role in chemical biology, drug discovery, and origins of life. Chemical Biology & Drug Design. 2017;89:16–37. doi:10.1111/cbdd.12833.
- Lima JF, Cerqueira L, Figueiredo C, Oliveira C, Azevedo NF. Anti-miRNA oligonucleotides: a comprehensive guide for design. RNA Biol. 2018;15:338–352. doi:10.1080/15476286.2018.1445959.
- Schachner-Nedherer A-L, Werzer O, Kornmueller K, Prassl R, Zimmer A. Biological activity of miRNA-27a using peptide-based drug delivery systems. Int J Nanomedicine. 2019;14:7795. doi:10.2147/IJN.S208446.
- Zou L-L, Ma J-L, Wang T, Yang T-B, Liu C-B. Cell-penetrating peptide-mediated therapeutic molecule delivery into the central nervous system. Chemical Biology & Drug Design. 2013;11(2):197–208. doi:10.2174/1570159X11311020006.
- Fabani MM, Gait MJ. miR-122 targeting with LNA/2′-O-methyl oligonucleotide mixmers, peptide nucleic acids (PNA), and PNA–peptide conjugates. Rna. 2008;14:336–346. doi:10.1261/rna.844108.
- Orellana EA, Abdelaal AM, Rangasamy L, Tenneti S, Myoung S, Low PS, Kasinski AL. Enhancing microRNA activity through increased endosomal release mediated by nigericin. Molecular Therapy-Nucleic Acids. 2019;16:505–518. doi:10.1016/j.omtn.2019.04.003.
- Xie Y, Yu F, Tang W, Alade BO, Peng Z-H, Wang Y, Li J, Oupický D. Chloroquine-containing DMAEMA copolymers as efficient anti-miRNA delivery vectors with improved endosomal escape and anti-migratory activity in cancer cells. Macromol Biosci. 2018;18(1). doi:10.1002/mabi.201700194.
- Siemens H, Jackstadt R, Hünten S, Kaller M, Menssen A, Götz U, Hermeking H. miR-34 and SNAIL form a double-negative feedback loop to regulate epithelial-mesenchymal transitions. Cell Cycle. 2011;10(24):4256–4271. doi:10.4161/cc.10.24.18552.
- Li WJ, Wang Y, Liu R, Kasinski AL, Shen H, Slack FJ, Tang DG. microRNA-34A: potent tumor suppressor, cancer stem cell inhibitor, and potential anticancer therapeutic. Front Cell Dev Biol. 2021;9:640587. doi:10.3389/fcell.2021.640587.
- Tong A, Nemunaitis J. Modulation of miRNA activity in human cancer: a new paradigm for cancer gene therapy? Cancer Gene Ther. 2008;15:341–355. doi:10.1038/cgt.2008.8.
- Thomson DW, Dinger ME. Endogenous microRNA sponges: evidence and controversy. Nat Rev Genet. 2016;17(5):272–283. doi:10.1038/nrg.2016.20.
- Fernandez-Piñeiro I, Badiola I, Sanchez A. Nanocarriers for microRNA delivery in cancer medicine. Biotechnol Adv. 2017;35:350–360. doi:10.1016/j.biotechadv.2017.03.002.
- Conde J, Artzi N. Are RNAi and miRNA therapeutics truly dead? Trends Biotechnol. 2015;33:141–144. doi:10.1016/j.tibtech.2014.12.005.
- Ganju A, Khan S, Hafeez BB, Behrman SW, Yallapu MM, Chauhan SC, Jaggi M. miRNA nanotherapeutics for cancer. Drug Discov Today. 2017;22(2):424–432. doi:10.1016/j.drudis.2016.10.014.