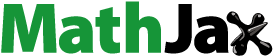
ABSTRACT
Micro/nanogels are unique materials that exhibit the properties of both colloids and hydrogels, i.e. being colloids they exhibit a large specific surface area, while they are hydrophilic and porous allowing them to swell to a great degree with water. Engineering micro/nanogels, through the rational design of various polymer compositions and/or optical structures, can enable them to respond to a myriad of stimuli, e.g. temperature, pH, biomolecules, CO2, light, and electricity. These multi-responsive micro/nanogels and their assemblies, are capable of recognizing and transducing analyte signals into changes in optical properties observable spectroscopically or via the naked eye, allowing their use as optical sensors. In this review, we have highlighted recent state-of-the-art examples of stimuli-responsive micro/nanogel-based systems for optical sensors.
Graphical Abstract
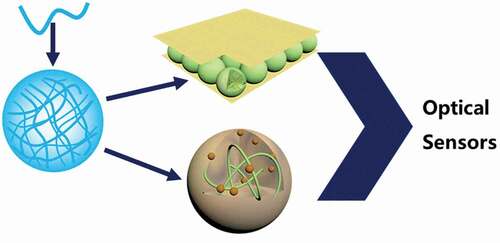
Introduction
The color of objects is a characteristic that can be attributed to the wavelength of light they emit, reflect, and/or absorb [Citation1–3]. Color can be described using three parameters: hue (dominant wavelength), saturation (chromatic purity) and brightness (photon intensity). In everyday life, colors help us identify objects and their environments via different variations and combinations of these three parameters. The various interactions between light and materials have been of considerable interest to society, resulting in significant advances in photophysical technologies such as lighting [Citation4], photography [Citation5], telecommunication [Citation6], imaging [Citation7] and optical instruments [Citation8]. Such interactions can be sensitive enough to track physical and/or chemical changes in the environment, and these interactions can also be used to develop various novel optical sensors [Citation9].
Micro/nanogels are micro-to-nano-sized colloidal particles composed of a crosslinked network of water soluble/swellable polymers, which have found applications in various areas, including encapsulation, tissue engineering, and controlled delivery [Citation10,Citation11]. Generally, micro/nanogels in the water swollen state scatter little light, and their solutions appear transparent. Thus, additional chemistry is required to equip micro/nanogels with the ability to respond to light, and to produce changes in color. Copolymerization or post-polymerization modification of micro/nanogels with chromophores and/or luminophores are the most common approaches to colorize micro/nanogels. The color observed from chromophores is derived from the ‘reflectance’ of complementary wavelengths of visible light being absorbed. In comparison, the color from luminophores stems from emitted photons that are generated by absorption of photon energy, a concomitant energy level transition, and electron relaxation. Looking to colors observed in nature, such as the wings of a butterfly, opal gemstones, and beetles, another widely-studied phenomena, called structural coloration, emerges [Citation12–14]. Unlike chromophores or luminophores, structural color is a result of visible light wavelengths undergoing constructive/destructive interference upon interaction with the ordered/periodic structure of a material. When micro/nanogels are used as the structural features in such materials, the wavelengths of light that are reflected depend on the size of the micro/nanogels, and their center-to-center distance. An interesting feature of micro/nanogels is their ability to be rendered stimuli-responsive, i.e. they can be modified chemically to allow them to change size and/or refractive index in response to specific stimuli. The modification or copolymerization of signal recognition units, such pH-sensitive units, thermosensitive agents, dyes/luminophores, chelating groups, enzymes/DNA, can endow micro/nanogels with desired selectivity. As a result, when micro/nanogels change their size and/or refractive index, the materials reflect different wavelengths of light, and hence exhibit different colors. Specific stimuli include: pH [Citation15,Citation16], light [Citation17,Citation18], temperature [Citation18], metal ions [Citation19], and biomolecules [Citation20].
In this review, stimuli-responsive micro/nanogel-based ‘etalons’ as a specific class of optical sensing devices will be discussed first. Subsequently, the focus will be on micro/nanogels that are modified by, or loaded with, small molecules and nanomaterials. Throughout all the topics addressed in this review, we will highlight the process of analyte recognition and subsequent optical signal transductions.
Etalon-based optical sensors
Photonic materials exhibit optical properties, such as color, as a result of their single- or multi- dimensional structural periodicity. As mentioned above, butterfly wings and opals are colored due to this phenomenon [Citation21]. Fabry-Perot etalons are photonic materials that exhibit characteristic optical properties due to their structure, composed of a dielectric material sandwiched between two thin reflective films/mirrors. Changes in the distance between the two mirrors results in predictable changes to the optical properties, such as the wavelengths of light reflected from the etalons, which can be observed as a color change of the device.
The Serpe Group has generated etalons using poly(N-isopropylacrylamide)-based (pNIPAm) microgels as the dielectric layer [Citation22]. That is, by ‘sandwiching’ a monolithic layer of pNIPAm-based microgels between two thin Au layers/mirrors, devices with tunable optical properties could be constructed () [Citation22]. Incident light entering the optical cavity formed between the two Au mirrors undergoes interference resulting in specific wavelengths of light being reflected that can be observed spectroscopically and/or visually (). The reflected wavelength maxima can be predicted by EquationEquation 1(1)
(1) .
Where is the position of the peak in the reflectance spectrum,
is the peak order,
is the refractive index of the microgel (dielectric) layer,
is the distance between the two Au films and
is the angle of observation. For a given peak order, Equationequation 1
(1)
(1) shows that
is directly proportional to
, i.e. modulation of the distance between the two Au layers can shift
. The optical property changes imparted upon etalons by the reversible solvation state changes of pNIPAm-based microgels in response to external stimuli has been exploited in numerous studies by the group [Citation18,Citation22–29].
Figure 1. (A) Structure of an etalon device with (a, c) two Au-films ‘sandwiching’ a monolithic layer of (b) pNIPAm-based microgel particles on a (d) glass substrate. (B) Characteristic multipeak spectra for etalons assembled with pNIPAm-co-AAc microgels and the visually observable color of the etalons in the (f) hydrated and (k) dry state. Reprinted with permission from ref [Citation23]. Wiley, 2011.
![Figure 1. (A) Structure of an etalon device with (a, c) two Au-films ‘sandwiching’ a monolithic layer of (b) pNIPAm-based microgel particles on a (d) glass substrate. (B) Characteristic multipeak spectra for etalons assembled with pNIPAm-co-AAc microgels and the visually observable color of the etalons in the (f) hydrated and (k) dry state. Reprinted with permission from ref [Citation23]. Wiley, 2011.](/cms/asset/ec5d8336-2f9e-40ba-862f-a02098fee490/tapx_a_2043185_f0001_oc.jpg)
Early publications from the group focused on establishing the basic function of the microgel-based etalons. For example, etalon devices assembled with pNIPAm-based microgels copolymerized with various functional comonomers could render the microgels responsive towards stimuli other than the inherent temperature responsivity of pNIPAm-based microgels [Citation17,Citation30]. Etalon devices assembled with pNIPAm-based microgels copolymerized with acrylic acid (AAc) (pNIPAm-co-AAc) have shown temperature and pH-dependant changes in their optical properties [Citation22,Citation30]. AAc copolymerization also has an added utility as a handle for further chemical modification of microgels, thus expanding the potential applicability of microgel-based etalons for sensing.
In another example, pNIPAm-co-AAc microgels were modified with ferrocene for sensing H2O2 in solution. Sensing H2O2 in biological samples is of great importance due to its function as a regulator of many physiological processes and its role as a by-product in many biological reactions. Etalons assembled using ferrocene-modified microgels showed a reflectance peak blue shift in response to H2O2 due to oxidation of the Fe2+ in ferrocene to Fe3+ and the subsequent interactions between the polar amide groups in pNIPAm and the Fe3+. It was shown that the position of the etalon’s reflectance peak was proportional to the concentration of H2O2 present in the sample and the limit of detection (LOD) was calculated to be around ~40 µM. Given that H2O2 is a by-product of many enzymatic reactions, the utility of this approach as an indirect method for monitoring biological analytes such as glucose was shown using the oxidation of glucose in the presence of glucose oxidase enzyme [Citation31].
Recently, the group reported on the use of microgel-based etalons for the detection of volatile organic compounds (VOCs) in water. VOCs, e.g. tetrahydrofuran (THF), are hazardous when dissolved in water, and can have severe negative impacts on aquatic life [Citation24]. This study demonstrated that etalons assembled with microgels consisting of 95% pNIPAm, crosslinked with 5% N,N’-methylenebis(acrylamide) (BIS), exhibited a reflectance peak blue shift when THF vapor was bubbled through the aqueous solution they were immersed in (). Cononsolvency of pNIPAm-based microgels in THF-water mixtures was proposed as the mechanism of the etalon response. A linear relationship () between reflectance peak shift and the amount of THF bubbled through the experimental setup was obtained with a LOD of 1.27 mM of THF, detected within 4 min. Furthermore, it was shown that the LOD could be improved by modulating the experimental parameters, such as the vapor flow rate and vapor bubble size.
Figure 2. (A) Experimental setup for studying the etalon response to vapour phase VOCs. (B) Absolute total peak shift recorded in response to different volumes of THF being bubbled through the experimental system showing a linear response. Reprinted (adapted) with permission from ref [Citation24]. Copyright 2019, Elsevier.
![Figure 2. (A) Experimental setup for studying the etalon response to vapour phase VOCs. (B) Absolute total peak shift recorded in response to different volumes of THF being bubbled through the experimental system showing a linear response. Reprinted (adapted) with permission from ref [Citation24]. Copyright 2019, Elsevier.](/cms/asset/e914d632-4a6f-478d-9444-08d089486202/tapx_a_2043185_f0002_oc.jpg)
Etalon devices that exhibit responsivity to CO2 dissolved in water were assembled using pyridine-containing microgels [Citation26]. Specifically, when CO2 gas was dissolved in water H2CO3 was formed, which can protonate the microgels’ pyridine groups forming charged pyridinium ions (). The resultant Coulombic repulsion between the polymer chains in the microgels induces swelling, which was observed spectroscopically as a reflectance peak red shift. It was shown that the extent of CO2 response could be modulated by controlling the amount of pyridine groups incorporated into the microgel, with higher pyridine content resulting in a larger overall response and that the etalon response could be reversed by bubbling N2 gas through the reaction solution ().
Figure 3. (a) Schematic diagram depicting the response mechanism of the etalon to CO2. (b) Response of an etalon assembled with microgel containing 60% pNIPAm and 25% 4-vinylpyridine, crosslinked with 5% BIS to repeated CO2 and N2 exposures. Reprinted (adapted) with permission from ref [Citation26]. Copyright 2015, Royal Society Chemistry.
![Figure 3. (a) Schematic diagram depicting the response mechanism of the etalon to CO2. (b) Response of an etalon assembled with microgel containing 60% pNIPAm and 25% 4-vinylpyridine, crosslinked with 5% BIS to repeated CO2 and N2 exposures. Reprinted (adapted) with permission from ref [Citation26]. Copyright 2015, Royal Society Chemistry.](/cms/asset/b2cafa5d-974b-4a21-b4c4-ac0d2494b873/tapx_a_2043185_f0003_oc.jpg)
Biomolecule sensing with pNIPAm-based etalons has also been extensively studied by the Serpe Group. Etalon-based optical sensing systems have been prepared for sensing antibodies [Citation32], steroid hormones such as β-Estradiol (E2) [Citation33] and progesterone (P4) [Citation34], proteins [Citation28] and ssDNA [Citation29]. Recently, the group reported that etalon devices assembled with microgels containing pNIPAm copolymerized with acrylic acid (pNIPAm-co-AAc), and modified with polyclonal anti-P4 antibodies could be used to quantify ng/mL levels of P4 in aqueous solutions. P4 binding to the antibody-modified microgels induced crosslinking in the microgel network, leading to a collapse of the microgel particles which manifests as a reflectance peak blue shift. To demonstrate the selectivity of this approach the sensor was shown to have a low cross reactivity to E2, a common interferent when it comes to P4 detection. This study demonstrated the versatility of this approach by using two different anti-P4 antibodies and showed that the same approach could potentially be used for the detection and quantification of a wide array of antigens, just by using the appropriate antibodies for microgel modification [Citation34].
Furthermore, an optical sensor for detecting picomolar levels of E2 was developed by using an aptamer-modified etalon device assembled from pNIPAm-co-AAc microgels [Citation33]. In this work, disulfide-modified aptamers were reduced to form a thiolated aptamer and subsequently applied on to the top Au layer of the etalon device. Aptamers can be chemically engineered to undergo a change in their conformation from a more linear state to a more compact state upon binding their targets (). The sensing mechanism of this work exploits this change of conformation as a means to block the diffusion of Na+ or Ca2+ ions into the etalon device. Cation diffusion into the microgels can induce a collapse in the microgel network by neutralization of the charged AAc moieties, yielding a spectroscopic blue shift. Therefore, an etalon exposed to a high concentration of E2 would exhibit a smaller blue shift in a given time compared to an etalon exposed to a lower concentration of E2. We hypothesize that this is a direct result of more surface pores being blocked by the aptamer bound to E2 than at low concentration, which allows easier entry of the ions into the microgel layer. Specificity of the device was also investigated by observing the response to P4, the response from 10 ng/mL of P4 was significantly less than that from 5 pg/mL E2, indicating the device was selective towards E2.
Figure 4. Schematic depiction of the sensing mechanism of the aptamer based E2 sensing system showing the effect of E2 binding to the aptamer and the resultant blocking of the surface to cation diffusion into the microgel layer. Reprinted (adapted) with permission from ref [Citation33]. Copyright 2018, Springer.
![Figure 4. Schematic depiction of the sensing mechanism of the aptamer based E2 sensing system showing the effect of E2 binding to the aptamer and the resultant blocking of the surface to cation diffusion into the microgel layer. Reprinted (adapted) with permission from ref [Citation33]. Copyright 2018, Springer.](/cms/asset/a15b4849-c32e-41fc-8c6e-e286256dc818/tapx_a_2043185_f0004_oc.jpg)
In addition to the studies discussed in this section, we have demonstrated that the etalon-based sensing systems are capable of sensing a myriad of other analytes including ethanol in gasoline [Citation25], glucose [Citation27] and polyelectrolytes [Citation35].
Micro/nanogels modified with small molecules
Micro/nanogels can be functionalized with fluorescent molecules for the purpose of optical sensing. Typical fluorophores include: azobenzene [Citation36], spiropyran [Citation37], coumarin [Citation38], and a variety of others [Citation39,Citation40]. The fluorescence of the micro/nanogels can be ‘turned on’ or ‘turned off’ triggered by the physical/chemical interactions between the analytes of interest and the fluorescent probes, and/or the interaction between fluorophores mediated by the solvation state of the micro/nanogels. Consequently, the type and/or concentration of analytes can be easily determined by measuring the change in fluorescence intensity, both visually and/or spectroscopically. So far, micro/nanogel-based fluorescence sensors have been developed for detecting temperature and pH [Citation41,Citation42], metal ions [Citation43,Citation44], strain [Citation42], and reactive oxygen species (ROSs) in vivo [Citation45].
Fluorescent molecules can simply be embedded within the gel matrix by physical entrapment/encapsulation, however leaching of fluorescent molecules from the gel is practically unavoidable. Therefore, fluorescent molecules are typically bound to gels through covalent attachment, effectively solving the leaching problem. One feasible strategy is to modify fluorescent molecules with unsaturated carbon-carbon double bonds [Citation38,Citation46,Citation47]. For example, O’Reilly and coworkers designed aminobromomaleimide (ABM)-labelled microgels where ABM was covalently attached through the copolymerization of ABM methacrylate, with ABM located in the gels’ hydrophobic core [Citation46,Citation47]. While bubbling CO2 gas into solution, microgels were highly swollen, this is due to the protonation of the tertiary amines of poly(N, N,-diethylaminoethyl methacrylate) (pDMAEMA). This increased the microgel hydrophilicity and led to a decrease in fluorescence. After simply purging N2 gas into the solution, the microgels collapsed, and the fluorescence intensity recovered. As a result, ABM-labelled microgels were able to respond to the hydrophilicity/hydrophobicity of the microgels’ core and the size of the microgels. Fluorescent molecules can also be used as crosslinkers in the gel matrix. For example, Zhu et al. reported pNIPAm microgels that were crosslinked with di-chlorinated 1,1’-di[4-(vinyl)benzyl]-4,4’-pyridinium salt (MSPC2+ 2Cl−), which rendered the gels with optical sensing ability. MnO4− anions induced significant fluorescence quenching of the pNIPAm-MSPC microgels. They found that the fluorescent sensor was able to detect trace MnO4− anions in aqueous solutions with a fast response time of 5 min [Citation44].
The abovementioned systems relied on monitoring fluorescence intensity at a single wavelength, however, such a strategy is easily affected by many factors, e.g. environmental noise, and fluctuations in the light source intensity, among other things [Citation48]. As a result, micro/nanogel-based ratiometric fluorescence sensors have been developed. For instance, a colorimetric fluorescent poly(acrylamide-co-N-(3-aminopropyl)methacrylamide) (pAAm-co-APMA) nanogel sensor was reported for detecting X-ray dosages. Under exposure to X-rays, 5(6)-carboxytetramethylrhodamine is stable, and emits red fluorescence (I546). Alternatively, coumarin-3-carboxylic acid, when exposed to X-rays, transitioned to 7-hydroxyl-coumarin-3-carboxylic acid, which emitted blue fluorescence under UV irradiation (I405). Consequently, the dose of X-rays can be determined by measuring the ratio of I405 and I546 [Citation38]. In another example, Yin et al. prepared ratiometric fluorescent K+ sensors that are based on pNIPAm microgels covalently incorporated with K+-recognizing 4-acrylamidobenzo-18-crown-6 residues (B18C6Am), fluorescence resonance energy transfer (FRET) donor dyes (i.e. 4-(2-acryloyloxyethylamino)-7-nitro-2,1,3-benzoxadiazole (NBDAE)), and rhodamine-B-based FRET acceptors (RhBEA) [Citation49]. When the spectra of donor emission and acceptor absorption overlap, and the distance between the donor and acceptor is less than 10 nm, non-radiative energy transfer occurs. Under this condition, excited-state energy is transferred from the donor to the acceptor, resulting in fluorescence from the acceptor. In this case, when the temperature was increased above the lower critical solution temperature (LCST) of pNIPAm, the p(NIPAm-B18C6Am-NBDAE-RhBEA) the microgels collapsed, generating a FRET effect due to the reduced distance between the donor and acceptor. The addition of K+ ions in solution can lead to the reswelling of initially collapsed microgels. This is because the B18C6Am can capture K+ ions, resulting in the enhancement of microgel hydrophilicity and elevated LCST temperatures. Thus, the fluorescence intensity from the acceptor decreased. Using the FRET concept, Zhu et al. synthesized poly(methyl methacryate-co-methacrylic acid-co-(9-phenanthryl)methyl methacrylate-co-(9-anthryl)methacrylate (pMMA-co-MAA-co-Ph-co-An) nanogels for determining solution pH [Citation42]. When pH < pKa of MAA, the protonated -COOH groups cause the nanogels to deswell. Therefore, the donor (Ph) and acceptor (An) are very close to each other, exhibiting enhanced fluorescence signal from the acceptor (An). As pH increased above the pKa of MAA, the -COOH groups were deprotonated, resulting in the swelling of the gels. This process increased the distance between the donor (Ph) and acceptor (An), hence the fluorescence intensity from the donor (Ph) was increased () [Citation42].
Figure 5. Schematic illustration and fluorescence-based sensing of pH-responsive p(MMA-co-MAA-co-Ph-co-An) nanogels. Reprinted (adapted) with permission from ref [Citation42]. Copyright 2017, American Chemical Society (https://pubs.acs.org/doi/abs/10.1021/acsmacrolett.7b00709), further permissions related to this figure must be directed to ACS.
![Figure 5. Schematic illustration and fluorescence-based sensing of pH-responsive p(MMA-co-MAA-co-Ph-co-An) nanogels. Reprinted (adapted) with permission from ref [Citation42]. Copyright 2017, American Chemical Society (https://pubs.acs.org/doi/abs/10.1021/acsmacrolett.7b00709), further permissions related to this figure must be directed to ACS.](/cms/asset/17c9f657-c2cd-4529-8e58-2e1eac75d12e/tapx_a_2043185_f0005_oc.jpg)
Micro/nanogels doped with nanomaterials
Besides the direct modification of micro/nanogels with small molecules to achieve multi-responsive properties for optical sensing, an alternative approach involves the incorporation of nanomaterials into the gels. Owing to the porous structure of micro/nanogels, a variety of nanomaterials, including nanoclusters [Citation50], nanorods [Citation51], nanosheets [Citation52], metallic nanoparticles [Citation53–60] and quantum/carbon dots [Citation61–63], can be successfully embedded into the polymeric matrix via facile methods, without interfering with the transport process between the nanomaterials and the outside environment. The multi-responsive properties can, therefore, be obtained by this unique multi-component structure via the synergistic effect of each component’s stimuli-responsivity in those hybrid systems, i.e. the incorporated nanomaterials can respond to some specific stimuli whereas the polymeric micro/nanogel matrix can be sensitive to other stimuli [Citation63,Citation64]. Beyond achieving multi-stimuli responsivities, providing the embedded nanomaterials with the polymeric micro/nanogel matrix also offers other advantages, such as tunable physicochemical properties, increased stability in the sensing environment, improved biocompatibilities (which is critical in biologically relevant settings and clinical applications), as well as enhanced optical signals, compared to using nanomaterials or micro/nanogels alone. Due to these advantages, over the past few years, nanomaterial-incorporated micro/nanogels have seen an upsurge as optical sensing platforms/devices for various fields [Citation18,Citation33,Citation51,Citation55,Citation62,Citation63]. In this section, some recent examples of multi-stimuli responsive optical sensing strategies using nanomaterial-incorporated micro/nanogels, especially focusing on metallic nanoparticles [Citation56] and carbon dots-incorporated nano/microgels [Citation63] will be discussed.
Metallic nanoparticles, especially silver and gold nanoparticles (AgNPs and AuNPs, respectively), are ideal choices for optical sensing applications [Citation65,Citation66] due to their unique size-dependent optical properties, facile synthetic procedures, and versatility/simplicity of chemical modification. A wide range of optical sensors based on AgNP- or AuNP-incorporated micro/nanogel have been studied and developed over the past few years, including several examples from the Serpe Group [Citation18,Citation53,Citation54]. Even so, the versatility and practicality of such hybrid systems deems them worthwhile of further discussion here. For example, as shown in , Li et al. developed a core-shell structured, multi-stimuli-responsive nanoprobe where AuNPs were contained in nanogels, to achieve in vivo imaging of caspase activity [Citation56]. They first prepared AuNPs covered with Cy5-labeled, caspase-degradable peptide (pep-AuNPs), which resulted in the quenching of fluorescent Cy5 dyes due to the FRET effect between Cy5 and AuNPs. Next, they synthesized AuNP@hydrogel hybrid nanogels by polymerizing a hydrogel shell around the AuNPs that was composed of acid degradable linkers, ionic monomers, and tumor-targeting moieties. Once engulfed by the tumor cells, the polymer portion of the AuNP@hydrogel nanogel could be degraded rapidly in acidic endosomes, leading to the release of pep-AuNPs into the cytoplasm, where caspase-3/7 could cleave the Cy5-labelled peptide, thus freeing Cy5 from AuNPs and increasing fluorescence. The caspase-3/7 activity can, therefore, be related to the intensity of fluorescence. In this approach, the hydrogel shell provided a suitable matrix for AuNPs immobilization, which greatly increased the stability and delivery efficiency by preventing protein corona formation around AuNPs. Overall, this method presented new opportunities for metallic nanoparticle-embedded nanogel based systems for in vivo bio-sensing/imaging applications.
Figure 6. Detection of caspase activity using the AuNP@hydrogel nanogel probes. (a) Schematic diagram of nanogel degradation under acidic (endosome) conditions and subsequent peptide cleavage and Cy5 release by Cas-3/7. (b) Fluorescence signals in various conditions, including or excluding Cas-3/7. (c) Kinetic studies of the fluorescence activation by the nanoprobe under different conditions. Reprinted (adapted) with permission from ref [Citation56]. Copyright 2019, American Chemical Society.
![Figure 6. Detection of caspase activity using the AuNP@hydrogel nanogel probes. (a) Schematic diagram of nanogel degradation under acidic (endosome) conditions and subsequent peptide cleavage and Cy5 release by Cas-3/7. (b) Fluorescence signals in various conditions, including or excluding Cas-3/7. (c) Kinetic studies of the fluorescence activation by the nanoprobe under different conditions. Reprinted (adapted) with permission from ref [Citation56]. Copyright 2019, American Chemical Society.](/cms/asset/84c32e2b-e880-438b-bff7-a1350dca4a1d/tapx_a_2043185_f0006_oc.jpg)
Carbon dots (CDs), which are small carbon nanoparticles possessing fluorescent properties, have many attractive characteristics, such as excellent chemical robustness and inertness, good biocompatibility, environmentally-friendliness, tunable fluorescent properties, as well as simple synthetic procedures [Citation67,Citation68]. Therefore, they have been extensively investigated for building optical sensing platforms, especially for constructing biosensors [Citation63,Citation68]. The combination of CDs and polymeric micro/nanogels can further provide some unique opportunities in these areas, as shown by the study from Li et al [Citation63]. In this example, a temperature sensitive carbon dots/SiO2/molecularly imprinted polymer (CDs/SiO2/MIP) system for cytochrome c (cyt c) sensing was developed. In their work, CDs acted as the fluorescence source; the silanized CDs were the anchors for further molecular imprinting polymerization using NIPAm and MAA as the functional monomers, and N- and C-terminal nonapeptides of cyt c as the templates. The hybrid CDs/SiO2/MIP nanogel exhibited selective cyt c capture, which led to fluorescence quenching of CDs due to the FRET mechanism resulting from the interaction between cyt c and the recognition cavities. The imprinted cavities in the polymer were tested for the selective binding of cty c by competitive binding experiments with other proteins, lysozyme (Lyz) and trypsin (Try). This assay ultimately showed that although Lyz and Try have similar molecular weight to cyt c, they did not match the binding sites and therefore did not effectively quench fluorescence. As demonstrated by the authors (), their sensor was able to detect cyt c in the range of 0.1 to 40 μM with a limit of detection of 89 nM. The selection of NIPAm and MAA as functional monomer units to synthesize the polymeric nanogel network enabled unique advantages from this system. First, the nonapeptide template could be pre-assembled with NIPAm and MAA via double hydrogen bonding effects, thus increasing overall template incorporation efficiency and further target recognition ability/sensitivity. Second, the temperature responsive pNIPAm polymer shell allowed reversible swelling/deswelling of the nanogel in response to temperature, which could translate into a temperature-reversible presentation of the template as well as temperature-reversible binding of the target molecule, making the fluorescent signal respond to temperature as well. In summary, this novel CDs/MIP nanogel sensor broadened the horizons of using CDs and MIP for optical protein sensing applications.
Figure 7. Detection of cyt c using the CDs/SiO2/MIP nanogels. (a) Schematic illustration of nanogel synthesis and reversible binding mechanism. (b) Temperature-response of the fluorescence signal of the nanogel upon exposure to cyt c. (c) Fluorescence emission spectra of nanogels with different concentrations of cyt c added, inset was the plot of (F0/F)-1 against the concentration of cyt c, showing a linear relationship. Reprinted (adapted) with permission from ref [Citation63]. Copyright 2016, Elsevier.
![Figure 7. Detection of cyt c using the CDs/SiO2/MIP nanogels. (a) Schematic illustration of nanogel synthesis and reversible binding mechanism. (b) Temperature-response of the fluorescence signal of the nanogel upon exposure to cyt c. (c) Fluorescence emission spectra of nanogels with different concentrations of cyt c added, inset was the plot of (F0/F)-1 against the concentration of cyt c, showing a linear relationship. Reprinted (adapted) with permission from ref [Citation63]. Copyright 2016, Elsevier.](/cms/asset/a3676af0-3572-402c-90c0-e3ffb6eb4853/tapx_a_2043185_f0007_oc.jpg)
Conclusion and perspectives
As outlined above, the chemical functionality of micro/nanogels can be tailored to achieve responsivity triggered by specific stimuli. Chromophores and luminophores can been attached to the polymeric chains of such micro/nanogels, which allow them to be used for sensing by monitoring changes in electromagnetic field phenomena. While analytes can react directly with fluorophores attached to the micro/nanogels to produce changes in fluorescence, changes in the micro/nanogel solvation state as a result of their interaction with analytes can also produce changes in fluorescence intensity via FRET. Similar to micro/nanogels using either chromophores or luminophores, nanomaterials were immobilized in micro/nanogels to generate specific fluorescent characteristics. In addition, stimuli-responsive micro/nanogels have been employed as elements in photonic devices. The stimuli-responsive behaviors of micro/nanogels can be converted into optical property changes of the photonic material, and likewise employed for sensing. Therefore, the devices bridge the relation between molecular responses and detectable signals.
Although a lot of milestones have been achieved in the field of micro/nanogel research, there are still plenty of interesting areas that need exploration. For example, the colorimetric characteristic of the micro/nanogel-based photonic devices provides a variety of opportunities as wearable sensors. The users could know the concentration of metabolites in body fluids by visually observing color changes and further obtain information about their health. This kind of device can also be used to detect bacteria and other organisms in the wound healing process. This will be beneficial to prevent the occurrence of serious infections. Of course, modification of the technology to detect emerging viruses is of utmost importance. On the other hand, there are still various applications left to study in regard to new stimuli responsive micro/nanogels, such as radiation-responsive microgels. The detection of X/γ-ray in clinical medicine, nuclear power plants, and war zones is still a great challenge today. The radiation-responsive micro/nanogels would find important applications, resolve some global issues, and generate new theory and knowledge, all throughout the research. The hope for this paper is to acquaint readers with new achievements in the field of micro/nanogels and to inspire even more innovative research.
Acknowledgments
M.J.S. acknowledges funding from the University of Alberta (the Department of Chemistry and the Faculty of Science), the Natural Sciences and Engineering Research Council of Canada (NSERC), the Canada Foundation for Innovation (CFI), the Alberta Advanced Education & Technology Small Equipment Grants Program (AET/SEGP). C.F. acknowledges Alberta Innovates Technology Futures for a Graduate Student Scholarship. T.S. acknowledges support from the National Natural Science Foundation of China (No: 21904011, 21890742). M.J.S and T.S. acknowledge the National Natural Science Foundation of China (21750110449). Q.Z. acknowledges funding from the International Cooperation Project of Jilin Province Science and Technology Development Plan (20200801008GH), National Natural Science Foundation of China (No: 21604091), and the National Key Research and Development Program of China (2018YFD1100503). L.H. thanks funding from the National Natural Science Foundation of China (No: 51873137), the Priority Academic Program Development of Jiangsu Higher Education Institutions (PAPD).
Disclosure statement
No potential conflict of interest was reported by the author(s).
Additional information
Funding
References
- Hadjikhani N, Liu AK, Dale AM, et al. Retinotopy and color sensitivity in human visual cortical area V8. Nat Neurosci. 1998;1:235–18.
- Witzel C, Gegenfurtner KR. Color perception: objects, constancy, and categories. Annu Rev Vis Sci. 2018;4:475–499.
- Price TD, Witzel C, Gegenfurtner KR. Sensory drive, color, and color vision. Am Nat. 2017;190:157–170.
- Pimputkar S, Speck JS, DenBaars SP, et al. Prospects for LED lighting. Nat Photonics. 2009;3:180–182.
- Lucieer A, SMd J, Turner D. Mapping landslide displacements using structure from motion (SfM) and image correlation of multi-temporal UAV photography. Prog Phys Geog. 2014;38:97–116.
- Kikuchi K. Fundamentals of coherent optical fiber communications. J Lightwave Technol. 2016;34:157–179.
- Adrian RJ. Particle-imaging techniques for experimental fluid mechanics. Annu Rev Fluid Mech. 1991;23:261–304.
- Leonhardt U. Quantum physics of simple optical instruments. Rep Prog Phys. 2003;66:1207–1249.
- Rai VK. Temperature sensors and optical sensors. Appl Phys B. 2007;88:297–303.
- Soni KS, Desale SS, Bronich TK. Nanogels: an overview of properties, biomedical applications and obstacles to clinical translation. J Control Release. 2016;240:109–126.
- Xu W, Wei M, Serpe MJ. Janus microgels with tunable functionality, polarity, and optical properties. Adv Opt Mater. 2017;5:1600614.
- Hu L, Wan Y, Zhang Q, et al. Harnessing the power of stimuli‐responsive polymers for actuation. Adv Funct Mater. 2019;30:1903471.
- Zhang QM, Serpe MJ. Stimuli-responsive polymers for actuation. Chemphyschem. 2017;18:1451–1465.
- Zhang Q, Serpe MJ, Mugo SM. Stimuli responsive polymer-based 3D optical crystals for sensing. Polymers. 2017;9:436.
- Carter MCD, Sorrell CD, Serpe MJ. Deswelling kinetics of color tunable poly(N-isopropylacrylamide) microgel-based etalons. J Phys Chem B. 2011;115:14359–14368.
- Lee SF, Zhu XM, Wang YX, et al. Ultrasound, pH, and magnetically responsive crown-ether-coated core/shell nanoparticles as drug encapsulation and release systems. ACS Appl Mater Interfaces. 2013;5:1566–1574.
- Zhang QM, Xu W, Serpe MJ. Optical devices constructed from multiresponsive microgels. Angew Chem Int Ed. 2014;53:4827–4831.
- Wei M, Serpe MJ. Temperature-light dual-responsive Au@pNIPAm core-shell microgel-based optical devices. Part Part Syst Char. 2019;36:1800326.
- Heppner IN, Serpe MJ. Poly(N-isopropylacrylamide) microgel-based etalons constructed from various metal layers. Colloid Polym Sci. 2013;291:1557–1562.
- Zhang QM, Berg D, Mugo SM, et al. Lipase-modified pH-responsive microgel-based optical device for triglyceride sensing. Chem Commun. 2015;51:9726–9728.
- Bonifacio LD, Lotsch BV, Puzzo DP, et al. Stacking the nanochemistry deck: structural and compositional diversity in one-dimensional photonic crystals. Adv Mater. 2009;21:1641–1646.
- Sorrell CD, Carter MCD, Serpe MJ. Color tunable poly(N-isopropylacrylamide)-co-acrylic acid microgel-Au hybrid assemblies. Adv Funct Mater. 2011;21:425–433.
- Sorrell CD, Serpe MJ. Reflection order selectivity of color-tunable poly(N-isopropylacrylamide) microgel based etalons. Adv Mater. 2011;23:4088–4092.
- Zhang Y, Carvalho WSP, Fang C, et al. Volatile organic compound vapor detection with responsive microgel-based etalons. Sens Actuators B Chem. 2019;290:520–526.
- Huang H, Serpe MJ. Poly(N-isopropylacrylamide) microgel-based etalons for determining the concentration of ethanol in gasoline. J Appl Polym Sci. 2015;132:42106.
- Zhang QM, Ahiabu A, Gao Y, et al. CO2-switchable poly(N-isopropylacrylamide) microgel-based etalons. J Mater Chem C. 2015;3:495–498.
- Sorrell CD, Serpe MJ. Glucose sensitive poly(N-isopropylacrylamide) microgel based etalons. Anal Bioanal Chem. 2012;402:2385–2393.
- Islam MR, Serpe MJ. Label-free detection of low protein concentration in solution using a novel colorimetric assay. Biosens Bioelectron. 2013;49:133–138.
- Islam MR, Serpe MJ. Polymer-based devices for the label-free detection of DNA in solution: low DNA concentrations yield large signals. Anal Bioanal Chem. 2014;406:4777–4783.
- Hu L, Serpe MJ. Controlling the response of color tunable poly(N-isopropylacrylamide) microgel-based etalons with hysteresis. Chem Commun. 2013;49:2649–2651.
- Zhang QM, Berg D, Duan J, et al. Optical devices constructed from ferrocene-modified microgels for H2O2 sensing. ACS Appl Mater Interfaces. 2016;8:27264–27269.
- Zhang W, Wei M, Carvalho WSP, et al. Enzyme-assisted polymer film degradation-enabled biomolecule sensing with poly(N-isopropylacrylamide)-based optical devices. Anal Chim Acta. 2018;999:139–143.
- Yaxin J, Marcos GC, Michael JS. Poly(n-isopropylacrylamide) microgel-based etalons for the label-free quantitation of estradiol-17β in aqueous solutions and milk samples. Anal Bioanal Chem. 2018;410:4397–4407.
- Jiang Y, Colazo MG, Serpe MJ. Poly(n-isopropylacrylamide) microgel-based sensor for progesterone in aqueous samples. Colloid Polym Sci. 2016;294:1733–1741.
- Islam MR, Serpe MJ. Penetration of polyelectrolytes into charged poly(N-isopropylacrylamide) microgel layers confined between two surfaces. Macromolecules. 2013;46:1599–1606.
- Zhou X, Wu X, He H, et al. Contrast-enhancing fluorescence detection of copper ions by functional fluorescent microgels. Sens Actuators B Chem. 2020;320:128328.
- Zhang QM, Wang W, Y-q S, et al. Biological imaging and sensing with multiresponsive microgels. Chem Mater. 2016;28:259–265.
- Li W, Nie J, Hu R, et al. A nanogel sensor for colorimetric fluorescence measurement of ionizing radiation doses. Chem Commun. 2019;55:9614–9617.
- Denisov SA, Pinaud F, Chambaud M, et al. Saccharide-induced modulation of photoluminescence lifetime in microgels. Phys Chem Chem Phys. 2016;18:16812–16821.
- Yan F, Fan K, Bai Z, et al. Fluorescein applications as fluorescent probes for the detection of analytes. TrAC, Trends Anal Chem. 2017;97:15–35.
- Kim Y, Kim D, Jang G, et al. Fluorescent, stimuli-responsive, crosslinked pNIPAm-based microgel. Sens Actuators B Chem. 2015;207:623–630.
- Zhu M, Lu D, Wu S, et al. Responsive nanogel probe for ratiometric fluorescent sensing of pH and strain in hydrogels. ACS Macro Lett. 2017;6:1245–1250.
- Dong S, Ji W, Ma Z, et al. Thermosensitive fluorescent microgels for selective and sensitive detection of Fe3+ and Mn2+ in aqueous solutions. ACS Appl Polym Mater. 2020;2:3621–3631.
- Zhu Z, Xue J, Wen B, et al. Ultrasensitive and selective detection of MnO4− in aqueous solution with fluorescent microgels. Sens Actuators B Chem. 2019;291:441–450.
- Liu J, Zhang C, Dong J, et al. Nanogel-loading a triarylboron-based AIE fluorophore to achieve ratiometric sensing for hydrogen peroxide and sequential response for pH. New J Chem. 2017;41:4733–4737.
- Robin MP, Raymond JE, O’Reilly RK. One-pot synthesis of super-bright fluorescent nanogel contrast agents containing a dithiomaleimide fluorophore. Mater Horiz. 2015;2:54–59.
- Mabire AB, Robin MP, Quan W-D, et al. Aminomaleimide fluorophores: a simple functional group with bright, solvent dependent emission. Chem Commun. 2015;51:9733–9736.
- Shu T, Wang JX, Lin XF, et al. Dual-emissive gold nanoclusters for label-free and separation-free ratiometric fluorescence sensing of 4-nitrophenol based on the inner filter effect. J Mater Chem C. 2018;6:5033–5038.
- Yin J, Li C, Wang D, et al. FRET-derived ratiometric fluorescent K+ sensors fabricated from thermoresponsive poly(N-isopropylacrylamide) microgels labeled with crown ether moieties. J Phys Chem B. 2010;114:12213–12220.
- Goswami N, Lin FX, Liu YB, et al. Highly luminescent thiolated gold nanoclusters impregnated in nanogel. Chem Mater. 2016;28:4009–4016.
- Huaizhi K, Anna Carolina T, Guizhi Z, et al. Near-infrared light-responsive core-shell nanogels for targeted drug delivery. ACS Nano. 2011;5:5094–5099.
- Cheong AC, Benny R, Insik I, et al. Selective redox-responsive theragnosis nanocarrier for breast tumor cells mediated by MnO2/fluorescent carbon nanogel. Eur J Pharm Sci. 2019;134:256–265.
- Shu T, Shen Q, Wan Y, et al. Silver nanoparticle-loaded microgel-based etalons for H2O2 sensing. RSC Adv. 2018;8:15567–15574.
- Han DM, Zhang QM, Serpe MJ. Poly(N-isopropylacrylamide)-co-(acrylic acid) microgel/ag nanoparticle hybrids for the colorimetric sensing of H2O2. Nanoscale. 2015;7:2784–2789.
- Yecang T, Yi D, Ting W, et al. A turn-on fluorescent probe for Hg2+ detection by using gold nanoparticle-based hybrid microgels. Sens Actuators B Chem. 2016;228:767–773.
- Qiang L, Xinkai Q, Fengchao W, et al. Encapsulating a single nanoprobe in a multifunctional nanogel for high-fidelity imaging of caspase activity in vivo. Anal Chem. 2019;91:13633–13638.
- Wu W, Zhou T, Shen J, et al. Optical detection of glucose by CdS quantum dots immobilized in smart microgels. Chem Commun. 2009;4390–4392. 10.1039/b907348e.
- Wu W, Zhou T, Zhou S. Tunable photoluminescence of Ag nanocrystals in multiple-sensitive hybrid microgels. Chem Mater. 2009;21:2851–2861.
- Wu W, Mitra N, Yan ECY, et al. Multifunctional hybrid nanogel for integration of optical glucose sensing and self-regulated insulin release at physiological pH. ACS Nano. 2010;4:4831–4839.
- Wu W, Shen J, Li Y, et al. Specific glucose-to-SPR signal transduction at physiological pH by molecularly imprinted responsive hybrid microgels. Biomaterials. 2012;33:7115–7125.
- Liu J, Shu T, Su L, et al. Synthesis of poly(N-isopropylacrylamide)-co-(acrylic acid) microgel-entrapped CdS quantum dots and their photocatalytic degradation of an organic dye. RSC Adv. 2018;8:16850–16857.
- Park J, Yi S, Kim HJ, et al. Sunflower-type nanogels carrying a quantum dot nanoprobe for both superior gene delivery efficacy and tracing of human mesenchymal stem cells. Biomaterials. 2016;77:14–25.
- Li DY, Zhang XM, Yan YJ, et al. Thermo-sensitive imprinted polymer embedded carbon dots using epitope approach. Biosens Bioelectron. 2016;79:187–192.
- Molina M, Asadian-Birjand M, Balach J, et al. Stimuli-responsive nanogel composites and their application in nanomedicine. Chem Soc Rev. 2015;44:6161–6186.
- Saha K, Agasti SS, Kim C, et al. Gold nanoparticles in chemical and biological sensing. Chem Rev. 2012;112:2739–2779.
- Lee KS, and El-Sayed MA . Gold and silver nanoparticles in sensing and imaging: sensitivity of plasmon response to size, shape, and metal composition. J Phys Chem B. 2006;110:19220–19225.
- Li L M, Bin Bin C, Chun Mei L, et al. Carbon dots: synthesis, formation mechanism, fluorescence origin and sensing applications. Green Chem. 2019;21:449–471.
- Wang H, Chen Q, Zhou S. Carbon-based hybrid nanogels: a synergistic nanoplatform for combined biosensing, bioimaging, and responsive drug delivery. Chem Soc Rev. 2018;47:4198–4232.