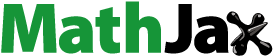
ABSTRACT
Despite more than ten years of extensive research, the superconducting mechanism of iron-based superconductors (FeSCs) is still an open question. Generally, the high-temperature superconductivity is often observed with suppression of magnetic ordering, spin-density-wave, or even the structure transition by carrier doping. Furthermore, an electronic state ordering is also observed at temperatures close to or even above these transitions. Due to its proximity to the superconducting state and disappearance near the optimal superconductivity, it has been also suggested to interplay with superconductivity on a phenomenological level. Nevertheless, there is still no direct evidence to bridge the superconductivity to these transitions. Recently, another nematic order was observed in the superconducting state of heavily hole-doped compound AFeAs
(A = K, Rb, Cs), providing a possibility to explore the superconductivity gap symmetry nature. Here, by reviewing the recent experimental progresses on the nematic superconductivity in the FeSCs, we will introduce the progresses by various methods including the quasi-particle interference from scanning tunneling microscope, anisotropic gap magnitudes from angular resolved photoemission, the upper critical field and the superconducting transition temperatures from transport measurements. In addition, some recent reports and theoretical explanations for experimental results are followed.
Graphical abstract
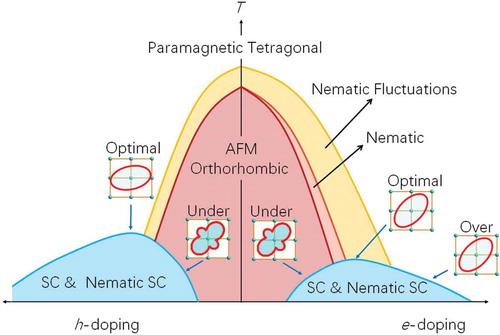
1. Introduction
The phenomena of spontaneous rotational symmetry breaking with invariant translational symmetry, which are termed as electronic ordering, have been discovered in many unconventional superconductors, such as cuprates [Citation1,Citation2], heavy-fermion materials [Citation3,Citation4], and the iron-based superconductors (FeSCs) [Citation5–12]. This anisotropic state is a so-called ‘nematic’ phase, which is borrowed from a liquid-crystal phase to describe the electronic state [Citation13,Citation14]. In these unconventional superconductors, quantum fluctuations with multiple orders including nematicity, antiferromagnetism, structure distortion, pseudo gap, and orbital fluctuation [Citation15–24] emerge in the parent compounds and evolve with doping, often coexist with superconductivity in some region, and are suppressed under the circumstance where the highest superconductivity is observed. Thus, the interplay of these orders with superconductivity has been the central theme in the research and is often considered as a window for the insight into the unconventional superconducting mechanism.
Nevertheless, the pairing glue of Cooper pairs in FeSCs is still under debate [Citation25], and there has been little direct evidence for a link from nematicity to superconductivity yet [Citation14], although the competition of the possible ordering and superconductivity within the superconducting state is essential. In this respect, we will introduce the recent progress of electronic nematicity in the supercondcuting state. As more comprehensive overviews on the interplay between magnetism, nematicity and superconductivity already exist [Citation7,Citation25–28], in this short review we intend to give more attention on recent experimental progress and try to establish the connection between nematic order and nematic behaviours of superconductivity. The rest pages are organized as follows: In section 2, we introduce the nematicity in normal state and fundamental properties of iron-based superconductors, such as the phase diagrams and electronic structures, with the theory of driven forces of nematicity. In section 3, we will show the gap symmetry of the ‘11’- system (FeSe) superconductors and the nematic superconductivity. In section 4, we shed light on the novel nematic superconductivity observed in hole-doped iron pnictides and introduce our latest related progress on the doped ‘122’- system (BaFeAs
) superconductors. Finally in section 5, we briefly summarize the article and give the perspectives.
2. Nematicity in normal state
In FeSCs, a tetragonal-to-orthorhombic structure transition usually occurs upon cooling from room temperature, in advance of the emergence of superconductivity [Citation5–12]. Because of the existence of electricity grain boundary, it is a challenge to probe the nematicity accompanied by the structure distortion with macroscopic techniques such as electronic transport, for which the key prerequisite is to detwin the crystals. Early successful attempt on measuring in-plane anisotropy was achieved by applying uniaxial pressure to detwin the sample [Citation29], as shown in and (b). In , the measurement setup of resistivity along the direction of
- and
-axes in orthorhombic lattice and the mechanical cantilever used to apply compressive stress are exhibited. presents the temperature-dependent in-plane anisotropy of resistivity
and
of Ba(Fe
Co
)
As
samples with different Co concentrations, from which a significant transition (marked with solid lines) is observed.
Figure 1. (a) Diagram of the experimental configuration and device used to detwin single crystals in situ. The order of magnitude estimation of the force on samples can be obtained by estimating the curvature of the cantilever [29]. (b) Temperature dependence of the in-plane resistivity (green) and
(red) of Ba(Fe
Co
)
As
. Solid and dashed vertical lines mark critical temperatures for the structural and magnetic phase transitions T
and T
Citation29. (c) and (d) Phase diagrams of Ba(Fe
Co
)
As
and FeSe
S
with inserts showing the crystal structures of their parent compounds, respectively [Citation30–32]
![Figure 1. (a) Diagram of the experimental configuration and device used to detwin single crystals in situ. The order of magnitude estimation of the force on samples can be obtained by estimating the curvature of the cantilever [29]. (b) Temperature dependence of the in-plane resistivity ρa (green) and ρb (red) of Ba(Fe 1−xCo x) 2As 2. Solid and dashed vertical lines mark critical temperatures for the structural and magnetic phase transitions T s and T NCitation29. (c) and (d) Phase diagrams of Ba(Fe 1−xCo x) 2As 2 and FeSe 1−xS x with inserts showing the crystal structures of their parent compounds, respectively [Citation30–32]](/cms/asset/b785e542-a65b-4328-a389-569db97016a4/tapx_a_1878931_f0001_oc.jpg)
With the information of in-plane resistivity, the phase diagram can be then mapped out. In the phase diagram of Co-doped BaFeAs
Citation31 in , the modulation of Co doping gradually suppresses both static antiferromagnetic order and structure transition with an increasing separation between them. Superconductivity emerges simultaneously and reaches the highest
as both orders are suppressed, suggesting a competition between superconductivity and the orders. However, the phase diagram of FeSe [Citation30] in suggests another prospect of the interactions. In the S-doped FeSe, superconductivity emerges without the presence of magnetic order and shows little dependence with doping and the evolution of nematic order. Such contrasting phenomena between different families of FeSCs suggest the complex interplay between nematicity, magnetism and superconductivity.
While it is widely believed that nematicity plays an important role in the pairing mechanism of superconductivity, the microscopic mechanism of nematic order has not been figured out and remains controversial. Since the resistivity anisotropies are significantly larger than relative lattice distortions and also exhibit a non-trivial dependence on doping, the nematicity is likely to be electronic driven rather than phonon-driven. Considering the multiple-orbital physics of FeSCs, all five Fe orbitals get involved in forming a complex Fermi surface as shown in . A popular theory [Citation14] is based on the multi-orbital Hubbard model, which describes hopping between Fe-As orbitals and local interactions, including intra-band and inter-band Hubbard repulsions and Hund’s exchange.
Figure 2. (a) Calculated orbital assignment band structure of iron pnictide based on the five-band model [Citation33]. (b) Minimal Fermi surface presented with hole pockets (green lines) at the centre () of the 1-Fe lattice Brillouin zone and electron pockets (blue lines) centred at the X = (
, 0) and Y = (0,
) points of the Brillouin zone. V
is the inter-pocket interaction [Citation14]
![Figure 2. (a) Calculated orbital assignment band structure of iron pnictide based on the five-band model [Citation33]. (b) Minimal Fermi surface presented with hole pockets (green lines) at the centre (Γ) of the 1-Fe lattice Brillouin zone and electron pockets (blue lines) centred at the X = (π, 0) and Y = (0,π) points of the Brillouin zone. V inter is the inter-pocket interaction [Citation14]](/cms/asset/77dc6551-b20a-4942-9e80-bda5125f17b8/tapx_a_1878931_f0002_oc.jpg)
Specifically, two scenarios named magnetic and charge/orbital scenario with opposite sign of the effective inter-pocket interaction V, which is a combination of the Hubbard and Hund interactions dressed up by coherence factors, are proposed for the possible mechanisms [Citation15–19]. In the magnetic scenario, the spin fluctuations can split two magnetic orders with ordering vectors along
and
directions, defining the nematic and magnetic order simultaneously. Superconductivity can be induced by the enhancement of inter-pocket repulsion (positive V
), which becomes larger than intra-pocket repulsion. In the charge/orbital scenario, the only difference from the magnetic one is the sign of the interaction V
between electron and hole pockets. If this interaction turns out to be negative, it is the charge/orbital susceptibility rather than the spin susceptibility that is enhanced, and superconductivity develops as the intra-pocket interaction is attractive and enhanced.
Both of the scenarios proposed unique types of superconducting gap structures, which can be verified via experiments. In the magnetic scenario, an unconventional superconductivity, in which the gap functions have different signs in the hole and in the electron pockets, a
superconductivity, or even the mixture of the two types can be developed [Citation39]. While in the charge/orbital scenario, a conventional
state, where the gap functions have the same sign in all pockets, can be realised. Such verdict also triggers the tremendous efforts for defining the superconducting gap structure in the subsequent works, as to be introduced in the following sections.
3. Nematic superconductivity in 11-system
In the past few years, the field of iron-based superconductivity has been largely promoted by studies on the simplest FeSe family of FeSCs. Without the presence of magnetic order at ambient pressure, FeSe has provided an ideal platform to study the nematicity and superconductivity.
While the similar anisotropy of in-plane resistivity has been observed with the efforts to overcome difficulties of detwining the fragile FeSe [Citation9], more impressive facts of the anisotropic superconducting gap have been revealed by microscopic techniques such as scanning tunneling microscopy (STM) with subkelvin Bogoliubov quasiparticle interference (BQPI) and Angle-resolved photoemission spectroscopy (ARPES). Early STM investigations of thin film FeSe observed elongated magnetic vortices indicating the twofold pairing symmetry [Citation34], as shown in . Later calculation constructed on the extended Ginzburg-Landau free energy is found to be consistent with the elliptical vortex [Citation35], where a mixed -wave and
-wave superconducting order is coupled to the nematic order().
Figure 3. (a) Zero-bias conductance map of FeSe for a single vortex at 0.4 K and 1 T [34]. (b) Calculated profiles of superconducting order around vortex core with nematic order [35]
![Figure 3. (a) Zero-bias conductance map of FeSe for a single vortex at 0.4 K and 1 T [34]. (b) Calculated profiles of superconducting order around vortex core with nematic order [35]](/cms/asset/21ed717c-bfc0-4462-8301-483f8da9f2a9/tapx_a_1878931_f0003_oc.jpg)
Investigations by BQPI and ARPES further obtain affluent details of the superconducting gap structures. In the results from BQPI [Citation36], the Fermi surface geometry of the electronic bands around point and X point of FeSe are determined. Both gaps are extremely anisotropic with opposite signs and gap maxima oriented orthogonally in momentum space, as shown in and (b). Orbital-selective Cooper pairing from the
orbitals of the iron atoms is also suggested. On the other hand, by mapping the gap function
on the Fermi surface, ARPES measurements [Citation38,Citation38] similarly find strongly anisotropic gaps with maxima on the flat sides of the elliptic
pocket, with a possible nodal structure in the situation of a single domain. The data are summarized in .
Figure 4. (a) Anisotropic energy gaps of FeSe. The red and blue colors indicate the different signs of the two gap functions. (b) Measured angular dependence of FeSe superconducting energy gaps around
point and
around X point [36]. (c) Superconducting gap anisotropy of the elliptical Fermi surface. Results of the multi-domain sample with
- (red circle) and
- (blue circle) polarized light are shown together. (d) Result of (c) presented in Cartesian coordinates [Citation37]. (e) (Left) Energy distribution curves (EDCs) on the holelike pocket plotted as a grayscale image, and (right) location of the Fermi momentum on the holelike pocket. (f) Momentum dependence of the superconducting gap derived from fitting the symmetrized EDCs [Citation38]
![Figure 4. (a) Anisotropic energy gaps of FeSe. The red and blue colors indicate the different signs of the two gap functions. (b) Measured angular dependence of FeSe superconducting energy gaps Δα(k) around Γ point and Δε(k) around X point [36]. (c) Superconducting gap anisotropy of the elliptical Fermi surface. Results of the multi-domain sample with p- (red circle) and s- (blue circle) polarized light are shown together. (d) Result of (c) presented in Cartesian coordinates [Citation37]. (e) (Left) Energy distribution curves (EDCs) on the holelike pocket plotted as a grayscale image, and (right) location of the Fermi momentum on the holelike pocket. (f) Momentum dependence of the superconducting gap derived from fitting the symmetrized EDCs [Citation38]](/cms/asset/9e0091ce-ee26-4449-9ab6-9b69dc78bed8/tapx_a_1878931_f0004_oc.jpg)
4. Nematic superconductivity in 122 system
Owing to the absence of magnetic ordering and its competition with superconductivity in FeSe, one can hardly conclude a universal behavior of nematic superconductivity in the Fe-based family. Instead, the 122-system demonstrates the typical superconductors, which behaves a competition between superconductivity and various transitions in the under-doped state, including the transitions of antiferromagnetic ordering, lattice distortion, and nematic ordering [Citation15–19,Citation42]. Here, we focus on the discussion of nematic superconductivity on the 122-system. Generally, the gap structure of the 122-system [Citation43] is considered as multi-gap -wave, including a large gap on the two small hole-like and electron-like Fermi surface (FS) sheets, and a small gap on the large hole-like FS. However, both gaps are nodeless and nearly isotropic around their respective FS sheets. In this case, it seems impossible to observe a symmetry breaking from the isotropic gap system.
In 2017, however, a nematic order was also observed in an optimally doped hole-type BaK
Fe
As
superconductor in the superconducting state [40]. It shows a strong anisotropy in the in-plane magnetoresistivity on ultra-thin single crystals, which substantially enhanced the resistance and thus improved the sensitivity of the measurements as shown in . Similar to that in the normal state, the nematicity is basically along the
or
direction, which corresponds to
or
in the momentum space. However, it should be noted that there is also a weak
anisotropic component. Since the sample was fabricated into a Corbino shape on an ultrathin crystal, the Lorentz force would not induce the anisotropic resistivity, and thus these results represent an intrinsic nematic superconducting state.
Figure 5. (a) Schematic image of the Corbino-shape sample for in-plane magnetoresistivity measurements. The electric current is lead to flow radially from the center to the outermost electrode, and the magnetic field is applied within the -plane. (b) The angular dependence of the in-plane magnetoresistivity. (c) The angular dependence of the second magnetic critical field at 38.4 K (
39 K). From theoretical model with
-wave,
, and
-wave symmetries (full red and blue lines depending on dominant component) [Citation40]
![Figure 5. (a) Schematic image of the Corbino-shape sample for in-plane magnetoresistivity measurements. The electric current is lead to flow radially from the center to the outermost electrode, and the magnetic field is applied within the ab-plane. (b) The angular dependence of the in-plane magnetoresistivity. (c) The angular dependence of the second magnetic critical field at 38.4 K (Tc≈39 K). From theoretical model with s-wave, dx2−y2, and dxy-wave symmetries (full red and blue lines depending on dominant component) [Citation40]](/cms/asset/b4b50752-5e79-4e7a-93fa-be86a1e43694/tapx_a_1878931_f0005_oc.jpg)
Until recently, all the discovered nematic orders in FeSCs have B (
) symmetry, namely, along the nearest Fe-Fe direction [Citation15–24,Citation42]. Recently, however, nematic order/fluctuation with B
(
) symmetry, rotated by 45 degrees from the conventional B
nematicity, has been discovered in the heavily hole-doped compound
Fe
As
(
= K, Rb, Cs) as shown in [Citation41]. Furthermore, elastoresistance measurements in the normal state on this system also revealed a
nematicity [Citation44]. ARPES measurements have shown that the Fermi surfaces of the heavily hole-doped FeSCs only have hole pockets centered at the
point without electron pockets at the M point [Citation45–47]. This is very different from the under- and optimally doped samples where both electron and hole pockets are present [Citation15–18,Citation42].
Figure 6. (a) Topographic image of type B surface (b) Sketch of the unfolded Brillouin zone of RbFeAs
and its relation to the FFT pattern. (c) and (d) FFT profile of type B surface along the
and
directions, respectively [Citation41]
![Figure 6. (a) Topographic image of type B surface (b) Sketch of the unfolded Brillouin zone of RbFe 2As 2 and its relation to the FFT pattern. (c) and (d) FFT profile of type B surface along the (π,π) and (π,−π) directions, respectively [Citation41]](/cms/asset/9dab2fd8-8e2a-4193-9222-ea9e188f6a8d/tapx_a_1878931_f0006_oc.jpg)
Since the Fermi-surface topology is special in heavily hole-doped Fe
As
(
= K, Rb, Cs), one may argue that such
-type nematic order is unique for this concentration or universal in the whole doping range. In the recent work from author, we have observed a nematic superconducting order in electron-doped BaFe
Ni
As
with
ranging from under-doped to heavily over-doped samples [Citation48]. As shown in , while the normal state in-plane magnetoresistances are found to be nearly isotropic, the observed critical temperature under the suppression of magnetic field along different angles shows a C
symmetry with maxima along the (
,
) direction, rotated by 45
from the nematicity along (0,
) or (
, 0) direction as widely discussed in the normal state. For the hole-type superconductor, the optimally doped sample Ba
K
Fe
As
displays a
symmetry as described above, but the maximum and minimum are basically along the Fe–Fe bond, or (0,
)/(
, 0) direction, being about 45
rotated from those of electron-doped BaFe
Ni
As
samples [Citation48]. However, such anisotropy is not aligned strictly along the (0,
)/(
, 0) direction, but another weak anisotropy contributes as well, indicating the contribution of (
,
) nematicity. For the under-doped samples, we found that the nematic superconductivity mainly shows a
symmetry, although a weak
symmetry contributes to the anisotropy as well. In other word, the remarkable phenomenon from our results is that, even in the overdoped region where the symmetry breaking force from structure transition along (0,
)/(
, 0) direction is absent, a
symmetry breaking in the superconducting state along (
,
) direction is universal in our electron- and hole-doped samples, instead of inheriting from the normal state crystal symmetry of
or
along (0,
)/(
, 0) direction or induced by the according structure transitions.
Figure 7. (a)-(d), Angular dependence of the normalized for BaFe
Ni
As
with
=0.065, 0.075, 0.1 and 0.18, respectively [48]. The
is defined where the slopes of RT curves have their maximums under a field of 9 T. The angle here corresponds to the angle between the external field and
-axis. All curves demonstrate an obvious
symmetry, while the under-doped sample with
=0.075 demonstrates
symmetry. (e)-(g), Angular dependence of the normalized
for Ba
K
Fe
As
with
=0.2, 0.25, and 0.5, respectively [40]. The measurement condition is the same as above. Also, the under-doped samples show a
symmetry, while the optimally doped sample reveals a
symmetry basically along the
direction
![Figure 7. (a)-(d), Angular dependence of the normalized Tc/Tc−max for BaFe 2−xNi xAs 2 with x=0.065, 0.075, 0.1 and 0.18, respectively [48]. The Tc is defined where the slopes of RT curves have their maximums under a field of 9 T. The angle here corresponds to the angle between the external field and a-axis. All curves demonstrate an obvious C2 symmetry, while the under-doped sample with x=0.075 demonstrates C4 symmetry. (e)-(g), Angular dependence of the normalized Tc/Tc−max for Ba 1−yK yFe 2As 2 with y=0.2, 0.25, and 0.5, respectively [40]. The measurement condition is the same as above. Also, the under-doped samples show a C4 symmetry, while the optimally doped sample reveals a C2 symmetry basically along the (π,0)/(0,π) direction](/cms/asset/f30c3e1e-0914-4501-952b-f576814439cb/tapx_a_1878931_f0007_oc.jpg)
5. Discussion
As we have discussed the nematic superconductivity of both electron- and hole-type superconductors, we can now provide a phase diagram for the nematic superconductivity as given in . The most direct reason, the nematicity superconductivity demonstrates obviously an anisotropic behavior, which is inconsistent with the isotropic
-wave pairing symmetry as well studied in the optimally doped samples [Citation15–21], rather than the four-fold symmetric
-wave pairing [Citation49–51]. Of course, once mixing the commonly considered
-wave with the rarely discussed
-wave, we can obtain a gap orienting along the (
,
) direction as discussed in the hole-type system in Ref. [Citation40]. However, since the
-wave and
-wave pairings belong to different irreducible representations of the
point group, they can hardly mix spontaneously [Citation14]. The possible driven mechanism is still an open question.
Figure 8. Phase diagram of 122-type superconductors. The yellow region demonstrates the nematic fluctuation. A narrow light orange region reveals the nematic order for the electron-doped samples. The light red region corresponds to the orthorhombic magnetism. The light blue area exhibits to superconducting dome with the existence of the nematic superconducting state. The schematic diagrams of red curves in the crystal lattices show the symmetries obtained by transport measurement with different doping levels
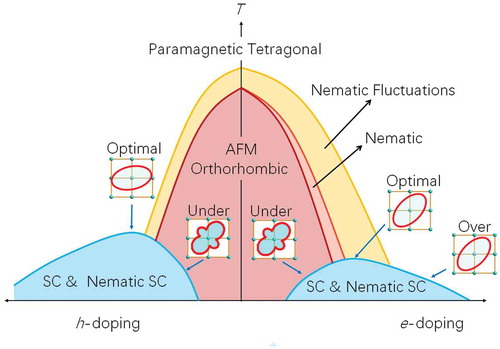
In the whole superconducting dome in electron-type, there is a nematic superconducting order with a symmetry along the (
,
) direction, obtained by measuring the angular dependencies of the magnetoresistivity,
, and upper critical field
. Such a nematic state also exists in the underdoped and optimally doped hole-type samples, as well as the heavily hole-doped
Fe
As
[Citation41,Citation44], although the optimally doped sample has a dominant
nematicity. These results demonstrate unambiguously the universal presence of a (
,
) nematic superconducting order in the whole superconducting dome in both the electron- and hole-doped BaFe
As
. Such nematicity is most pronounced in the heavily under- and over-doped regimes for both the electron- and hole-doped cases.
Since the (,
) nematic superconductivity extends to the overdoped region, we can hardly attribute it to be driven by the tetragonal-to-orthogonal structural transition or magnetic ordering, which exist only in the underdoped region as shown in . For the
nematic order, however, more and more evidence shows that the nematicity is driven by magnetic fluctuations [Citation14], although the orbital scenario is also proposed in the special case as FeSe [Citation12]. As discussed in section 2, in the magnetic scenario, the nematic orders differing along the
and
directions are normally attributed to some quadrupole magnetic orders or fluctuations. In the orbital scenario, the occupations of the
and
orbits are different, which induces divergent charge fluctuations breaking the equivalence between the
and
directions.
To explain the new-type (,
) nematic superconductivity, some theoretical studies have been reported for the heavily hole-doped
Fe
As
(
= Rb, Cs) [Citation52–54]. Onari and Kontani applied the itinerant picture [Citation52], and found that the origin of the nematicity can be attributed to the
bond order driven by the spin fluctuations in the
orbits, which corresponds to the breaking of the equivalence in the next-nearest-neighbor hoppings along the two diagonals of the Fe-only square lattice. Alternatively, Wang
. proposed a localized picture based on an extended bilinear-biquadratic Heisenberg model [53], and suggested that incommensurate
magnetic fluctuations dominate the (
,
) nematic. Quite recently, Borisov
. [Citation54] suggested a
magnetic states origin based on first-principles calculations, for which the symmetry-breaking composite order parameters can condense even in the paramagnetic phase, resulting in the so-called vestigial phases.
The itinerant scenario seems unlikely to be consistent with the experimental results, because the nematicity exists in the whole doping range, regardless of the hole- or electron-type. Thus, the Fermi surface structures are completely different with respect to the doping state, so the nematicity should not exist steadily in the itinerant nature. The highly possible origin is that the
nematicity comes from local moments, while the
nematicity originating from the itinerant electrons. Here, the
nematicity has a strong doping dependence, while the
one originating from the localized electrons resists the doping concentration.
On the other hand, in a very recent work [Citation55], the important roles disorder or strain play in the samples have also been highlighted. While the authors demonstrate that the elastoresistance of CsFeAs
is dominated by the symmetric A
strain component rather than the asymmetry B
and B
channels, they also find that gluing samples can induce irregular tension and damage samples microscopically, thus significantly affect the result if the thermal expansivities of samples and substrates mismatch, which could explain the distinction between their results and the others. Such finding has shed light on the importance of experiment configurations and promoted the investigations of the possible origins of observed
nematicity.
6. Summary
We gave an overview of nematic order in the superconducting state of the Fe-based family. The nematic electronic state along /
direction was widely found in the under-doped case of various Fe-based superconductors, which was basically considered to be related to the antiferromagnetic ordering, structure distortion, or even the orbital fluctuation. However, such a nematic state was observed in only the normal state, how it can be correlated with the superconducting state is still unclear. In this respect, an important progress is the observation of a newly nematic order in the superconducting state.
Firstly, we considered the superconducting gap symmetry which may break the lattice symmetry, once an anisotropic gap or gap component exists. The ARPES measurement reports a significant
-symmetry on the
pocket in lightly sulfur doped FeSe, reveals a
/
nematic superconductivity. For the 122-type superconductors, ARPES results suggested an isotropic
-wave pairing symmetry, which can not induce symmetry breaking. Nevertheless, the transport measurements on the optimally doped hole-type Ba
K
Fe
As
superconductor show a strong
-symmetry basically along
/
direction, for which the ordering within the under-doped state should be absent. The major development of this study is to study on the in-plane magnetoresistivity on a Corbino-shape ultra-thin single crystal, which substantially enhanced the resistance to improve the measurement sensitivity and also avoid the Lorentz force which is unavoidable in the traditional methods. Although it was argued that the nematicity in the superconducting state may result from the normal-state nematic fluctuations typically surviving well above
, the following study from the quasi-particle-interference measurement on the heavily hole-doped RbFe
As
, whose doping concentration is far away from the nematic phase of the normal state in the phase diagram, demonstrates the nematic superconducting state as well. However, the symmetry of such nematicity is broken from
lattice symmetry to along
(the Fe–As bond) direction, indicating a newly type nematicity.
In our quite recent work, we have studied nematic superconductivity of the electron-doped BaFeNi
As
within the whole doping range. All samples share a
symmetry with gap maxima along the (
,
) direction, rotated by 45
from the nematicity along (0,
) or (
, 0) direction as widely discussed in the normal state. In addition, we also observed the (
,
)-type nematicity in the under-doped and optimally doped hole-type Ba
K
Fe
As
, with
= 0.2 and 0.25, even a weak (
,
)-nematicity component for the optimally doped Ba
K
Fe
As
, with
=0.5. In conclusion, we propose that the newly discovered (
,
) nematic superconducting order might be a universal feature in the electron- and hole-doped 122-type FeSCs.
Disclosure statement
No potential conflict of interest was reported by the authors.
Additional information
Funding
References
- Hinkov V, Haug D, Fauqué B, et al. Electronic liquid crystal state in the high-temperature superconductor YBa2Cu3O6.45. Science. 2008;319:597–600.
- Daou R, Chang J, LeBoeuf D, et al. Broken rotational symmetry in the pseudogap phase of a high –Tc superconductor. Nature. 2010;463:519–522.
- Okazaki R, Shibauchi T, Shi HJ, et al. Rotational symmetry breaking in the hidden-order phase of URu2Si2. Science. 2011;331:439–442.
- Ronning F, Helm T, Shirer KR, et al. Electronic in-plane symmetry breaking at field-tuned quantum criticality in CeRhIn5. Nature. 2017;548:313–317.
- Chu JH, Kuo HH, Analytis JG, et al. Divergent nematic susceptibility in an iron arsenide superconductor. Science. 2012;337:710–712.
- Kuo HH, Chu JH, Palmstrom JC, et al. Ubiquitous signatures of nematic quantum criticality in optimally doped Fe-based superconductors. Science. 2016;352:958–16.
- Fisher IR, Degiorgi L, Shen ZX, In-plane electronic anisotropy of underdoped ’122ʹ Fe-arsenide superconductors revealed by measurements of detwinned single crystals, Reports on Progress in Physics 74, 124506 (2011).
- Liu Z, Gu Y, Zhang W, et al. Nematic quantum critical fluctuations in BaFe2-xNixAs2. Phys Rev Lett. 2016;117:157002.
- Tanatar MA, Böhmer AE, Timmons EI, et al. Origin of the resistivity anisotropy in the nematic phase of FeSe. Phys Rev Lett. 2016;117:127001.
- Gastiasoro MN, Paul I, Wang Y, et al. Emergent defect states as a source of resistivity anisotropy in the nematic phase of iron pnictides. Phys Rev Lett. 2014;113:127001.
- Ying JJ, Wang XF, Wu T, et al. Measurements of the anisotropic in-plane resistivity of underdoped FeAs-based pnictide superconductors. Phys Rev Lett. 2011;107:067001.
- Baek SH, Efremov DV, Ok JM, et al. Orbital-driven nematicity in FeSe. Nat Mater. 2015;14:210.
- Alexander GP, Chen BG, Matsumoto EA, et al. Colloquium: disclination loops, point defects, and all that in nematic liquid crystals. Rev Mod Phys. 2012;84:497.
- Fernandes RM, Chubukov AV, Schmalian J. What drives nematic order in iron-based superconductors? Nat Phys. 2014;10:97.
- Paglione J, Greene RL. High-temperature superconductivity in iron-based materials. Nat Phys. 2010;6:645.
- Johnston DC. The puzzle of high temperature superconductivity in layered iron pnictides and chalcogenides. Adv Phys. 2010;59:803.
- Hirschfeld PJ, Korshunov MM, Mazin II. Gap symmetry and structure of Fe-based superconductors, Rep Prog Phys. 2011;74:124508.
- Chubukov A. Pairing mechanism in Fe-based superconductors. Annu Rev Condens Matter Phys. 2012;3:57.
- Mazin II. Superconductivity gets an iron boost. Nature. 2010;464:183–186.
- Wang F, Lee DH. The electron-pairing mechanism of iron-based superconductors. Science. 2011;332:200–204.
- Norman MR. The challenge of unconventional superconductivity. Science. 2011;332:196–200.
- Luo H, Lu X, Zhang R, et al. Electron doping evolution of the magnetic excitations in BaFe2-xNixAs2. Phys Rev B. 2013;88:144516.
- Prozorov R, Koczykowski M, Tanatar MA, et al. Interplay between superconductivity and itinerant magnetism in underdoped Ba1-xKxFe2As2 (x=0.2) probed by the response to controlled point-like disorder. Npj Quantum Material. 2019;4:34.
- Zhao J, Rotundu CR, Marty K, et al. Effect of electron correlations on magnetic excitations in the isovalently doped iron-based superconductor Ba(Fe1-xRux)2As2. Phys Rev Lett. 2013;110:147003.
- Kreisel A, Hirschfeld PJ, Andersen BM. On the remarkable superconductivity of FeSe and its close cousins. Symmetry-Basel. 2020;12:1402.
- Chen T, Yi M, Dai PC. Electronic and magnetic anisotropies in FeSe family of iron-based superconductors. Front Phys. 2020;8:314.
- Bohmer AE, Kreisel A. Nematicity, magnetism and superconductivity in FeSe. J Phys-Condens Matter. 2018;30:023001.
- Shibauchi T, Hanaguri T, Matsuda Y. Exotic superconducting states in FeSe-based materials. J Phys Soc Jpn. 2020;89:102002.
- Chu JH, Analytis JG, De Greve K, et al. In-plane resistivity anisotropy in an underdoped iron arsenide superconductor. Science. 2010;329:824–826.
- Licciardello S, Buhot J, Lu J, et al. Electrical resistivity across a nematic quantum critical point. Nature. 2019;567:213–217.
- Pratt DK, Tian W, Kreyssig A, et al. Coexistence of competing antiferromagnetic and superconducting phases in the underdoped Ba(Fe0.953Co0.047)2As2. Phys Rev Lett. 2009;103:087001.
- Wen J. Magnetic neutron scattering studies on the fe-based superconductor system Fe1+yTe1-xSex. Ann Phys. 2015;358:92.
- Zhang Y, Chen F, He C, et al. Orbital characters of bands in the iron-based superconductor BaFe1.85Co0.15As2. Phys Rev B. 2011;83:054510.
- Song CL, Wang YL, Cheng P, et al. Direct observation of nodes and twofold symmetry in FeSe superconductor. Science. 2011;332:1410–1413.
- Lu DC, Lv YY, Li J, et al. Elliptical vortex and oblique vortex lattice in the FeSe superconductor based on the nematicity and mixed superconducting orders. npj Quantum Material. 2018;3:12.
- Sprau PO, Kostin A, Kreisel A, et al. Discovery of orbital-selective cooper pairing in FeSe. Science. 2017;357:75–80.
- Hashimoto T, Ota Y, Yamamoto HQ, et al. Superconducting gap anisotropy sensitive to nematic domains in FeSe. Nat Commun. 2018;9:282.
- Liu D, Li C, Huang J, et al. Orbital origin of extremely anisotropic superconducting gap in nematic phase of FeSe superconductor. Phys Rev X. 2018;8:031033.
- Kang J, Chubukov AV, Fernandes RM. Time-reversal symmetry-breaking nematic superconductivity in FeSe. Phys Rev B. 2018;98:064508.
- Li J, Pereira PJ, Yuan J, et al. Nematic superconducting state in iron pnictide superconductors. Nat Commun. 2017;8:1880.
- Liu X, Tao R, Ren MQ, et al. Evidence of nematic order and nodal superconducting gap along [110] direction in RbFe2As2, Nat. Commun. 2019;10:1039.
- Dai P, Hu J, Dagotto E. Magnetism and its microscopic origin in iron-based high-temperature superconductors. Nat Phys. 2012;8:709.
- Ding H, Richard P, Nakayama K, et al. Observation of fermi-surface–dependent nodeless superconducting gaps in Ba0.6K0.4Fe2As2. Europhys Lett. 2008;83:47001.
- Ishida K, Tsujii M, Hosoi S, et al. Novel electronic nematicity in heavily hole-doped iron pnictide superconductors. Proc Nat Acad Sci. 2020;117:6424.
- Sato T, Nakayama K, Sekiba Y, et al. Band structure and fermi surface of an extremely overdoped iron-based superconductor KFe2As2. Phys Rev Lett. 2009;103:047002.
- Yoshida T, Nishi I, Fujimori A, et al. Fermi surfaces and quasi-particle band dispersions of the iron pnictides superconductor KFe2As2 observed by angle-resolved photoemission spectroscopy. J Phys Chem Solids. 2011;72:465–468.
- Okazaki K, Ota Y, Kotani Y, et al. Octet-line node structure of superconducting order parameter in KFe2As2. Science. 2012;337:1314.
- Dong Y, Lv Y, Xu Z, et al. Observation of a ubiquitous (π,π)-type nematic superconducting order in the whole superconducting dome of ultra-thin BaFe2-xNixAs2 in single crystals. Present at Young Scientists Symposium on Superconductivity-2019, Beijing. 2019 (unpublished).
- Koike Y, Takabayashi T, Noji T, et al. Fourfold symmetry in the ab plane of the upper critical field for single-crystal Pb2Sr2Y0.62Ca0.38Cu3O8: evidence for dx2-y2 pairing in a high-Tc superconductor. Phys Rev B. 1996;54:R776.
- Mao ZQ, Maeno Y, NishiZaki S, et al. In-plane anisotropy of upper critical field in Sr2RuO4. Phys Rev Lett. 2000;84:991.
- Wu J, Bollinger AT, He X, et al. Spontaneous breaking of rotational symmetry in copper oxide superconductors. Nature. 2017;547:432–435.
- Onari S, Kontani H. Origin of diverse nematic orders in Fe-based superconductors: 45° rotated nematicity in AFe2As2(A=Cs,Rb). Phys Rev B. 2019;100:020507.
- Wang Y, Hu W, Yu R, et al. Broken mirror symmetry, incommensurate spin correlations, and B2g nematic order in iron pnictides. Phys Rev B. 2019;100:100502.
- Borisov V, Fernandes RM, Valent R. Evolution from B2g nematics to B1g nematics in heavily hole-doped iron-based superconductors. Phys Rev Lett. 2019;123:146402.
- Wiecki P, Haghighirad -A-A, Weber F, et al. Böhmer, Dominant in-plane symmetric elastoresistance in CsFe2As2. Phys Rev Lett. 2020;125: 187001