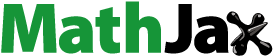
ABSTRACT
The nitrogen-vacancy (NV) center is one of the major platforms in the evolving field of quantum technologies. The inertial surveying technology based on NV centers in diamond is a developing field with both scientific and technological importance. Quantum measurement using the solid-state spin of the NV center has demonstrated potential in both high-precision and small-volume low-cost devices. In terms of rotation measurement, the optically detected magnetic resonance has provided a perspective of the rotation measurement mechanism via the solid-state spin of the NV center. A new type of gyroscope based on the solid-state spin in diamond according to the theory has attracted considerable attention. In addition, combined with the ingenious quantum mechanics manipulation and coupling mechanism, acceleration measurement can be achieved through an efficient quantum detection technology of the NV center. This review summarizes the recent research progress in diamond-based inertial measurement, including sensitivity optimization methods for inertial measurement systems based on the NV center.
Graphical abstract
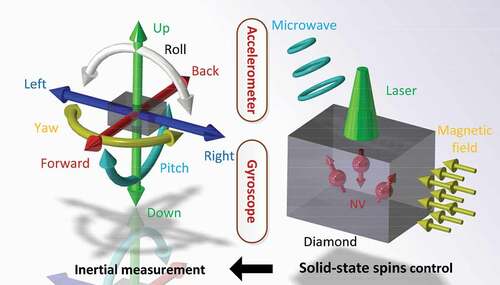
1. Introduction
High-precision inertial measurement plays a paramount important role in both fundamental research and applications [Citation1]. Rotation and acceleration measurements in an inertial frame are vital for modern advanced navigation systems [Citation2]. In recent years, quantum physics-based technologies have played an important role in high-precision measurements, and their accuracy has improved considerably compared with that of classical systems. The nitrogen-vacancy (NV) center () has recently emerged as a powerful quantum system that combines the good coherence properties and control techniques of atomic systems with the small size and fabrication capabilities of solid-state devices [Citation3]. The advantages of the NV center quantum technology are reflected in various fields, such as quantum sensing [Citation4–14] and quantum computing [Citation15–17]. After their rapid development in the past decades, NV center quantum inertial measurement systems have been recognized as promising candidates for inertial measurement systems [Citation18,Citation19].
Figure 1. Energy level diagram of the NV center spins in diamond. The inset shows the crystal structure of the NV center in diamond. Two adjacent carbon atoms (red spheres) are substituted by a nitrogen atom (brown sphere) and a vacancy (green sphere). With the phonon sideband and singlet states, the electron spin can be initialized to state using a 532 nm laser. The electron spin-state population is detected by monitoring the 600–800 nm fluorescence signal. The zero phonon line is at 637 nm and the zero-field splitting of the triplet ground state is at 2.87 GHz [Citation20].
![Figure 1. Energy level diagram of the NV center spins in diamond. The inset shows the crystal structure of the NV center in diamond. Two adjacent carbon atoms (red spheres) are substituted by a nitrogen atom (brown sphere) and a vacancy (green sphere). With the phonon sideband and singlet states, the electron spin can be initialized to ms=0 state using a 532 nm laser. The electron spin-state population is detected by monitoring the 600–800 nm fluorescence signal. The zero phonon line is at 637 nm and the zero-field splitting of the triplet ground state is at 2.87 GHz [Citation20].](/cms/asset/ae3519c4-15a2-40c6-9252-f348f61a3d98/tapx_a_2004921_f0001_oc.jpg)
With regard to rotation measurement, Wood et al. [Citation21–23] reported a measurement method using a single NV-spin phase shift arising directly from physical rotation. The rotation is obtained by measuring the phase difference between a microwave driving field and a rotating two-energy level electron spin system, and the phase shift can accumulate nonlinear behaviors over time. The carrier’s rotation information can be obtained by measuring the accumulation of the quantum geometric phase. This solid-state spin inertial sensor based on NV center is competitive with conventional gyroscopes in terms of sensitivity, cost, and size. For acceleration measurements, a gravimeter based on the spin superposition of the NV center can achieve a higher precision compared with that of the atomic interference gravimeter, because of the higher mass of the mechanical resonator in the former system [Citation24]. In addition, compared to conventional atomic inertial sensors, inertial sensors based on the solid-state spin of NV center have faster start time. Moreover, because they can operate at normal temperature and have more relax requirements of working temperature, an air chamber is not required, so they have the potential for future miniaturization.
This review focuses on inertial measurement using solid-state spins of the NV Center to guide future research efforts. First, the concept and background of the inertial measurement with solid-state spins of the NV center in diamond are introduced in Section 1. Then, the development of the spin-based rotation inertial measurement is presented in Section 2, with Section 2.1 and Section 2.2 describing the development of the spin-based rotation measurement and spin-based acceleration measurement, respectively. Section 3 discusses the sensing mechanisms of spin-based rotation inertial measurements, namely, the solid-state spin rotation measurement (Section 3.1) and solid-state spin acceleration measurement (Section 3.2). Subsequently, the methods for improving the sensitivity of gyroscopes (Section 4.1) and accelerometers (Section 4.2) are reviewed in Section 4. Finally, the summary and outlook are presented in Section 5.
2. Development of spin-based inertial measurement
2.1 Spin-based rotation measurement
The NV center is an atomic-scale defect point color center, which has good optical [Citation25] and spin properties [Citation26]. The NV center can be optically addressed, and the spin state of the NV center can be observed optically by the fluorescence [Citation25]. The long coherence time of the NV− electronic spin [Citation26,Citation27] at both room temperature and cryogenic condition makes it possible to realize high precise sensing utilizing the quantum resources at ambient conditions. Physical quantities that coupled with the NV spin can be sensed with high precision, including magnetic field [Citation28–30], electric field [Citation31], force/strain field [Citation32] and thermometry [Citation33–35]. The best sensitivity achieves 0.43 pT [Citation36], 200 V
[Citation31] and 76 mK
[Citation37], respectively.
Recently, the NV-based quantum sensing has been used for inertial measurements. In 2012, Maclaurin et al. proposed a scheme for detecting the phase induced by the rotation of a system with a single NV center [Citation38], Ledbetter et al. and Ajoy and Cappellaro et.al. proposed the NV-based gyroscopes [Citation39,Citation40]. The sensitivity of the proposed scheme [Citation39] without and with the use of 14N nuclear spin is and
. In 2017 and 2018, Maclaurin et al. further explored the effect of rotation-induced pseudo-fields on sample spins, using a fast-spinning diamond containing either an ensemble of NV centers or a single NV center mounted on a rotor, providing a technical reference for the development of accurate NV center-based spin measurements [Citation21,Citation23]. In 2021, utilizing the
nuclear spin within the corresponding NV center and the double-quantum pulse protocol, an integral rotating scheme was designed and demonstrated in the laboratory. The rotation sensitivity and zero bias stability are
and
respectively [Citation41]. The experimental progress stimulated the practicalization of gyroscopes. In 2016, Zhang et al. in Beihang University put forward a structure of an NV-based gyroscope in which a bilateral layout design of coils was adopted to guarantee the uniformity of the distribution of the MW and the radio frequency (RF) field [Citation1]. In 2018, Song et al. in China Academy of space technology showed a
-NV rotation sensing system, which is analogous to the scheme mentioned above [Citation42]. In 2021, Soshenko et al. at Lebedev Physical Institute obtained the dynamic phase difference induced by the macroscopic rotation of the diamond via the double-quantum Ramsey spectroscopy. The validity of their measurement setup based on the
nuclear spin ensemble under sub-Hz rotation was verified by a MEMS gyroscope, which further promotes the miniaturization and integration of diamond gyroscope [Citation43].
2.2 Spin-based acceleration measurement
Acceleration measurements based on interferometry have evolved from optics to matter waves [Citation44,Citation45]. Matter-wave interferences have been demonstrated with neutrons [Citation46,Citation47], atoms [Citation48,Citation49], and electrons. The relatively shorter de Broglie wavelength of matter waves can increase the sensitivity of phase shift measurements [Citation50]. Diamond particles levitating in optical tweezers act as nanoscale resonators, facilitating the creation of macroscopic superposition states, which is a remarkable feature of quantum mechanics [Citation51,Citation52]. Inspired by this, a superposition generation scheme of a macroscopic mechanical resonator hybrid with an NV center was proposed to improve the interferometric precision of the gravitationally induced phase shift [Citation24,Citation53]. Due to their broad application prospects, techniques for coupling mechanical resonators with the spin of the NV center have been developed [Citation54,Citation55]. A hybrid system with an NV center [Citation56] or a magnetic tip [Citation57] attached to the end of a cantilever, with the help of a magnetic field gradient, can sense the motion of the mechanical cantilever using a single [Citation58] or multiple NV centers [Citation59,Citation60]. With the development of optomechanics [Citation61–63], an optical force levitated diamond containing an NV center has emerged as a powerful scheme for realizing a high-quality factor [Citation64,Citation65].
3. Sensing mechanism of spin-based inertial measurement
3.1 Spin-based rotation measurement
shows the sensing principle of the NV-based rotation measurement. The measured precession frequency , which is completely determined by the external field in a non-rotating frame, changes with different angular velocities. The rotation information can be calculated from the acquired signal and a measurable external field.
where is the measured frequency,
is the Larmor precession frequency, and
is the rotation angular velocity [Citation66]. The quantum phase accumulated on the spin states is then expressed as:
where is the rotation time and
is the uniform angular velocity of the carrier. By measuring the spin states, the angular velocity of the carrier is then obtained. Notably, the influences of external parameters, such as magnetic fields, can be examined independently during the operation of the carrier [Citation39,Citation40]. The carrier rotation information can be obtained by measuring the accumulation of the quantum phase.
Figure 2. Mechanism diagram of NV-based rotation measurement. In a non-rotating state, the spin magnetic moment processes in the direction of the external magnetic field, and the precession frequency is proportional to the applied magnetic field (black arrow). In a rotating state, the precession frequency increases by a term proportional to the rotational angular velocity. The 532 nm laser is used for spin polarization, the microwave source is used for spin manipulation, and the detected fluorescence contains rotation information.
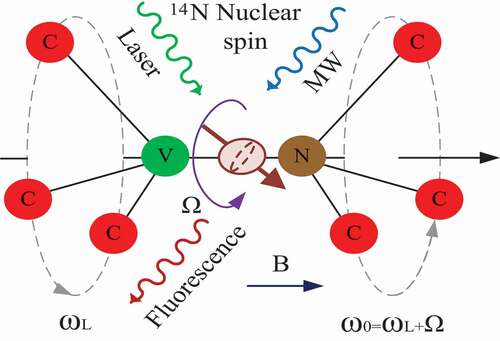
Consider a solid-state NV spin system with an electron spin and
nuclear spin
. The ground-state Hamiltonian of the NV− center electron spin and the adjacent
nuclear spin is [Citation67]:
where is the zero-field splitting;
and
are the nuclear and electron gyromagnetic ratios
;
is the external magnetic field;
is the intrinsic quadrupole interaction;
and
is the hyperfine constant. An external magnetic field can realize the ground-state Zeeman splitting of the NV electronic spin, thus forming a two-level system. Microwave pulses are required to achieve spin-state flipping and manipulation. An off-resonance laser (ex. 532 nm) is chosen to help electron spin polarization [Citation68–71]. The
state will decay directly to the ground state and
state will shelve into a non-radiative metastable state preferentially. The fluorescence of
state is weaker, and thus the spin state can be readout by the photon detector. Thus, the spin-state population can be determined via fluorescence detection owing to the phonon sideband and non-radiative transition of the metastable state [Citation72]. The long coherence time of NV center (1.8 ms at room temperature [Citation26] and ~ 1 s at liquid nitrogen temperature [Citation27]) allows complicated control methods [Citation73] and high sensitive measurements of the physics quantities coupled to the NV center [Citation74].
Although the NV center has good optical and spin properties, the coherence time is limited [Citation29,Citation75] and the readout efficiency [Citation29,Citation76] depends on the photonic structure and operation temperature, especially for the NV center ensemble. The adjacent nuclear spin can be used as a sensor, which has a longer relaxation time and can be readout with high efficiency by quantum logic [Citation11,Citation77]. The
nuclear spin can be conveniently polarized, controlled and readout by the NV center. Both excited-state level anti-crossing [Citation78,Citation79], dynamic nuclear polarizing techniques [Citation80–84] is applied to polarize the
nuclear spin. Due to the development of the NV-based quantum information, the high-fidelity controls of the adjacent
nuclear spin and different quantum gates are realized in Ref [Citation16,Citation85]. The nuclear spin state can be readout with high fidelity [Citation86,Citation87] enhanced by the quantum non-demolition detection [Citation86].
Note that the natural quantization axis of the NV is set by the host diamond crystal orientation, and rotating the crystal can effectively induce rotation of the qubit [Citation22]. The large quantum superposition of nanomechanical resonator can be achieved with the help of NV center [Citation57,Citation64,Citation88,Citation89]. To improve the sensitivity of the mechanical measurement, diamonds hosting NV centers have been trapped both in liquid [Citation89,Citation90] and under vacuum [Citation91–94] for spin readout, which are important steps towards coupling spins to macroscopic particle motion. Ramsey interferometry is a fundamental method used in atomic physics and quantum measurements, one example of which is the preparation of the ground state of motion of macroscopic oscillators [Citation58,Citation65,Citation95,Citation96]. Notably, the influences of external parameters, such as magnetic fields, can be examined independently during the operation of the carrier [Citation39,Citation40]. The carrier rotation information can be obtained by measuring the accumulation of the quantum geometric phase (). Consider an adiabatic angular velocity measurement system whose Hamiltonian depends on the magnetic field and evolves in cycles. The research presented in [Citation97] is based on the coupling characteristics of the Hamiltonian control of the NV center spin system and the quantum geometric phase in the Bloch space (). Depending on the angle between the intrinsic axis of the NV center, the microwave field, and the axis of rotation, this rotation-induced phase can accumulate nonlinearly and be detected using a spin-echo scheme.
Figure 3. Schematic of rotation measurement. In the external magnetic field, the physical rotation of the carrier causes a change in the spin precession frequency, and the angular velocity information can be obtained via the quantum geometric phase [Citation38,Citation101].
![Figure 3. Schematic of rotation measurement. In the external magnetic field, the physical rotation of the carrier causes a change in the spin precession frequency, and the angular velocity information can be obtained via the quantum geometric phase [Citation38,Citation101].](/cms/asset/44053f0f-28dc-40a2-91f8-3302dd7e1421/tapx_a_2004921_f0003_oc.jpg)
In accordance with the above-mentioned sensing mechanism, more scholars have engaged in research on gyroscopes based on the NV center. Maclaurin [Citation38] proposed a method using a single NV center in a spinning diamond to measure the geometric phase accumulated by the electron spin. The Ramsey pulse sequence method and spin-echo pulse sequence method were suggested for geometric phase measurement. As illustrated in , Wood et al. [Citation21–23] utilized a rotor to spin the NV center in a magnetic field. The macroscopic rotation information can be obtained by observing the phase difference of the spin states. In the experiment of Soshenko [Citation43], rotation of the platform is measured using the nitrogen nuclear spins of an ensemble of NV centers; in particular, no external reference is used.
Figure 4. Principle of the rotating test bench. A single NV center in diamond on the spindle of a rotor. NV centers are optically prepared and addressed by a scanning confocal microscope. MW (produced by the micro coil), magnetic field, and laser are coupled to the NV center. The fluorescence signal is collected by the detector, which contains the angular velocity information [Citation22].
![Figure 4. Principle of the rotating test bench. A single NV center in diamond on the spindle of a rotor. NV centers are optically prepared and addressed by a scanning confocal microscope. MW (produced by the micro coil), magnetic field, and laser are coupled to the NV center. The fluorescence signal is collected by the detector, which contains the angular velocity information [Citation22].](/cms/asset/a268e1eb-c47c-4dff-a8fb-3196fff8c1d2/tapx_a_2004921_f0004_oc.jpg)
3.2 Spin-based acceleration measurement
Spin-oscillator coupling schemes have been proposed as accelerometers, whereby a spatial superposition is created through the interaction of an embedded two-level system such as an NV center with an external magnetic field gradient [Citation54,Citation98]. A hybrid system coupling a nanomechanical resonator to the electron spin of an NV center can achieve high-precision gravity measurement via matter-wave interferometry. For acceleration applications, large objects provide significant advantages over small objects. A relative detection accuracy for gravitational acceleration measurement has been estimated in [Citation24], and the measured relative phase difference is three orders of magnitude larger than that using the atom interference scheme. The proposed hybrid schemes are exhibited in .
Figure 5. Spin-oscillator coupling acceleration sensing scheme based on NV center with (a)the optical tweezer or (b)the cantilever [Citation24,Citation65,Citation98]. (c) The free-flight acceleration sensing scheme using a Ramsey interferometer facilitates the possibility of realizing a larger superposition. The spin superposition creation, the spin states flipping (at times and
) and wave packet mergence (at time
) are controlled by microwave pulses [Citation99,Citation100].
![Figure 5. Spin-oscillator coupling acceleration sensing scheme based on NV center with (a)the optical tweezer or (b)the cantilever [Citation24,Citation65,Citation98]. (c) The free-flight acceleration sensing scheme using a Ramsey interferometer facilitates the possibility of realizing a larger superposition. The spin superposition creation, the spin states flipping (at times t1 and t2) and wave packet mergence (at time t3) are controlled by microwave pulses [Citation99,Citation100].](/cms/asset/fdcc885b-5a7e-4439-8066-21573f5eb96a/tapx_a_2004921_f0005_oc.jpg)
As shown in , the center of mass (CoM) motion of the nanodiamond, which is trapped by a light force tweezer in a vacuum environment, couples to the spin of the built-in NV center of the diamond via a magnetic field gradient. A magnetic tip is placed in the vicinity of the diamond to induce the required magnetic field gradient along the z direction. Suppose that the trapping frequency is sufficiently small compared with
and
to neglect the motion effect along the x and y directions. Considering the Earth’s gravitational field, the corresponding Hamiltonian [Citation24] is
where is the spin-motion coupling strength, and
is the gravity-induced displacement,
is the electron Landé g-factor,
is the mass of the nanoparticle,
is the Bohr magneton, and
is the magnetic field gradient along the z acceleration-sensitive direction.
and
are annihilate operator and produce operator, respectively. It can be seen that the CoM motion of the oscillator varies with different eigenvalues of
.
Ramsey interferometry is utilized to detect the gravitational acceleration-induced phase shift of the resonator-spin hybrid system. By applying the first microwave pulse (
duration) after initialization of the solid-spin state, superposition of
and
states with the same amplitude is produced. The equilibrium position separation of the two states due to the spin-dependent acceleration is
. After recombination of the two classic paths at
, the states become
, and a relative phase shift [Citation24] appears between the two states.
The phase shift is proportional to the acceleration and can be determined through the application of the second microwave pulse to read the population of the spin state [Citation24].
Another spin-oscillator hybrid system is depicted in , where the magnetic tip is fixed on a cantilever resonator to couple the mechanical vibration mode with the NV center in diamond. It creates a magnetic gradient near the electronic spin of the NV center. Similar to the aforementioned scheme, a laser is used to initialize and measure the spin states, and the Ramsey sequence is adopted to manipulate and probe the spin states.
The untrapped nanodiamond interferometric scheme [Citation51,Citation99,Citation100] ( is proposed to measure the mass-independent dynamic phase induced by the spin-dependent gravitational potentials. The magnetic field gradient is still used to couple the CoM motion and spin states of the object. The spin superposition creation and spin state flipping (at times and
) are controlled by microwave pulses. After splitting, acceleration, deceleration, and mergence of the wave packet, a phase factor [Citation99] proportional to gravity can be acquired via fluorescence detection.
where ,
, and
are as previously defined,
is the angle between the applied magnetic field
and the direction of acceleration
, and
is the total free-fall time. Compared with the levitated method, this free-flight scheme facilitates the possibility of realizing a larger superposition because of its mass independence [Citation51].
4. Sensitivity optimization of spin-based inertial measurement
4.1 Sensitivity optimization of spin-based rotation measurement
When the magnetic field B is perturbated, its correction factor is [Citation29]:
where ,
and
are the first-, second- and third-level mini-disturbance items, respectively.
The error brought by the external magnetic field interference is as follows [Citation38]:
Although a single NV center in diamond has a long coherence time [Citation27], the measurement sensitivity is limited by the single-photon signal. In addition, it is difficult to integrate a single-photon counting system into a compact diamond gyroscope. Therefore, spin ensembles have been used to replace the single NV center in diamond. According to the fluorescence signal, the sensitivity limit of the rotation measurement is [Citation40]:
where is the number of the sensing spins,
is the coherence time,
is the fluorescence collection efficiency,
is the total of the preparation time
and the readout dead time
.
The comparation in sensitivity and size of some representative gyroscopes and the NV-based gyroscope [Citation39,Citation40,Citation101–110] are shown in , including ring laser gyroscope (RLG), fiber optical gyroscope (FOG), spin relaxation free (SERF) gyroscope, atom interferometer gyroscope (AIG), micro-electro-mechanical systems (MEMS) gyroscope, and NV-based gyroscope. As a new type of atomic gyroscopes, the NV-based gyroscope has the potential for medium-sensitivity and miniaturization in the future.
Table 1. Comparison in sensitivity and size of some representative gyroscopes and the NV-based gyroscope
Efforts have been made to enhance the sensitivity of gyroscopes. One of the keys to reach this goal is to increase the coherence of the NV center, which is a challenge when the diamond is in an optical trap and the spin angle becomes unstable. This will offer prospects for experiments, such as matter-wave interferometry [Citation89,Citation102], quantum gravity sensing [Citation111], strong coupling [Citation56,Citation57], and cat state preparation [Citation112], which rely on the ability to maintain a long coherence time for spin-state superpositions in a trapped object. The nuclear spin has a much longer coherence time than the electron spin, so the fundamental sensitivity can be significantly improved by using the
nuclear spin. Meanwhile, increasing the NV center density also improves the sensitivity. Considering these facts, Ledbetter et al. proposed the idea of developing a gyroscope based on nuclear spin ensembles in diamond [Citation39]. At the same time, in the proposal of Cappellaro [Citation40], the nitrogen nuclear spins controlled by the NV center are used as a ‘three-axis diamond gyroscope’. The relative phase of the spin state is measured and the carrier rotation is estimated from it with a theoretical sensitivity of
.
Optimized spin coherent control can also increase the sensitivity. The electronic spin resonance width can be determined mainly by coupling to the diamond strain and coupling to impurities [Citation113,Citation114]. Delord [Citation115,Citation116] applied a magnetic field that lifts the degeneracy between the state . The Rabi envelope decay is a characteristic of the environmental noise spectrum [Citation117]. However, the observed damping time does not provide direct access to
. In fact, the decay time can be longer than
and is determined largely by the employed microwave power [Citation118–120]. Jaskula [Citation121] used NV centers to sense local magnetic field fluctuations, estimated with a stable rotation measurement of several days, allowing navigation with loose or no requirement for geolocalization.
4.2 Sensitivity optimization of acceleration measurement
Preparing the quantum superpositions of a large object is challenging because of the interaction between the system and the environment [Citation122]. To achieve this goal, many methods have been investigated. In 2010, Romero-Isart et al. [Citation52] proposed a method that used an optical cavity levitating a nano-dielectric object. The resonance frequency dependence on the position of the trapped object yields an optomechanical coupling. Because the object was not attached to other mechanical pieces, this optomechanical scheme could avoid the main source of heating, thus facilitating the realization of ground-state cooling. Romero-Isart et al. [Citation122] further presented a cavity quantum optomechanical scheme to implement a matter-wave interference experiment. This scheme can be utilized to realize spatially separated superposition preparation of a large object and test of quantum mechanics, including wave function collapse models. In 2013, Yin et al. [Citation64] proposed a method for generating and detecting quantum superposition states of the CoM oscillation of a light force-trapped diamond based on magnetic field gradient–induced spin-optomechanical coupling. Their scheme entails the possibility of creating large quantum superpositions under feasible conditions. In the same year, Bose et al. [Citation98] presented a Ramsey interferometry scheme that satisfies five requirements (i.e. modest cooling, no cavities, no ensembles, no spatially resolved measurements, and controllable phase). The motion of a diamond bead, which is trapped by an optical tweezer, couples to the spin of the NV center via a static magnetic field gradient. The proposed method can measure the gravitational acceleration-induced relative phase difference of the spin state. An appropriate Ramsey scheme can attain good robustness against initial conditions, such as thermal fluctuations, yet places great demands on magnetic field control [Citation63].
In terms of acceleration measurement, considering the interferometric accelerometers, matter-wave interferometry may replace light interferometry to obtain an improved accuracy due to the shorter de Broglie wavelength of the former. The gravimeter based on a diamond resonator has higher precision than the cold atom interferometry gravimeter with suitable parameter settings, which can be explained by the mass difference. For the optical spin-oscillator sensing system and the cantilever spin-oscillator system mentioned before, the resonators are 1010 and 1016 times more massive than sodium atoms, respectively [Citation24]. EquationEq. (5)(5)
(5) can be rewritten as
. Given a fixed precision target and a fixed integration time, the size of the accelerometer can be considered to be inversely proportional to the mass of the resonator [Citation24]. Therefore, the NV center accelerometer has a higher miniaturization potential and is easier to integrate into a chip.
In the future, the realization and performance improvement of the accelerometer based on the NV center can be promoted in many aspects. First, as a basic system of light force accelerometers, optical tweezers have been widely investigated [Citation62,Citation123]. As a promising tool for creating macroscopic superposition states of the NV center spin, levitated optomechanics needs further studies to realize precise position detection of the nanodiamond [Citation124], reliable cooling control of the spin state [Citation125], and stable fluorescence collection [Citation63,Citation93]. Second, the sensitivity can be improved by using better fluorescence detection techniques to achieve shot noise performance [Citation29,Citation126]. The efficiency of photoconversion can be improved with the total internal reflection light guiding method [Citation127]. Additionally, the material properties [Citation128] can be optimized toward longer dephasing and coherence times of spin states. Finally, to improve the long-term performance of the sensor, further exploration could focus on the sensing and stabilization of temperature and magnetic fields through passive and active methods. Schemes that implement magnetic field feedback control [Citation121,Citation129] would result in better robustness of the device against field fluctuations in the environment.
5. Summary and outlook
The application of inertial surveying technology based on NV centers in diamond is a rapidly developing field with both scientific and technological importances. Over the past decades, quantum measurement using the solid-state spin of the NV center has demonstrated its potential in both high-precision applications and small-volume low-cost devices. In terms of rotation measurement, the optically detected magnetic resonance has provided a perspective on the rotation measurement mechanism via the solid-state spin of the NV center. The theory has been used to describe a gyroscope based on the solid-state spin in a diamond. In addition, with the ingenious ‘spin-oscillator’ coupling mechanism, acceleration measurement can be achieved via superposition generation. In summary, acceleration and angular velocity measurements based on the solid-state spin are in the experimental stage. The development of inertial measurement systems based on this principle is still facing huge challenges. Nevertheless, the NV center spin-based inertial measurement is an innovative technology at its early stage with rapid development. It has scientific exploratory significance and theoretical application value in terms of research and development of new inertial devices.
Acknowledgments
We are grateful to Jiawen Xu for the critical reading of the manuscript.
Disclosure statement
No potential conflict of interest was reported by the author(s).
Additional information
Funding
References
- Zhang C, Yuan H, Tang Z, et al. Inertial rotation measurement with atomic spins: from angular momentum conservation to quantum phase theory. Appl Phys Rev. 2016 Dec;3:041305.
- Yazdi N, Ayazi F, Najafi K. Micromachined inertial sensors. Proc IEEE. 1998 Aug;86:1640–20.
- Rembold P, Oshnik N, Müller MM, et al. Introduction to quantum optimal control for quantum sensing with nitrogen-vacancy centers in diamond. AVS Quantum Sci. 2020 Jun;2:024701.
- Taylor MA, Bowen WP. Quantum metrology and its application in biology. Phys Rep. 2016 Feb;615:1–59.
- Taylor MA, Janousek J, Daria V, et al. Biological measurement beyond the quantum limit. Nat Photonics. 2013 Feb;7:229–233.
- Morris PA, Aspden RS, Bell J, et al. Imaging with a small number of photons. Nat Commun. 2015 Jan;6:5913.
- Hu Z-K, Sun B-L, Duan X-C, et al. Demonstration of an ultrahigh-sensitivity atom-interferometry absolute gravimeter. Phys Rev A. 2013 Oct;88:043610.
- Demkowicz-Dobrzański R, Banaszek K, Schnabel R. Fundamental quantum interferometry bound for the squeezed-light-enhanced gravitational wave detector geo 600. Phys Rev A. 2013 Oct;88:041802.
- Purdy TP, Peterson RW, Regal CA. Observation of radiation pressure shot noise on a macroscopic object. Science. 2013 Feb;339:801–804.
- Adhikari RX. Gravitational radiation detection with laser interferometry. Rev Mod Phys. 2014 May;86:121–151.
- Urbach E LISAO, Sushkov AO, Urbach E, et al. Nuclear magnetic resonance detection and spectroscopy of single proteins using quantum logic. Science. 2016 Feb;351:836–841.
- Neumann P ANPM, Pfender M, Neumann P, et al. Nanoscale nuclear magnetic resonance with chemical resolution. Science. 2017 Jun;357:67–71.
- Zhang T, Pramanik G, Zhang K, et al. Toward quantitative bio-sensing with nitrogen-vacancy center in diamond. ACS Sens. 2021 May.
- Rong X, Lu D, Kong X, et al. Harnessing the power of quantum systems based on spin magnetic resonance: from ensembles to single spins. Adv Phys -X. 2017;2:125–168.
- Zaiser S WGWY, Wang Y, Zaiser S, et al. Quantum error correction in a solid-state hybrid spin register. Nature. 2014 Feb;506:204–207.
- Ying L XRGJSF, et al. Experimental fault-tolerant universal quantum gates with solid-state spins under ambient conditions. Nat Commun. 2015 Nov;6:8748.
- Li Z XKXT, et al. Experimental adiabatic quantum factorization under ambient conditions based on a solid-state single spin system. Phys Rev Lett. 2017 Mar;118:130504.
- Cong W, Bicak J, Kubiznak D, et al. Quantum detection of inertial frame dragging. Phys Rev D. 2021 Jan;103:024027.
- Ju Z, Lin J, Shen S, et al. Preparations and applications of single color centers in the diamond. Adv Phys -X. 2021;6:1858721.
- Shen X, Zhao L-Y, Huang P, et al. Atomic spin and phonon coupling mechanism of nitrogen-vacancy center. Acta Phys Sin. 2021 Mar;70:068501.
- Wood AA, Lilette E, Fein YY, et al. Quantum measurement of a rapidly rotating spin qubit in diamond. Sci Adv. 2018 May;4:eaar7691.
- Wood AA, Hollenberg LCL, Scholten RE, et al. Observation of a quantum phase from classical rotation of a single spin. Phys Rev Lett. 2020;124:020401.
- Wood AA, Lilette E, Fein YY, et al. Magnetic pseudo-fields in a rotating electron-nuclear spin system. Nat Phys. 2017;13:1070–1074.
- Chen X-Y, Yin Z-Q. High-precision gravimeter based on a nano-mechanical resonator hybrid with an electron spin. Opt Express. 2018;26:31577–31588. Nov, 26.
- Gruber A, Dräbenstedt A, Tietz C, et al. Scanning confocal optical microscopy and magnetic resonance on single defect centers. Science. 1997 Jun;276:2012–2014.
- Balasubramanian G, Neumann P, Twitchen D, et al. Ultralong spin coherence time in isotopically engineered diamond. Nat Mater. 2009 May;8:383–387.
- Bar-Gill N, Pham LM, Jarmola A, et al. Solid-state electronic spin coherence time approaching one second. Nat Commun. 2013 Apr;4:1743.
- Maze JR, Stanwix PL, Hodges JS, et al. Nanoscale magnetic sensing with an individual electronic spin in diamond. Nature. 2008 Oct;455:644–647.
- Barry JF, Schloss JM, Bauch E, et al. Sensitivity optimization for NV-diamond magnetometry. Rev Mod Phys. 2020;92:015004.
- Xie T, Zhao Z, Kong X, et al. Beating the standard quantum limit under ambient conditions with solid-state spins. Sci Adv. 2021 Aug;7:eabg9204.
- Dolde F, Fedder H, Doherty MW, et al. Electric-field sensing using single diamond spins. Nat Phys. 2011 Jun;7:459–463.
- Barfuss A, Teissier J, Neu E, et al. Strong mechanical driving of a single electron spin. Nat Phys. 2015 Oct;11:820–U185.
- Sokolov K. Tiny thermometers used in living cells: nanotechnology. Nature. 2013 Aug;500:36–37.
- Kucsko G, Maurer PC, Yao NY, et al. Nanometre-scale thermometry in a living cell. Nature. 2013;500:54–58.
- Wang N, Liu G-Q, Leong W-H, et al. Magnetic criticality enhanced hybrid nanodiamond thermometer under ambient conditions. Phys Rev X. 2018;8:011042.
- Chatzidrosos G, Wickenbrock A, Bougas L, et al. Miniature cavity-enhanced diamond magnetometer. Phys Rev Appl. 2017 Dec;8:044019.
- Liu C-F, Leong W-H, Xia K, et al. Ultra-Sensitive hybrid diamond nanothermometer. Natl Sci Rev. 2021 Jun;8:nwaa194.
- Maclaurin D, Doherty MW, Hollenberg LC, et al. Measurable quantum geometric phase from a rotating single spin. Phys Rev Lett. 2012 Jun;108:240403.
- Ledbetter MP, Jensen K, Fischer R, et al. Gyroscopes based on nitrogen-vacancy centers in diamond. Phys Rev A. 2012 Nov;86:052116.
- Ajoy A, Cappellaro P. Stable three-axis nuclear-spin gyroscope in diamond. Phys Rev A. 2012 May;86:062104.
- Jarmola A, Lourette S, Acosta VM, et al. Demonstration of diamond nuclear spin gyroscope. Sci Adv. 2021 Oct;7:eabl3840.
- Song X, Wang L, Feng F, et al. Nanoscale quantum gyroscope using a single 13C nuclear spin coupled with a nearby NV center in diamond[J]. J Appl Phys. 2018;123:114301.
- Soshenko VV, Bolshedvorskii SV, Rubinas O, et al. Nuclear spin gyroscope based on the nitrogen vacancy center in diamond. Phys Rev Lett. 2021 May;126:197702.
- Niebauer TM, Sasagawa GS, Faller JE, et al. A new generation of absolute gravimeters. Metrologia. 1995 Nov;32:159–180.
- Merlet S, Bodart Q, Malossi N, et al. Comparison between two mobile absolute gravimeters: optical versus atomic interferometers. Metrologia. 2010 Aug;47:L9–L11.
- Litterll KC, Allman BE, Werner SA. Two-wavelength-difference measurement of gravitationally induced quantum interference phases. Phys Rev A. 1997 Sep;56:1767–1780.
- Abele H, Leeb H. Gravitation and quantum interference experiments with neutrons. New J Phys. 2012 May;14:1–5.
- De Angelis M, Bertoldi A, Cacciapuoti L, et al. Precision gravimetry with atomic sensors. Meas Sci Technol. 2009 Feb;20:022001.
- Bodart Q, Merlet S, Malossi N, et al. A cold atom pyramidal gravimeter with a single laser beam. Appl Phys Lett. 2010 Mar;96:849.
- Zhang J, Chen L, Cheng Y, et al. Movable precision gravimeters based on cold atom interferometry. Chin Phys B. 2020 Sep;29:88–96.
- Rademacher M, Millen J, Li YL. Quantum sensing with nanoparticles for gravimetry: when bigger is better. Adv Opt Technol. 2020 Oct;9:227–239.
- Romero-isart O, Juan ML, Quidant R, et al. Toward quantum superposition of living organisms. New J Phys. 2010 Mar;12:033015.
- Castelletto S, Rosa L, Boretti A. Micro-manipulation of nanodiamonds containing NV centers for quantum applications. Diam Relat Mat. 2020 Jun;106:107840.
- Kumar P, Bhattacharya M. Magnetometry via spin-mechanical coupling in levitated optomechanics. Opt Express. 2017 Aug;25:19568–19582.
- Rabl P, Kolkowitz SJ, Koppens FHL, et al. A quantum spin transducer based on nanoelectromechanical resonator arrays. Nat Phys. 2010 Aug;6:602–608.
- Arcizet O, Jacques V, Siria A, et al. A single nitrogen-vacancy defect coupled to a nanomechanical oscillator. Nat Phys. 2011 Nov;7:879–883.
- Rabl P, Cappellaro P, Dutt MVG, et al. Strong magnetic coupling between an electronic spin qubit and a mechanical resonator. Physical Review B. 2009 Jan;79:041302.
- Kolkowitz S, Bleszynski Jayich AC, Unterreithmeier QP, et al. Coherent sensing of a mechanical resonator with a single-spin qubit. Science. 2012 Mar;335:1603–1606.
- Xu ZY, Hu YM, Yang WL, et al. Deterministically entangling distant nitrogen-vacancy centers by a nanomechanical cantilever. Phys Rev A. 2009 Aug;80:2–5.
- Zhou L-G, Wei LF, Gao M, et al. Strong coupling between two distant electronic spins via a nanomechanical resonator. Phys Rev A. 2010 Apr;81:1–5.
- Ranjit G, Cunningham M, Casey K, et al. Zeptonewton force sensing with nanospheres in an optical lattice. Phys Rev A. 2016 May 93;93:053801.
- Monteiro F, Ghosh S, Fine AG, et al. Optical levitation of 10-ng spheres with nano-g acceleration sensitivity. Phys Rev A. 2017 Dec;96:063841.
- Millen J, Monteiro TS, Pettit R, et al. Optomechanics with levitated particles. Rep Prog Phys IOP Publishing. 2020;83:026401.
- Yin Z-Q, Li T, Zhang X, et al. Large quantum superpositions of a levitated nanodiamond through spin-optomechanical coupling. Phys Rev A. 2013 Sep;88:033614.
- Yin Z, Zhao N, Li T. Hybrid opto-mechanical systems with nitrogen-vacancy centers. Sci China Phys Mech Astron. 2015 Feb;58:1–12.
- Simpson JHJ, Fraser JT, Greenwood IAJ. An optically pumped nuclear magnetic resonance gyroscope. IEEE Trans Aerosp. 1963;1:1107–1110.
- Doherty MW, Manson NB, Delaney P, et al. The nitrogen-vacancy colour centre in diamond. Phys Rep. 2013 Jul;528:1–45.
- Bayat K, Choy J, Baroughi MF, et al. Efficient, uniform, and large area microwave magnetic coupling to NV centers in diamond using double split-ring resonators. Nano Lett. 2014 Feb;14:1208–1213.
- Popa I, Gaebel T, Neumann P, et al. Spin polarization in single spin experiments on defects in diamond. Isr J Chem. 2010;46. 4:393–398.
- Jelezko F, Gaebel T, Popa I, et al. Observation of coherent oscillations in a single electron spin. Phys Rev Lett. 2004 Feb;92:076401.
- Stanwix PL, Pham LM, Maze JR, et al. Coherence of nitrogen-vacancy electronic spin ensembles in diamond. Phys Rev B. 2010 Nov;82:201201.1–201201.4.
- Kilin SY, Nizovtsev AP, Maevskaya TM, et al. Spectroscopy on single N-V defect centers in diamond: tunneling of nitrogen atoms into vacancies and fluorescence spectra. J Lumines. 2000 Apr;86:201–206.
- Suter D, Álvarez GA. Colloquium: protecting quantum information against environmental noise. Rev Mod Phys. 2016 Oct;88:041001.
- Degen CL, Reinhard F, Cappellaro P. Quantum sensing. Rev Mod Phys. 2017 Jul;89:035002.
- Zhao N, Ho S-W, Liu R-B. Decoherence and dynamical decoupling control of nitrogen vacancy center electron spins in nuclear spin baths. Phys Rev B. 2012 Mar;85:115303.
- Beha K, Fedder H, Wolfer M, et al. Diamond nanophotonics. Beilstein J Nanotechnol. 2012 Dec;3:895–908.
- Jiang L, Hodges JS, Maze JR, et al. Repetitive readout of a single electronic spin via quantum logic with nuclear spin ancillae. Science. 2009 Oct;326:267–272.
- Steiner M, Neumann P, Beck J, et al. Universal enhancement of the optical readout fidelity of single electron spins at nitrogen-vacancy centers in diamond. Phys Rev B. 2010 Jan;81:035205.
- Jacques V, Neumann P, Beck J, et al. Dynamic polarization of single nuclear spins by optical pumping of nitrogen-vacancy color centers in diamond at room temperature. Phys Rev Lett. 2009 Feb;102:057403.
- Broadway DA, Tetienne J-P, Stacey A, et al. Quantum probe hyperpolarisation of molecular nuclear spins. Nat Commun. 2018 Mar;9:1246.
- Waddington DEJ, Sarracanie M, Zhang H, et al. Nanodiamond-enhanced mri via in situ hyperpolarization. Nat Commun. 2017 Apr;8:15118.
- Ajoy A, Nazaryan R, Liu K, et al. Enhanced dynamic nuclear polarization via swept microwave frequency combs. Proc Nat Acad Sci. 2018 Oct;115:10576–10581.
- Ajoy A, Liu K, Nazaryan R, et al. Orientation-independent room temperature optical 13 C hyperpolarization in powdered diamond. Sci Adv. 2018 May;4:eaar5492.
- Schwartz I, Scheuer J, Tratzmiller B, et al. Robust optical polarization of nuclear spin baths using hamiltonian engineering of nitrogen-vacancy center quantum dynamics. Sci Adv. 2018 Aug;4:eaat8978.
- Van DST, Wang ZH, Blok MS, et al. Decoherence-protected quantum gates for a hybrid solid-state spin register. Nature. 2012 Apr;484:82–86.
- Neumann P, Beck J, Steiner M, et al. Single-shot readout of a single nuclear spin. Science. 2010 Jul;329:542–544.
- Dréau A, Spinicelli P, Maze JR, et al. Single-shot readout of multiple nuclear spin qubits in diamond under ambient conditions. Phys Rev Lett. 2013 Feb;110:60502.
- Ma Y, Hoang TM, Gong M, et al. Proposal for quantum many-body simulation and torsional matter-wave interferometry with a levitated nanodiamond. Phys Rev A. 2017 Aug;96:023827.
- Geiselmann M, Juan ML, Renger J, et al. Three-dimensional optical manipulation of a single electron spin. Nature Nanotech. 2013 Feb;8:175–179.
- Horowitz VR, Alemn BJ, Christle DJ, et al. Electron spin resonance of nitrogen-vacancy centers in optically trapped nanodiamonds. Proc Natl Acad Sci U S A. 2012 Aug;109:13493.
- Hoang TM, Ahn J, Bang J, et al. Electron spin control of optically levitated nanodiamonds in vacuum. Nat Commun. 2016 Jul;7:12250.
- Neukirch LP, von Haartman E, Rosenholm JM, et al. Multi-dimensional single-spin nano-optomechanics with a levitated nanodiamond. Nat Photonics. 2015 Sep;9:653–657.
- Delord T, Nicolas L, Bodini M, et al. Diamonds levitating in a paul trap under vacuum: measurements of laser-induced heating via NV center thermometry. Appl Phys Lett. 2017 Jul;111:013101.
- Pettit RM, Neukirch LP, Zhang Y, et al. Coherent control of a single nitrogen-vacancy center spin in optically levitated nanodiamond. J Opt Soc Am B. 2017 Jun;34:C31–C35.
- Bennett SD, Kolkowitz S, Unterreithmeier QP, et al. Measuring mechanical motion with a single spin. New J Phys. 2012 Dec;14:125004.
- Delord T, Nicolas L, Chassagneux Y, et al. Strong coupling between a single nitrogen-vacancy spin and the rotational mode of diamonds levitating in an ion trap. Phys Rev A. 2017 Dec;96:063810.
- Kowarsky MA, Hollenberg LCL, Martin AM. Non-abelian geometric phase in the diamond nitrogen-vacancy center. Phys Rev A. 2014 Oct;90:042116.
- Scala M, Kim MS, Morley GW, et al. Matter wave interferometry of a levitated thermal nano-oscillator induced and probed by a spin. Phys Rev Lett. 2013 Oct;111:180403.
- Wan C, Scala M, Morley GW, et al. Free nano-object ramsey interferometry for large quantum superpositions. Phys Rev Lett. 2016 Sep;117:14.
- Geraci A, Goldman H. Sensing short range forces with a nanosphere matter-wave interferometer. Phys Rev D. 2015 Sep;92:062002.
- Schreiber KU, Klügel T, Wells JPR, et al. How to detect the chandler and the annual wobble of the earth with a large ring laser gyroscope. Phys Rev Lett. 2011;107:1–4.
- Lai Y-H, Suh M-G, Lu Y-K, et al. Earth rotation measured by a chip-scale ring laser gyroscope. Nat Photonics. 2020;14:345–349.
- Schreiber KU, Wells JPR. Invited review article: large ring lasers for rotation sensing. Rev Sci Instrum. 2013;84:4.
- Li Y, Cao Y, He D, et al. Thermal phase noise in giant interferometric fiber optic gyroscopes. Opt Express. 2019;27:14121.
- Müller T, Gilowski M, Zaiser M, et al. A compact dual atom interferometer gyroscope based on laser-cooled rubidium. Eur Phys J D. 2009;53:273–281.
- Durfee DS, Shaham YK, Kasevich MA. Long-term stability of an area-reversible atom-interferometer sagnac gyroscope. Phys Rev Lett. 2006;97:1–4.
- Fang JC, Qin J, Wan SA, et al. Atomic spin gyroscope based on 129Xe-Cs comagnetometer. Chinese Sci Bull. 2013;58:1512–1515.
- Liu K, Zhang W, Chen W, et al. The development of micro-gyroscope technology. J Micromech Microeng. 2009;19:113001.
- Wang L, Diao W, Song X, et al. Quantum gyroscope based on Berry phase of spins in diamond. 2018; (Feb 2018):88. doi:10.1117/12.2309334
- Zhou X, Wu Y, Xiao D, et al. An investigation on the ring thickness distribution of disk resonator gyroscope with high mechanical sensitivity. Int J Mech Sci. 2016;117:174–181.
- Bose S, Mazumdar A, Morley GW, et al. Spin entanglement witness for quantum gravity. Phys Rev Lett. 2017 Dec;119:240401.
- Barnett SJ. Magnetization by rotation. Science. 1915;42:163–164.
- Dreau A, Lesik M, Rondin L, et al. Avoiding power broadening in optically detected magnetic resonance of single NV defects for enhanced dc magnetic field sensitivity. Phys Rev B. 2011 Nov;84:195204.
- Jensen K, Acosta VM, Jarmola A, et al. Light narrowing of magnetic resonances in ensembles of nitrogen-vacancy centers in diamond. Phys Rev B. 2013 Jan;87:014115.
- Delord T, Nicolas L, Schwab L, et al. Electron spin resonance from NV centers in diamonds levitating in an ion trap. New J Phys. 2017 Mar;19:033031.
- Delord T, Huillery P, Schwab L, et al. Ramsey interferences and spin echoes from electron spins inside a levitating macroscopic particle. Phys Rev Lett. 2018 Jul;121:053602.
- Dobrovitski VV, Feiguin AE, Hanson R, et al. Decay of rabi oscillations by dipolar-coupled dynamical spin environments. Phys Rev Lett. 2009 Jun;102:237601.
- Dobrovitski VV, Feiguin AE, Awschalom DD, et al. Decoherence dynamics of a single spin versus spin ensemble. Phys Rev B. 2008 Jun;77:245212.
- Baibekov EI. Decay of rabi oscillations induced by magnetic dipole interactions in dilute paramagnetic solids. JETP Lett. 2011 May;93:292–297.
- Boscaino R, Gelardi FM, Korb JP. Non-Bloch decay of transient nutations in S=1/2 systems: an experimental investigation. Phys Rev B. 1993 Sep;48:7077.
- Jaskula J-C, Saha K, Ajoy A, et al. Cross-sensor feedback stabilization of an emulated quantum spin gyroscope. Phys Rev Appl. 2019 May;11:054010.
- Romero-isart O, Pflanzer AC, Blaser F, et al. Large quantum superpositions and interference of massive nanometer-sized objects. Phys Rev Lett. 2011 Jul;107:1–4.
- Butts DL. Development of a light force accelerometer. Massachusetts Institute of Technology; 2008.
- Ahn J, Xu Z, Bang J, et al. Optically levitated nanodumbbell torsion balance and ghz nanomechanical rotor. Phys Rev Lett. 2018 Apr;121:33603.
- Delić U, Reisenbauer M, Dare K, et al. Cooling of a levitated nanoparticle to the motional quantum ground state. Science. 2020 Jan;367:892–895.
- Hobbs PCD. Ultrasensitive laser measurements without tears. Appl Optics. 1997 Feb;36:903–920.
- Clevenson H, Trusheim ME, Teale C, et al. Broadband magnetometry and temperature sensing with a light trapping diamond wave guide. Nat Phys. 2015 Apr;11:393–397.
- Frangeskou A, Rahman A, Rahman A, et al. Pure nanodiamonds for levitated optomechanics in vacuum. New J Phys. 2018 Apr;20:043016.
- Hirose M, Cappellaro P. Coherent feedback control of a single qubit in diamond. Nature. 2016 Apr;532:77–80.