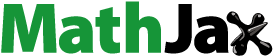
ABSTRACT
The need to measure high repetition rate ultrafast processes cuts across multiple areas of science. The last decade has seen tremendous advances in the development and application of new techniques in this field, as well as many breakthrough achievements analyzing non-repetitive optical phenomena. Several approaches now provide convenient access to single-shot optical waveform characterization, including the dispersive Fourier transform (DFT) and time-lens techniques, which yield real-time ultrafast characterization in the spectral and temporal domains, respectively. These complementary approaches have already proven to be highly successful to gain insight into numerous optical phenomena including the emergence of extreme events and characterizing the complexity of laser evolution dynamics. However, beyond the study of these fundamental processes, real-time measurements have also been driven by particular applications ranging from spectroscopy to velocimetry, while shedding new light in areas spanning ultrafast imaging, metrology or even quantum science. Here, we review a number of landmark results obtained using DFT-based technologies, including several recent advances and key selected applications.
GraphicalAbstract
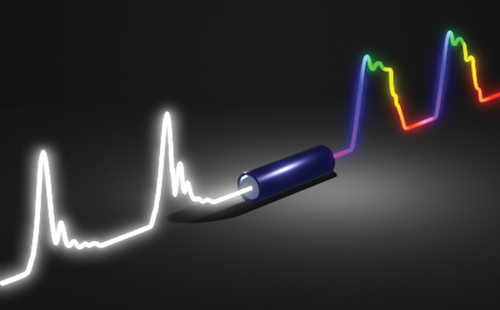
1. Introduction
In photonics, both frequency conversion mechanisms as well as the characterization of ultrashort pulses (or broadband optical signals) constitute the basis of extensive applications. These span advanced light source realization [Citation1–3] (e.g. supercontinuum generation, intense ultrashort laser pulses – Nobel Prize in Physics 2018), metrology [Citation4] (e.g. frequency-comb-based measurements – Nobel Prize in Physics 2005), quantum signal processing [Citation5,Citation6] (e.g. parametric-based two-photon interference) or even demanding imaging techniques (multiphoton microscopy [Citation7], coherent Raman spectroscopy [Citation8], etc.), to name a few. Such ubiquitous frequency conversion and ultrafast phenomena have widespread impact in our daily life, while efficient optical processing tools and architectures have been at the foundation of the most recent technological advances as well as cutting-edge fundamental studies in photonics.
Yet, the improvement of such ultrafast optical measurement tools is intrinsically linked to the quality and the ability to resolve the required wave packet characteristics. In this fast-evolving framework, optical characterization techniques have improved significantly over the last few decades [Citation3], mostly due to specific technological advances (e.g. higher electronic bandwidth of photodetectors and oscilloscopes) along with the deployment of hybrid measurement techniques (e.g. interferometric and optically based gating) for the detection of ultrafast optical dynamics and/or ultrashort events. Importantly, however, these measurement approaches typically rely on either the averaging or the sampling of successive optical signals originating from a periodic pulse train, usually generated with a laser featuring tens of MHz repetition rate. As a consequence, most of these techniques are limited to the characterization of fully coherent, stable or periodic optical waveforms, at the risk of otherwise providing incorrect or artificially averaged signals to the user.
As an example, the field of nonlinear fiber optics [Citation2,Citation9] typically deals with ultrafast trains (> MHz) of short pulses (< 10 ps), whose properties cannot be easily captured in the frequency domain where spectrometers typically have only kHz refresh rates, nor in the time domain where typical <100 GHz detectors possess insufficient bandwidth to resolve the waveform. To overcome these issues, a multitude of techniques based on optical interferometry or pulse gating have been developed over the last decades [Citation3,Citation10]. These include, for instance, well-known ubiquitous auto/cross-correlation measurements (based on e.g. harmonic generation or polarization gating) as well as their frequency or spatially resolved counterparts (such as the FROG, XFROG, sonogram or GRENOUILLE techniques [Citation11–13]). Although widely used for their convenience, price and their potential to access the complex optical field (after signal post-processing or deconvolution), these architectures typically rely on scanning systems yielding measurements that are temporally integrated over successive pulses. While single-shot variations of these techniques are available [Citation14], they require extremely high peak power and are limited in terms of refresh rate, still strongly hampering their applicability. Other related approaches based on direct and sheared interferometry (such as SPIDER or SPIRIT techniques [Citation15–17]) have shown promise for single-shot applications, but these suffer from inherent complexity (by using multiple optical fields for homodyne or heterodyne detection with the need of temporal or frequency synchronization) and the requirement for signal retrieval (via algorithmic processing [Citation18]).
However, many photonic applications based on ultrafast optical signal processing need to access non-repetitive waveforms in a simple and convenient way, yet with highly resolved temporal or spectral properties paired with the capability to access a continuous stream (or extensive data set) of these fluctuating optical properties. It is worth noting that, historically, non-repetitive and real-time measurement techniques stemmed from original research aiming to alleviate the tradeoff between speed and accuracy in analog-to-digital conversion of photonic signals [Citation19], while theoretical studies and early analytical derivation of the DFT can be traced back to the eighties [Citation20,Citation21]. Additionally, dispersive Fourier transform techniques were initially exploited to gain access to the accurate dispersion properties of optical fibers, by performing an average spectral measurement in the temporal domain, i.e. using the technique in reverse with respect to gaining access to spectral statistics with a known dispersion [Citation22]. Following these early developments, the technological advances yielded landmark achievements in the field of nonlinear fiber optics, in particular through the first observations of so-called ‘optical rogue waves’ identified experimentally as statistical anomalies (i.e. ‘rare’ extreme events) in supercontinuum generation [Citation23]. In particular, real-time measurement techniques allowed for the straightforward identification of the system statistics (and its corresponding outliers), while subsequent work further expanded and refined this analysis, for instance, providing the quantification of correlation functions in complex noise-driven nonlinear dynamics [Citation24–26].
While these seminal contributions triggered numerous research efforts in the study of extreme event formation and their associated complex nonlinear dynamics [Citation27] (an aspect further discussed in Section 2.1), it is, however, worth noting that the advances in such ultrafast monitoring were quickly exploited in many other fields where the use of real-time measurements are paramount [Citation28,Citation29]. Indeed, needs for ultrafast detection approaches of fluctuating signals directly arise from monitoring requirements in velocimetry [Citation30,Citation31], spectroscopy [Citation32–37], imaging [Citation38,Citation39] and microscopy [Citation40–44]. Consequently, several key studies have been reported in many research areas directly following the first implementations of time-stretch techniques. In fact, for numerous applications requiring real-time optical detection, a measurement of only the temporal or spectral waveform intensity can prove sufficient, especially if a specific performance gain is achieved by the architecture towards overall quality, simplicity or portability. In this framework, several approaches to ultrafast measurements borrowing concepts from ‘classical’ optics [Citation3,Citation45–49] have been implemented over the last few years (see ), with successful impact beyond their niche study or application in nonlinear photonics.
Figure 1. Schematic illustration of the space-time duality in optics: The equivalence between the diffraction (spatial domain) and dispersion (temporal domain) phenomena summarizes the physical concepts underlying the frequency-to-time mapping approach used in the ‘Dispersive Fourier transform’ technique as well as its implementation for the realization of so-called ‘Time-lens’ measurements. (a) Diffraction of light through a circular aperture. (b) Convergent lens and the construction of an enlarged image. (c) Principle of the dispersive Fourier transform technique, allowing to readily map the broadband spectrum of a pulsed light source in the temporal domain. (d) Principle of the time lens, allowing for the magnification of the temporal optical waveform by the concatenation of two dispersive elements separated by an element used to apply a quadratic temporal phase on the optical waveform (i.e. a lens in the temporal domain).
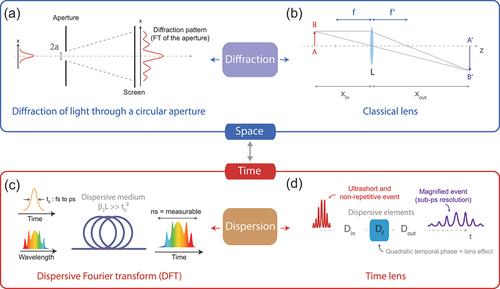
In particular, frequency domain measurements such as the dispersive Fourier transform (DFT), based on a well-known phenomenon in diffractive optics ()), have received considerable attention from the community for their simplicity and broad applicability. Simply put, the dispersion of a highly dispersive fiber can be leveraged during purely linear propagation of an optical waveform so as to retrieve the equivalent spectrum in the time domain by exploiting the different delays, respectively acquired by various spectral components (see )). Similarly, in the temporal domain, techniques such as time lens (or variations such as time microscopy) exploit similar dispersive features to enable controllable magnification of the ultrashort optical signal so that it can be readily recorded with fast (> GHz) detection (see )).
The DFT technique has become a widespread characterization tool because of its intrinsic simplicity in allowing straightforward (direct) spectroscopic intensity measurements in real-time. As illustrated in , frequency-to-time mapping is achieved by time-stretching the broadband pulses through group-velocity dispersion and, as a consequence, the output pulses in the time domain then mimic their input spectrum. In the far-field approximation, the mapping between time T and frequency ω is given by
Figure 2. Principle of DFT measurements. (a) A broadband optical signal is temporally stretched after linear propagation in a highly dispersive fiber. The various spectral components of the optical signal are mapped in the temporal domain so that the optical spectrum can be directly retrieved from the pulse temporal intensity waveform. (b) For the retrieval and analysis of non-repetitive events, real-time spectra from different pulses can be directly acquired from ultrafast optoelectronic measurements (e.g. using a > GHz photodiode + oscilloscope detection system) with the ability to readily analyze shot-to-shot fluctuations from successive traces. In this case, the equivalent DFT spectral resolution is related to the bandwidth of the detection system as well as the magnitude of DFT time-stretch. The achievable resolution is thus a tradeoff between the digitizer sampling rate, the spectral bandwidth of the broadband optical signal and the pulse train repetition rate (to avoid any temporal overlap between adjacent time-stretched spectra).
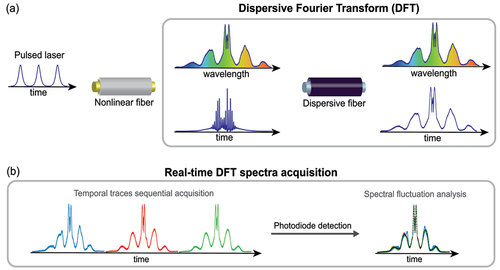
where βm are the mth order dispersion coefficients, z is the propagation distance and f0 = ω0 /2π is the central pulse frequency. When the higher dispersion orders are negligible, this equation then writes as It is worth noting that EquationEquation 1
(1)
(1) is only valid if the so-called temporal Fraunhofer approximation, analogous to the far field condition in the spatial domain (see )), is reached. In the diffraction case, this condition is expressed as d ≫2a2/λ, where a is the aperture radius and d is the distance between the aperture and the observation plane. Here, in the temporal domain, the equivalent condition is written as t02 ≪ |2β2z|, where t0 is the pulse duration.
Without loss of generality, it is worth noting that other approaches of space-time duality such as fractional or self-imaging (e.g. Talbot) effects or those based on near field (Fresnel) diffraction analogous have also been demonstrated [Citation50–54]. However, we limit this review to the DFT cases relying on such a temporal Fraunhofer approximation (see, e.g., Ref [Citation55]. for a theoretical description of the DFT principle). In this latter case, the spectral resolution δλ of DFT is then limited by three main factors: accumulated dispersion (intrinsically bounded by the repetition rate and spectral extension of the optical signal to be measured), photodetector bandwidth and oscilloscope sampling rate. It is then expressed as
δλdis is the resolution imposed by the dispersion process and is expressed as , with D being the dispersion parameter. δλph and δλosc are the resolutions enacted by the photodetector and oscilloscope, respectively, and written as
, where fph and fosc are the photodetector and oscilloscope bandwidths, respectively.
When these conditions are respected, in order to avoid any temporal overlap between successive optical signals, continuous single-shot spectral measurements can thereby be obtained simply using a photodetector and an oscilloscope with sufficiently high bandwidths, as illustrated in ).
Using this approach, landmark studies have been reported over the last decade, spanning characterization of modulation instability processes and supercontinuum formation as well as more advanced characterization of laser mode-locking build-up and optical parametric oscillation dynamics (see the Results section). Even beyond the fundamental study of complexity and nonlinear dynamics in optical systems, the capture of fast non-repetitive events in photonics has seen remarkable success in the last 15 years and many of these results have already been summarized [Citation28,Citation29].
In this article, we focus on four key applications. In particular, we discuss updated implementations of real-time-measurement techniques to address key aspects of nonlinear fiber propagation, and also novel DFT approaches to study dynamical evolution in fiber lasers and microresonators, with direct impact on several aspects of metrology. We also provide recent examples of the use of DFT for imaging ultrafast phenomena as well as underlining the potential of DFT approaches for the characterization and control of quantum photonics systems. Finally, we conclude with a discussion of the impact of DFT within ‘smart’ photonics and machine learning.
2. Results
2.1 Measurement of non-repetitive events in nonlinear fiber optics
Although many applications of photonics directly benefited from DFT and real-time measurement techniques, many efforts in this research direction were oriented towards the measurement, study and analysis of nonlinear fiber propagation dynamics. For instance, following the experimental discovery of optical rogue waves [Citation23] (initially obtained by supercontinuum selective spectral filtering), DFT techniques have been implemented to directly access single-shot fluctuations over the whole supercontinuum as seen in . This approach yielded straightforward identification of the system statistics [Citation56] and its corresponding outliers across the complete broadband spectrum (even leveraging the full dynamic of the digitizer from adaptive scaling) [Citation24]. The statistics of such a fully extended (broadband) supercontinuum are visible in ).
Figure 3. Typical DFT results for the real-time characterization and statistical analysis of spectral fluctuations in nonlinear fiber optics. The DFT technique, via the setup shown in (a), can be used to study a variety of dynamics highly sensitive to noise fluctuations, from a fully developed broadband incoherent supercontinuum (top) to, e.g., the onset phase of nonlinear propagation where spontaneous modulation instability takes place (bottom): (b) DFT experimental characterization of an extended incoherent supercontinuum, exhibiting important shot-to-shot spectral fluctuations. (c,d) The spectral intensity histograms reconstructed at different wavelengths exhibit very different distributions and illustrate how particular spectral regions are prone to the appearance of statistically rare ‘extreme’ events. (e) Spectral fluctuations of MI sidebands sequentially recorded by DFT. The statistical analysis of these fluctuations can be leveraged to reconstruct a map of spectral correlation (f) that can be directly compared to the same Pearson correlation maps obtained from stochastic Monte-Carlo simulations (g). Adapted from Ref. [Citation24].
![Figure 3. Typical DFT results for the real-time characterization and statistical analysis of spectral fluctuations in nonlinear fiber optics. The DFT technique, via the setup shown in (a), can be used to study a variety of dynamics highly sensitive to noise fluctuations, from a fully developed broadband incoherent supercontinuum (top) to, e.g., the onset phase of nonlinear propagation where spontaneous modulation instability takes place (bottom): (b) DFT experimental characterization of an extended incoherent supercontinuum, exhibiting important shot-to-shot spectral fluctuations. (c,d) The spectral intensity histograms reconstructed at different wavelengths exhibit very different distributions and illustrate how particular spectral regions are prone to the appearance of statistically rare ‘extreme’ events. (e) Spectral fluctuations of MI sidebands sequentially recorded by DFT. The statistical analysis of these fluctuations can be leveraged to reconstruct a map of spectral correlation (f) that can be directly compared to the same Pearson correlation maps obtained from stochastic Monte-Carlo simulations (g). Adapted from Ref. [Citation24].](/cms/asset/df1582bb-98d8-41bb-88b1-4c371473cb39/tapx_a_2067487_f0003_oc.jpg)
In addition to this more complete statistical characterization, DFT measurements also provided new insights into extreme event formation during the onset phase of nonlinear pulse propagation in fibers associated with modulation instability (MI) dynamics [Citation27]. In fact, this spectral broadening phase is highly sensitive to the initial conditions of the system, and DFT can provide a clear quantification of e.g. correlation functions in the complex noise-driven dynamics [Citation24,Citation26,Citation57]. Depending on the DFT and correlation post-processing involved, one can, for instance, easily retrieve spectrally resolved correlation maps as those illustrated in ). Moreover, one can leverage DFT monitoring to assess the impact of external factors (such as optical seeding, pump chirp, fiber design, laser properties [Citation26,Citation58–61]) on the system spectral stability.
Improvements on DFT techniques
Interestingly, these initial studies on MI and extreme event formation have subsequently been a driving force in a broader refinement and optimization of real-time measurement techniques [Citation25,Citation29]. For instance, improvements on DFT measurements have been reported for the characterization and analysis of octave-spanning spectra [Citation62], as illustrated in . In this case, DFT was implemented at another central wavelength (i.e. a region outside of the conventional telecom band where dispersion-compensating fiber is optimized and widely available) and with an extended bandwidth for which approximating the DFT as a result of group velocity dispersion only was not valid anymore. In this regime, the inclusion of a third-order dispersive term to the DFT analysis (as well as the inclusion of detector spectral sensitivity) needed to be taken into consideration for an efficient spectral reconstruction and a valid statistical analysis – see .
Figure 4. Example of DFT implementation for the experimental analysis of octave-spanning supercontinuum: (a) Experimental setup. (b) Single shot spectra obtained experimentally by DFT measurements. (c) Superimposed single shot (grey dashes) and mean (black line) DFT spectra obtained from real-time DFT measurement and compared to optical spectral analyzer ‘averaged’ measurement (red dashes). (d) Octave spanning spectral correlation map (via Pearson coefficient) reconstructed form experimental DFT shot-to-shot spectral fluctuation analysis. Adapted from Ref. [Citation62].
![Figure 4. Example of DFT implementation for the experimental analysis of octave-spanning supercontinuum: (a) Experimental setup. (b) Single shot spectra obtained experimentally by DFT measurements. (c) Superimposed single shot (grey dashes) and mean (black line) DFT spectra obtained from real-time DFT measurement and compared to optical spectral analyzer ‘averaged’ measurement (red dashes). (d) Octave spanning spectral correlation map (via Pearson coefficient) reconstructed form experimental DFT shot-to-shot spectral fluctuation analysis. Adapted from Ref. [Citation62].](/cms/asset/3db24d8b-f9fe-47e7-8c4c-b064b0ef34d4/tapx_a_2067487_f0004_oc.jpg)
In a similar framework, several examples of DFT implementations can provide significant spectral time-stretching, leading to a drastic reduction of the measurable power level and thus required an active amplification scheme to compensate the overall DFT system loss (and DFT-induced dispersion of the input signal power) [Citation29,Citation32]. Other advanced ultrafast optical processing techniques based on DFT analogues and relying on fractional effects including, e.g., Talbot effect [Citation63,Citation64] or anamorphic time-stretch [Citation33,Citation65,Citation66] have also been implemented, either considering the spectral or temporal domain. These are, however, paired with significant complexity or constrained to specific applicative fields.
In fact, the simplicity of DFT has made this technique mainstream for the advanced spectral monitoring of incoherent optical signals. However, one of the main drawbacks of this technique is linked with the requirement of ultrafast oscilloscopes and the limited bit depth of their ADCs (8 or 12 bits at best – see, for instance, Ref [Citation29]. for a detailed discussion on noise and ultrafast digitizers ENOBs). This corresponds typically to a dynamic range around 30 dB, which may in fact prove insufficient for particular applications. For instance, machine learning analysis of the spectral data set has been demonstrated to predict the emergence of extreme events via DFT measurements [Citation67], but required higher dynamic ranges than those available from conventional DFT schemes (>50 dB), reached in this case by means of mechanical scans and 2D detectors.
Accessing the time domain: Time-lens & alternatives
DFT techniques are extremely powerful in monitoring broadband spectral fluctuations, but unfortunately they neither allow for a direct nor an indirect access to the temporal structure of ultrashort optical pulses. A typical example is the case for the randomly localized breather structures emerging from MI during nonlinear fiber propagation (as illustrated in ) – with durations in the picosecond regime – which cannot be readily captured by conventional electronic systems.
Figure 5. Sea of random picosecond pulses, as typically generated via modulation instability during nonlinear fiber propagation (results obtained from numerical GNLSE simulations [Citation68]). Resolving such non-repetitive pulses would theoretically require a direct detection with a bandwidth close to THz values, a figure out of the range of current electronic detectors.
![Figure 5. Sea of random picosecond pulses, as typically generated via modulation instability during nonlinear fiber propagation (results obtained from numerical GNLSE simulations [Citation68]). Resolving such non-repetitive pulses would theoretically require a direct detection with a bandwidth close to THz values, a figure out of the range of current electronic detectors.](/cms/asset/e8fea1b2-6788-45f8-84b4-9d42027af483/tapx_a_2067487_f0005_oc.jpg)
For well-defined physical problems, this access to the spectral domain only may not be a stringent limitation, and these hurdles may even be circumvented by a priori knowledge of the dynamics at play or by the use of machine-learning to gain insight in the temporal properties of the optical waveform [Citation67,Citation69]. However, for many studies, accessing the time domain in real-time and with sub-picosecond resolution is of paramount importance. For instance, the prediction of analytical-like structures such as solitons on finite background from noise-driven MI [Citation70] or the formation of Riemann waves as shock-wave prototypes [Citation71,Citation72] directly benefited from experimental real-time measurement techniques in the temporal domain [Citation73–75].
In fact, while specific implementations of non-repetitive measurements have been developed over the years, such as ‘time microscopy’ [Citation74], most of these systems such as the ‘time-lens’ (TL) essentially rely on the space-time duality [Citation76] and time-stretch techniques [Citation19,Citation28,Citation29]. With compact on-chip implementations relying on e.g. four-wave mixing [Citation77–80], time lens systems are now commercially available, making them compatible with a wide range of applications. A typical example is shown in , where time-lens measurements give a 76 times magnification of randomly generated picosecond ‘breathers,’ allowing their straightforward measurement with a 30 GHz bandwidth detection system [Citation73].
Figure 6. Schematic of an experimental setup used for the characterization of random picosecond pulses (breathers) as generated after nonlinear fiber propagation of a noisy continuous wave input (see ). The time lens uses two dispersive propagation steps (D1 and D2), one on each side of an element that applies a quadratic temporal phase. In this experiment, the quadratic phase was applied from four wave mixing (FWM) with a linearly chirped pump pulse co-propagating in a silicon waveguide. The 90 ps chirped pump pulses are generated from a femtosecond pulse subsequently stretched through propagation in a fiber of dispersion Dp. The wavelength-converted idler after FWM (with applied quadratic phase) is filtered before further propagation in segment D2. The overall temporal magnification is 76.4 such that the temporally stretched replica can be resolved using a 30 GHz bandwidth detection system. Adapted from Ref. [Citation73].
![Figure 6. Schematic of an experimental setup used for the characterization of random picosecond pulses (breathers) as generated after nonlinear fiber propagation of a noisy continuous wave input (see Figure 5). The time lens uses two dispersive propagation steps (D1 and D2), one on each side of an element that applies a quadratic temporal phase. In this experiment, the quadratic phase was applied from four wave mixing (FWM) with a linearly chirped pump pulse co-propagating in a silicon waveguide. The 90 ps chirped pump pulses are generated from a femtosecond pulse subsequently stretched through propagation in a fiber of dispersion Dp. The wavelength-converted idler after FWM (with applied quadratic phase) is filtered before further propagation in segment D2. The overall temporal magnification is 76.4 such that the temporally stretched replica can be resolved using a 30 GHz bandwidth detection system. Adapted from Ref. [Citation73].](/cms/asset/6ceb1413-c16b-445d-bd7d-acbe1bd71363/tapx_a_2067487_f0006_oc.jpg)
Importantly, it is worth noting that DFT and TL approaches are complementary and have already proved to be highly successful to gain insight into not only numerous physical phenomena such as nonlinear frequency conversion processes but also in other optical cavity systems for which TL measurements helped gain important insights into complex dynamics [Citation81,Citation82], as further discussed in the next section. Finally, we would like to emphasize that temporal imaging systems, based on peculiar space-time duality implementations, are still at the forefront of current research. Recent results include the demonstration of single-shot and full-field measurements via heterodyne time lens arrangements (such as the SEAHORSE technique, allowing complex field reconstruction with excellent <100 fs temporal resolution) [Citation83–85] or the denoising and real-time capture of ultrafast noisy optical waveforms by leveraging a suitably tailored spectral Talbot effect [Citation86].
2.2 Time-stretch techniques applied for the characterization of laser and optical cavities dynamics
Study of fiber laser oscillations build-up and mode-locking dynamics
It is well known from early studies on laser oscillators that such systems exhibit intriguing dynamics, including periodic attractors, chaotic behaviors and a myriad of nonlinear effects. In the last year, the emergence of DFT associated with the performances of recent fast digitizers has allowed the photonics community to take another look at the transient dynamics at stake in ultrafast laser, with the aim of fully characterizing the processes involved in the birth, evolution and stabilization of ultrashort pulses. In particular, mode-locked (ML) lasers have been the ideal playground to leverage the high-throughput capabilities of DFT for recording shot-to-shot spectra on thousands of cavity roundtrips, from laser build-up to steady-state. Indeed, mode-locked oscillators are now the work-horses of many optical technologies and applications, such as multiphoton microscopy, distance measurements and frequency metrology (frequency combs), as well as nonlinear frequency conversion or THz sources. Therefore, understanding their intrinsic dynamics is indeed of particular importance.
The pulse-resolved spectral evolution of femtosecond pulse trains, from the initial fluctuations to transient interference dynamics has for instance been studied in the common Kerr-lens ML Ti:Sapphire laser using DFT [Citation87]. ML fiber lasers are, however, even more adapted to DFT measurements, and many efforts have been recently devoted to capturing the instabilities and behaviors of their various mode-locking regimes. Among them, the real-time characterization of dissipative solitons (i.e. with nonlinear gain and losses) has attracted much interest as the soliton’s stability against perturbation but also the interaction between individual solitons (bound states) have much in common with similar phenomena in other areas of physics. The booting dynamics and dissipative soliton formation in ML fiber lasers have then been thoroughly investigated [Citation88,Citation89] and the unstable dynamics of specific systems [Citation90], such as the soliton-similariton configuration [Citation91], have been unveiled. The latter was for instance studied with the setup shown in ), and a typical dynamical evolution is depicted in ) where DFT has been used to efficiently track the transition from a noisy regime to an explosion regime and eventually to the steady-state. Interestingly, following the Wiener-Khinchin theorem, one may also retrieve the field autocorrelation from the Fourier transform of these DFT spectra, illustrated in ), thus allowing us to study in detail the evolution (e.g. temporal separation and phase) within multiple or complex pulse states [Citation91,Citation92]. In fact, exotic dynamics, such as noise-like pulses [Citation93] or soliton explosion [Citation94] have also been observed in ultrafast fiber lasers. Among such fascinating effects, the concept of bound state formation has attracted much attention. Such a phenomenon appears when multiple dissipative solitons coherently interact or assemble to form a ‘soliton molecule,’ where the molecular analogy is suggested by the particle-like nature of the solitons, and the presence of a strong binding mechanism [Citation95]. The dynamical features of such soliton molecules have been characterized using DFT-based spectral interferometry [Citation92,Citation96–98] and novel self-localized structures, such as breathing dissipative solitons [Citation99] or vector dynamics [Citation88,Citation100], have been identified.
Figure 7. Examples of fiber laser evolution dynamics characterized via DFT analysis over hundreds of cavity roundtrips. (a) Experimental setup for the study of a fiber laser exhibiting soliton-similariton dynamics via DFT. (b) Single pulse mode-locking obtained after a transition from a noisy operation and an explosion regime (white dashed box), with an abrupt spectral broadening clearly captured by DFT measurements. (c) DFT measurements can be used to study the dynamical evolution of a soliton molecular state. The spectra evolution can be used to retrieve autocorrelations of the field, stemming from a ‘molecule state’ with a periodically oscillating phase. Panels (a-c) are adapted from [Citation91]. (d) Experimental observation of the Sagnac effect in a bidirectional fiber laser, illustrated by a change in the tilted modulation pattern within the DFT spectra. Adapted from [Citation103].
![Figure 7. Examples of fiber laser evolution dynamics characterized via DFT analysis over hundreds of cavity roundtrips. (a) Experimental setup for the study of a fiber laser exhibiting soliton-similariton dynamics via DFT. (b) Single pulse mode-locking obtained after a transition from a noisy operation and an explosion regime (white dashed box), with an abrupt spectral broadening clearly captured by DFT measurements. (c) DFT measurements can be used to study the dynamical evolution of a soliton molecular state. The spectra evolution can be used to retrieve autocorrelations of the field, stemming from a ‘molecule state’ with a periodically oscillating phase. Panels (a-c) are adapted from [Citation91]. (d) Experimental observation of the Sagnac effect in a bidirectional fiber laser, illustrated by a change in the tilted modulation pattern within the DFT spectra. Adapted from [Citation103].](/cms/asset/e37e2f7e-a7c2-48ba-9076-9790b1643223/tapx_a_2067487_f0007_oc.jpg)
Interestingly, DFT can be used to study and track the binding properties of these soliton molecules, where the phase evolution between localized optical structures can be readily obtained by DFT-based spectral interferometry [Citation96]. DFT has further proved its utility to probe and to allow the control of such bound states [Citation101] but also to track complex break-up and collision dynamics together with time-lens measurements [Citation82]. In addition to such soliton dynamics, DFT has also been used to observe other intriguing phenomena in laser oscillators, such as extreme events [Citation90,Citation102] or more recently the optical Sagnac effect [Citation103]. The latter is highlighted in ), where it is manifested as a specific modulation pattern on the DFT spectra.
Tracking OPO dynamics and spectral correlations with DFT and mutual information analysis
As highlighted previously, DFT is an efficient tool for capturing non-repetitive events on relatively extended time ranges and to generate large data ensembles, then enabling the use of various statistical tools to extract relevant information about the system’s dynamics. For instance, we have seen in section 2.1 that Pearson correlations or higher-order moments can be used to unveil the dynamics of complex and/or nonlinear phenomena such as supercontinuum generation [Citation24,Citation62], modulation instability [Citation26,Citation57], four-wave mixing [Citation61] or even random lasing [Citation60]. The study of wavelength intensity correlations is then very insightful as it allows to reveal energy transfers or dependency between different spectral regions. Pearson correlation, however, faces a limitation as it can only evidence the linear dependency between two variables and give information about the strength and nature (negative or positive) of the linearity. In order to obtain improved physical insights, it has been shown that mutual information analysis (MIA) could replace Pearson correlations as it rather quantifies the general dependency between two variables (either linear or nonlinear). Despite such a remarkable ability, MIA has been hardly used in nonlinear optics, but has already proved useful to track the spectral dynamics of fiber lasers [Citation60,Citation88]. The mutual information between two variables X and Y is given by:
where PXY(x, y), PX(x) and PY(y) are the joint probability mass function and marginal mass functions of X and Y, respectively.
At the frontier between laser oscillators and nonlinear optics, it is well-known that optical parametric oscillators exhibit rich spectro-temporal dynamics and particular wavelength correlations, and their study can then prove relevant in the frame of several quantum optics applications (see below). However, DFT-based studies on optical parametric oscillators have been mostly limited to (2)-based bulk OPOs [Citation104,Citation105]. On the other hand, fiber optical parametric oscillators (FOPOs) based on degenerate four-wave mixing have been little studied to date. DFT has been used recently on a FOPO [Citation106] but without investigating spectral correlations. Consequently, a recent work by Touil et al. [Citation107] leveraged the simplicity of DFT together with mutual information analysis to track energy transfers in a picosecond FOPO, as depicted in ). In this work, the nonlinear medium (a small core diameter fiber) is pumped in the normal dispersion regime at 1550 nm, and an optical delay line is set to synchronize the resonating signal (around 1490 nm) or idler (around 1650 nm) with the following pump pulses and to tune the emission wavelengths. After acquiring thousands of single-shot spectra, MIA has been combined with least square (LSQ) regression in order to infer the sign and magnitude of the dependency between the spectral regions of interest (residual pump, signal, idler) and to efficiently track the FOPO dynamics and conversion efficiency.
Figure 8. Capturing FOPO dynamics using DFT. (a) Experimental setup of the fiber optical parametric oscillator under study. C: couplers, CIRC: optical circulator, DSF, dispersion shifted fiber, EDFA: erbium-doped fiber amplifier. (b) Typical MI-LSQ map constructed from ~2000 consecutive shot-to-shot spectra. (c) Specific dynamical behaviour obtained when increasing the pump intensity fluctuations. Reproduced from Ref. [Citation107].
![Figure 8. Capturing FOPO dynamics using DFT. (a) Experimental setup of the fiber optical parametric oscillator under study. C: couplers, CIRC: optical circulator, DSF, dispersion shifted fiber, EDFA: erbium-doped fiber amplifier. (b) Typical MI-LSQ map constructed from ~2000 consecutive shot-to-shot spectra. (c) Specific dynamical behaviour obtained when increasing the pump intensity fluctuations. Reproduced from Ref. [Citation107].](/cms/asset/60573e7d-73ae-47d1-8461-4fbc5120065e/tapx_a_2067487_f0008_oc.jpg)
An example of the MI-LSQ maps generated in this experiment is shown in ), which fully represents the conversion efficiency (i.e. the phase-matching state) between pump and idler waves on a single wavelength basis but also the effect of pump fluctuations on this conversion efficiency (magnitude of the coefficient). Interestingly, this study showed that when increasing the pump intensity fluctuations above a given level, the FOPO exhibited a specific behaviour where the conversion efficiency oscillated between a high-efficiency state with strong pump depletion (state 1) and a low-efficiency state (state 2), as represented in ). In addition, plotting MI-LSQ maps in that case remarkably showed that the idler’s pump-induced fluctuations increased by a factor higher than 60 and are 20 times greater than those of the pump itself. In addition, MI-LSQ allowed to evidence that the spectral correlations could be shaped to a certain extent, which could be useful for quantum optics applications. Using DFT-based MIA then appears as a very simple and efficient tool to study the dynamics of nonlinear systems and could prove superior to standard correlations in a lot of cases involving nonlinear dependencies.
Characterization of frequency comb dynamics via DFT
DFT-diagnosis is intrinsically limited by the repetition rate of the optical signal studied. Indeed, most of the time, DFT is achieved through an optical fiber in which the ultrashort pulses temporally stretch by a factor of more than a million. Studying high repetition laser sources is consequently not directly doable as the stretched pulses would temporally overlap in the dispersive fiber, causing a loss of information. Some techniques based on compressive sensing and consisting in applying a random binary mask to the pulses and then recovering them numerically have been implemented [Citation42] to overcome this limitation, but they remain costly and require a lot of postprocessing. This limitation results in DFT being used for the most part on MHz sources as seen in previous sections, omitting trending GHz sources such as frequency combs generated by microresonators (also called microcombs).
Laser cavity solitons in microcavities nested in a fiber loop have recently emerged as a highly efficient, self-recovery approach for generating optical microcombs. In such microcombs, the generation of self-localized waves with a broad spectrum, high average powers and an unparalleled mode efficiency compared to traditional micro-cavity-based frequency combs have been demonstrated [Citation108]. A typical implementation of the setup that is able to produce this new category of waves is depicted in ). It comprises a nonlinear Kerr micro-cavity (50 GHz free spectral range) responsible for the broadband generation, which is nested in a fiber amplifying loop providing energy to the system. In this case, amplification is achieved using an Erbium-doped fiber amplifier (EDFA). A delay line is used to adjust the temporal matching between pulses circulating in the microcavity and in the fiber cavity. By only adjusting these two parameters, one can obtain a broad range of regimes, from the well-known Turing pattern regime to the recently described laser cavity solitons (LCSs). As mentioned earlier, this setup delivers pulses at a 50 GHz repetition rate, which is incompatible with any DFT application. In particular, administrating to the pulses the dispersion needed to reach the far-field condition () would result in pulses overlapping.
Figure 9. DFT characterization of high repetition-rate frequency combs. (a) Microcomb generation: A Laser Cavity-Solitons train is emitted from a microresonator nested in a fiber cavity. Modulated Dispersive Fourier Transform: the repetition rate of the pulse train is reduced using an electro-optic modulator connected to a pulse generator (1,2). DFT is then achieved through a DCF (3) and data is processed using a fast oscilloscope. (b) Typical DFT-plot of a Turing pattern, startup and stable regime over 2000 roundtrips. Optical spectrum analyzer plot on the right for comparison [Citation109].
![Figure 9. DFT characterization of high repetition-rate frequency combs. (a) Microcomb generation: A Laser Cavity-Solitons train is emitted from a microresonator nested in a fiber cavity. Modulated Dispersive Fourier Transform: the repetition rate of the pulse train is reduced using an electro-optic modulator connected to a pulse generator (1,2). DFT is then achieved through a DCF (3) and data is processed using a fast oscilloscope. (b) Typical DFT-plot of a Turing pattern, startup and stable regime over 2000 roundtrips. Optical spectrum analyzer plot on the right for comparison [Citation109].](/cms/asset/d6b1d826-f5ed-4466-a94e-a595d8641226/tapx_a_2067487_f0009_oc.jpg)
This problem is solved by optically sampling the laser emission rate using amplitude modulation via an electro-optical modulator connected to a pulse generator as seen in ). By lowering the repetition rate to 33 MHz, the signal duty cycle can be reduced thus allowing pulse temporal stretching without overlap. Nevertheless, as most modulation system bandwidths do not allow to select single pulses at this repetition rate, bunches of 10 pulses are kept and the approximation that these can be considered as one average pulse is made. This approximation can be justified by the fact that this study is focused on longer timescales for which the pulse-to-pulse variation is negligible. Thus, a 10 pulse bunch can be considered as one average pulse, allowing the study of the spectral evolution over longer timescales. This step is achieved using a 3 GHz pulse generator (Keysight 8133A) with a 10 Gb/s LiNbO3 optical intensity modulator. The signal is modulated over a 300 ps width window, at a 30 ns repetition rate. The modulated signal can then be submitted to a DFT diagnostic. To do so, a 10 km-long telecom dispersion compensation fiber (DCF) is used, with a dispersion of D = −1360 ps/nm (β2 = 1730 ps2 at 1550 nm). Using this technique, one must be careful about the peak power of the input pulses, as nonlinearities can easily affect femtosecond pulses in dispersive fibers. illustrates a typical DFT-plot of a Turing pattern acquired by this setup [Citation109]. The regime can be seen starting from noise and quickly stabilizing after 200 roundtrips. An optical analyzer trace is displayed on the right showing good agreement with the DFT trace.
2.3 Example of DFT application: Time-stretch imaging of laser-induced shock waves
Conventional imaging systems such as CCD sensors are intrinsically limited by the trade-off between sensitivity and frame rate, and are often too slow to capture ultrafast phenomena. As a consequence, considerable efforts have been devoted to the development of ultrafast imaging systems in the last decade. On the one hand, the best performances to date in terms of frame rate are undoubtedly obtained by single-shot techniques which exhibit Tera-frames-per-second (Tfps) rates [Citation110,Citation111] with versatile setups [Citation112] but with relatively low sequence depths (i.e. number of images acquired). Such techniques are then well-suited to track ultrashort phenomena on the picosecond timescale but are unable to acquire data on longer timescales and then to capture non-repetitive and unpredictable events or to perform high precision statistical analysis. On the other hand, the ability of DFT for fast continuous single-shot measurements can be leveraged for all-optical imaging in a technique known as amplified time-stretch imaging or serial time-encoded amplified imaging (STEAM) [Citation38]. The principle of this technique is to encode an image (spatial domain) onto the broadband spectrum of an ultrashort pulse (spectral domain), which is then mapped into the time domain using DFT, followed by the recording of the image using a high-speed digitizer. In this 2-step process, the space-to-frequency mapping is performed using diffractive and dispersive elements while the frequency-to-time mapping is obtained using DFT within an amplifying scheme, as shown in ).
Figure 10. Capturing ultrafast phenomena using DFT-based imaging. (a) Time-stretch imaging setup. (b) Snapshot of a typical laser-induced SW recorded using shadowgraphy. (c) Signal of a single SW: consecutive pulses are stacked horizontally (time axis), while positions (i.e. wavelengths) are displayed vertically. (d) Close-up on the shock wave and (e) corresponding velocities measurements. Reproduced from [Citation113].
![Figure 10. Capturing ultrafast phenomena using DFT-based imaging. (a) Time-stretch imaging setup. (b) Snapshot of a typical laser-induced SW recorded using shadowgraphy. (c) Signal of a single SW: consecutive pulses are stacked horizontally (time axis), while positions (i.e. wavelengths) are displayed vertically. (d) Close-up on the shock wave and (e) corresponding velocities measurements. Reproduced from [Citation113].](/cms/asset/83e643c9-f158-44f7-a588-3e21364cd428/tapx_a_2067487_f0010_oc.jpg)
In order to reach the sensitivity levels required to observe the physical phenomena of interest but also to compensate for the losses in peak power due to the time-stretching process, the optical signal needs to be significantly amplified throughout its propagation. When working in the telecom wavelength range, such an amplification can be easily obtained using consecutive fibered stages based on different mechanisms, as shown in ). First, Erbium-doped fiber amplifiers (EDFAs) can be used prior and after the time-stretching process to reach the necessary power levels. Second, the dispersive medium (i.e. an optical fiber) itself can be used as an amplifying medium through the process of Raman amplification. Such an amplification is obtained by propagating several pump beams (two in the case presented here) with wavelengths precisely shifted away from the image beam so that the latter gets a homogeneous amplification over its spectrum through Raman gain. In the example presented here [Citation113], as the signal to amplify is centered at 1560 nm, two fiber-coupled laser diodes centered at 1446 and 1462 nm, respectively, are used. This setup has been implemented in order to track the propagation of laser-induced supersonic shock waves (SWs) (see an example of such a SW in )) and to perform statistics in order to reveal SW-to-SW fluctuations. The study of the fast dynamics involved in laser matter-interactions has indeed been a hot topic in the last decade as it impacts various research fields such as material analysis (e.g. LIBS), micromachining, nanoparticles production or high-harmonic generation. In the work by Hanzard et al. [Citation113], summarized in , time-stretch imaging has been used to monitor the full dynamics of single SW and to acquire sufficient velocity statistics. The transient phenomena have been encoded onto a 1-D spectral sheet, allowing to monitor the complex gas dynamics following laser ablation on a long timescale, from SW deceleration ()) to other gas discontinuities such as plasma contact waves ()). This work then proves DFT-based imaging to be particularly suited to study SW dynamics and interactions as well as fast non-repetitive events occurring in laser ablation. Time-stretch imaging, which is usually based on standard ultrafast optics lab equipment, could then be routinely used as a complementary method to single-shot imaging techniques or streak cameras for a wide span of scientific applications.
2.4 Leveraging DFT for advanced quantum measurements
Combined with infrastructure employed to detect and manipulate quantum signals, the framework of quantum-inspired DFT has been developed to enable processing quantum signals at the single-photon level. It brings advantages by delivering improvements in run-time as well as sampling rate, enhancing sensitivity, and importantly enabling time-efficient characterization of photonic quantum states. In a similar fashion to classical DFT concepts, in quantum-inspired DFT, a non-classical signal propagates through a dispersive element whereupon the frequency components of the single-photon signal are mapped onto the temporal domain, such that the time-stretched output wave packet entirely mimics the characteristics of the spectral wave function. In lieu of intensity photo detectors, a single-photon detection system is deployed whose combination with a timing electronics module enables to record single-photon as well as coincidence detection events at given times. The repetitive execution of experiments comprising synchronized detections with the repetition rate of the excitation source allows to measure the statistics governing the process under study. It is important to note that the single-photon detections are probabilistic events and an ensemble of events is required to gain information on the quantum signal. Every single detection therefore contributes to the formation of a histogram from which the probability distribution corresponding to the spectral shape of the non-classical signal can be retrieved. Nevertheless, under the quantum-inspired DFT approach, correlations in non-repetitive signals can also be obtained through comparison of dependent and independent statistics (see below).
The performance of the quantum-inspired DFT scheme strongly depends on the characteristics of the single-photon detection system and the dispersive element employed. Large detector bandwidth, i.e. high timing resolutions with low jitter and high dispersion, brings adjustable access to high-frequency resolution. A high quantum efficiency at the same time allows for high sensitivity of this measurement concept.
As an efficient technique, quantum-inspired DFT has served optical coherence tomography (OCT) imaging by addressing the extremely low optical power levels emitted by scattering or highly absorptive objects, yet with comparable quality to standard OCT systems. Accordingly, fast and ultra-sensitive OCT imaging has enabled high-fidelity capture of fluctuations at permissible optical power densities that meet the human-eye safety thresholds [Citation114].
Quantum-inspired DFT has also proved useful towards obtaining repetitive spectral and temporal statistics of optical parametric processes, such as spontaneous parametric down conversion (SPDC) and spontaneous four wave mixing (SFWM), two widely exploited photon-pair sources with applications in quantum information processing and quantum-enhanced metrology. Indeed, the source characterization has benefited from single-photon spectrometers – a combination of DFT with a fiber-based dispersive medium and single-photon detection system – in obtaining unprecedented spectral resolution and significantly improved integration time per measurement [Citation115–117], by comparison to traditional characterization techniques that suffer from low sampling rate and long run-time implementation [Citation116,Citation118,Citation119].
Specifically, in recent realizations, highly time-energy correlated photon pairs (so-called signal and idler photons) were generated by pulsed-driven SPDC processes within a periodically poled lithium Niobate (PPLN) waveguide (see )).
Figure 11. (a) Experimental setup to implement quantum DFT employed for spectral characterization of photon pairs created in pulsed-excited SPDC process in a PPLN waveguide. (b) Joint Spectral Intensity (JSI) determined by quantum DFT: CAR versus signal and idler frequency. (c) Coincidence detection as a function of the frequency difference between the spectral components of the signal photon. The inset figure illustrates the dependency of
on frequency difference,
.
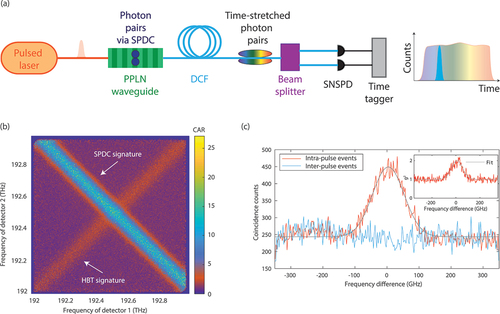
The frequency components of the SPDC output spectrum were stretched in time through the DFT process within a DCF. The frequency-dependent time-delayed arrival of the spectral components at the detection system were temporally resolvable thanks to the low timing jitter of the SNSPDs which lay in the range of approx. 25 ps. Together with a group delay dispersion of 960 ps/nm this combination led to a frequency resolution of approximately 3 GHz. Two single-photon detectors were employed to measure simultaneously two photons at a time. The time-resolved two-fold coincidence detections synchronized with the pulsed laser repetition rate (50 MHz) enabled the reconstruction of the joint spectral intensity (JSI) (see )) as an important characteristic of time-energy entangled photon sources, providing information on the frequency correlation and its bandwidth. Relating dependent statistics, i.e. two-photon coincidence events form the same excitation pulse, to independent statistics, i.e. coincidence events from consecutive excitation pulses, allows to measure the coincidence to accidental ratio (CAR) corresponding to different signal/idler frequency combinations. The CAR over the frequency range is in particular important to assess the quantum source performance, especially for applications in reconfigurable quantum networks.
At the same time, the quantum-inspired DFT can give further information on the purity and number of frequency modes of the quantum signal when combined with a Hanbury Brown and Twiss (HBT) experimental configuration. Specifically, in a recent work, the quantum-inspired DFT was deployed to demonstrate for the first time the direct implementation of frequency-domain HBT interferometry, revealing the dependency of the second-order auto-correlation function on frequency components, i.e. and
, expressed as
with and
being the single-photon creation and annihilation operators, respectively. Specifically, in the same experimental setup as described above, the signal (or idler) photon is directed merely through a DCF, and a 50:50 beam splitter is used to send the photons to two single-photon detectors for coincidence detection, allowing the determination of the frequency-resolved
associated with the signal (idler) spectral range (see )). Importantly, this gives access to single-photon wave packet characteristics. In particular, it allows the direct determination of the single-mode frequency bandwidth, here 106 GHz, which was not directly possible to measure with previously adopted characterization techniques relying on time-resolved measurement of
.
In conclusion, quantum-inspired DFT brings many advantages related to system sensitivity and direct characterization of photonic quantum states, with a plethora of application possibilities in imaging, metrology and quantum information processing.
3. Discussion
Owing to their inherent simplicity, DFT techniques and associated time-stretch instrumentation have had a tremendous impact in various fields of ultrafast photonics, spanning fundamental studies of laser build-up dynamics and the emergence of extreme events from noise-driven nonlinear interactions in fiber optics. Dispersive Fourier transform has now become a standard characterization tool (which has been thoroughly refined for dedicated applicative frameworks), while, at the same time, the ready availability of time-lens systems brought about advanced diagnostic techniques in many labs around the world, even those whose expertise is not necessarily focused on the development of ultrafast photonic diagnostic tools. As a result, these systems are now employed in a wide variety of applications, extending the landscape of standard approaches available for processing and measuring light properties in areas covering microscopy, imaging, spectroscopy, physical monitoring, metrology, as well as quantum science. The development of DFT has been a clear example of how the arrival of a new characterization technique has led to numerous new advances in different fields, and new ways of revisiting instability phenomena that had only been incompletely measured in the past.
While many avenues of ultrafast optics are currently being explored with DFT [Citation28,Citation29], it appears clear that the inherent processing speed and capabilities to generate and handle large data sets make these real-time characterization approaches extremely valuable for extending the dimensionality of DFT analysis (in e.g. multimode fiber [Citation120] or systems based on frequency combs [Citation4,Citation108,Citation121,Citation122]). In parallel, quantum inspired DFT implementations are expected to open new bridges between classical, nonlinear and quantum photonics, from both an applied viewpoint (with directly relevant applications such as imaging, ultrafast quantum processing, etc.) to the fundamental study of noise-driven and unstable nonlinear dynamics associated with low power optical signals (e.g. in regimes placed between spontaneous, induced and cascaded frequency conversion processes). In addition, the complementary implementations of DFT, TL and/or heterodyne techniques now allow to access the complex optical field structure in a straightforward manner, lifting intrinsic limitations associated with averaged or deconvolved measurements. Altogether, we expect these advances to allow for the exploration of novel frontiers in ultrafast and laser physics, but also to tackle more fundamental challenges in the study of light-matter interactions. Specifically, we foresee these latest developments in ultrafast optical monitoring to act as a strong catalyst to enable new implementations of ‘smart photonic systems’ which can readily exploit real-time measurements for machine learning analysis and optimization of complex optical wave packets [Citation67,Citation69,Citation123,Citation124]. There is no doubt that, once again, DFT and real-time measurements may prove a game changer to bridge gaps between an ever-growing variety of multidisciplinary applications in photonics and beyond.
Data availability
The data that support the findings of this study are available from the corresponding author, BW, upon reasonable request.
Acknowledgments
The authors are grateful to M. Rowley, B. Little, S. Chu and M. Peccianti for providing the microcomb source used to test the DFT method in section 2.2. The authors are also grateful to M. Chernysheva and S. Turitsyn for accepting the reproduction of their results in .
Disclosure statement
No potential conflict of interest was reported by the author(s).
Additional information
Funding
References
- Dudley JM, Taylor JR. Supercontinuum generation in optical fibers. Cambridge: Cambridge University Press; 2010.
- Boyd RW. Nonlinear Optics. Burlington: MA, Elsevier Science; 2013.
- Weiner A. Ultrafast Optics. Hoboken: NJ: Wiley Publishing; 2009.
- Pasquazi A, Peccianti M, Razzari L, et al. Micro-Combs: a novel generation of optical sources. Phys Rep. 2018;729:1.
- Reimer C, Kues M, Caspani L, et al. Cross-Polarized photon-pair generation and bi-chromatically pumped optical parametric oscillation on a chip. Nat Commun. 2015;6:8236.
- Kues M, Reimer C, Roztocki P, et al. On-chip generation of high-dimensional entangled quantum states and their coherent control. Nature. 2017;546:622.
- Zipfel WR, Williams RM, Webb WW. Nonlinear magic: multiphoton microscopy in the biosciences. Nat Biotechnol. 2003;21:1369.
- Camp CH Jr, Cicerone MT. Chemically sensitive bioimaging with coherent raman scattering. Nat Photonics. 2015;9:295.
- Agrawal GP. Nonlinear fiber optics, Nonlinear Science at the Dawn of the 21st Century. Vol. 542:195, Berlin: Springer; 2000.
- Walmsley IA, Dorrer C. Characterization of ultrashort electromagnetic pulses. Adv Opt Photonics. 2009;1:308.
- Trebino R. Frequency-resolved optical gating: the measurement of ultrashort laser pulses. Springer, US. 2000.
- O’Shea P, Kimmel M, Gu X, et al. Highly simplified device for ultrashort-pulse measurement. Opt Lett. 2001;26:932.
- Cormack IG, Sibbett W, Reid DT. Rapid measurement of ultrashort-pulse amplitude and phase from a two-photon absorption sonogram trace. JOSA B. 2001;18:1377.
- Wong TC, Rhodes M, Trebino R. Single-shot measurement of the complete temporal intensity and phase of supercontinuum. Optica. 2014;1:119.
- Iaconis C, Walmsley IA. Spectral phase interferometry for direct electric-field reconstruction of ultrashort optical pulses. Opt Lett. 1998;23:792.
- Messager V, Louradour F, Froehly C, et al. Coherent measurement of short laser pulses based on spectral interferometry resolved in time. Opt Lett. 2003;28:743.
- Pasquazi A, Peccianti M, Park Y, et al. Sub-picosecond phase-sensitive optical pulse characterization on a chip. Nat Photonics. 2011;5:10.
- Pasquazi A, Peccianti M, Azaña J, et al. FLEA: Fresnel-limited extraction algorithm applied to spectral phase interferometry for direct field reconstruction (SPIDER). Opt Express. 2013;21:5743.
- Bhushan AS, Coppinger F, Jalali B. Time-stretched analogue-to-digital conversion. Electron Lett. 1998;34:839.
- Jannson T. Real-time Fourier transformation in dispersive optical fibers. Opt Lett. 1983;8:232.
- Akhmanov SA, Vysloukh VA, Chirkin AS. Self-action of wave packets in a nonlinear medium and femtosecond laser pulse generation. Sov Phys Uspekhi. 1986;29:642.
- Tong YC, Chan LY, Tsang HK. Fibre dispersion or pulse spectrum measurement using a sampling oscilloscope. Electron Lett. 1997;33:983.
- Solli DR, Ropers C, Koonath P, et al. Optical Rogue Waves. Nature. 2007;450:7172.
- Wetzel B, Stefani A, Larger L, et al. Real-time full bandwidth measurement of spectral noise in supercontinuum generation. Sci Rep. 2012;2:882.
- Godin T, Wetzel B, Dudley JM, et al. Ultrafast single-shot measurements in modulation instability and supercontinuum. Opt. Photonics News. 2013;24:55.
- Wang X, Bigourd D, Kudlinski A, et al. Correlation between multiple modulation instability side lobes in dispersion oscillating fiber. Opt Lett. 2014;39:1881.
- Dudley JM, Dias F, Erkintalo M, et al. Instabilities, breathers and rogue waves in optics. Nat Photonics. 2014;8:10.
- Goda K, Jalali B. Dispersive Fourier transformation for fast continuous single-shot measurements. Nat Photonics. 2013;7. DOI:10.1038/nphoton.2012.359
- Mahjoubfar A, Churkin DV, Barland S, et al. Time stretch and its applications. Nat Photonics. 2017;11:6.
- Mahjoubfar A, Goda K, Ayazi A, et al. High-speed nanometer-resolved imaging vibrometer and velocimeter. Appl Phys Lett. 2011;98:101107.
- Mance JG, Lone BML, Dolan DH, et al. Time-stretched photonic Doppler velocimetry. Opt Express. 2019;27:25022.
- Solli DR, Chou J, Jalali B. Amplified wavelength–time transformation for real-time spectroscopy. Nat Photonics. 2008;2:48–30.
- DeVore PTS, Buckley BW, Asghari MH, et al. Coherent time-stretch transform for near-field spectroscopy. IEEE Photonics J. 2014;6:1.
- Dobner S, Fallnich C. Dispersive Fourier transformation femtosecond stimulated Raman scattering. Appl Phys B. 2016;122:278.
- Saltarelli F, Kumar V, Viola D, et al. Broadband stimulated Raman scattering spectroscopy by a photonic time stretcher. Opt Express. 2016;24:21264.
- Ryczkowski P, Amiot CG, Dudley JM, et al. Experimental demonstration of spectral domain computational ghost imaging. Sci Rep. 2021;11. DOI:10.1038/s41598-021-87355-z
- Kawai A, Hashimoto K, Dougakiuchi T, et al. Time-Stretch infrared spectroscopy. Commun Phys. 2020;3. DOI:10.1038/s42005-020-00420-3
- Goda K, Tsia KK, Jalali B. Serial time-encoded amplified imaging for real-time observation of fast dynamic phenomena. Nature. 2009;458:7242.
- Jiang Y, Karpf S, Jalali B. Time-Stretch LiDAR as a spectrally scanned time-of-flight ranging camera. Nat Photonics. 2020;14:14–18.
- Wong TTW, Lau AKS, Wong KKY, et al. Optical time-stretch confocal microscopy at 1µm. Opt Lett. 2012;37:3330.
- Lau AKS, Tang AHL, Xu J, et al. Optical time stretch for high-speed and high-throughput imaging—from single-cell to tissue-wide scales. IEEE J Sel Top Quantum Electron. 2016;22:89.
- Lei C, Wu Y, Sankaranarayanan AC, et al. GHz optical time-stretch microscopy by compressive sensing. IEEE Photonics J. 2017;9:1.
- Dong X, Zhou X, Kang J, et al. Ultrafast time-stretch microscopy based on dual-comb asynchronous optical sampling. Opt Lett. 2018;43:2118.
- Tan S, Wei X, Li B, et al. Ultrafast optical imaging at 2.0μm through second-harmonic-generation-based time-stretch at 1.0μm. Opt Lett. 2018;43:3822.
- Duffieux PM. The Fourier transform and its applications to optics. Hoboken: NJ: Wiley; 1983.
- Goodman JW. Introduction to Fourier optics. New York City: McGraw-Hill; 1968.
- Gaskill JD. Linear systems, Fourier transforms, and optics. Hoboken: NJ: Wiley; 1978.
- Born M, Wolf E. Principles of optics: electromagnetic theory of propagation, interference and diffraction of light. 7th ed. Cambridge: Cambridge University Press; 1999.
- Lei C, Guo B, Cheng Z, et al. Optical time-stretch imaging: principles and applications. Appl Phys Rev. 2016;3:011102.
- Grigoryan GV, Lima JIT, Yu T, et al., Using color to understand light transmission. Opt Photonics News. 2000;11:44.
- Azaña J, Chen LR. General Temporal Self-Imaging Phenomena. JOSA B. 2003;20:1447.
- Fernández-Pousa CR. A dispersion-balanced discrete Fourier transform of repetitive pulse sequences using temporal Talbot effect. Opt Commun. 2017;402:97.
- Konatham SR, de Chatellus HG, Azaña J. Photonics-based real-time spectrogram analysis of broadband waveforms. J Light Technol. 2020;38:5356.
- Cortés LR, Onori D, de Chatellus HG, et al. Towards on-chip photonic-assisted radio-frequency spectral measurement and monitoring. Optica. 2020;7:434.
- Goda K, Solli DR, Tsia KK, et al. Theory of amplified dispersive Fourier transformation. Phys Rev A. 2009;80:043821.
- Sørensen ST, Bang O, Wetzel B, et al. Describing supercontinuum noise and rogue wave statistics using higher-order moments. Opt Commun. 2012;285:2451.
- Solli DR, Herink G, Jalali B, et al. Fluctuations and correlations in modulation instability. Nat Photonics. 2012;6:463–468.
- Nguyen DM, Godin T, Toenger S, et al. Incoherent resonant seeding of modulation instability in optical fiber. Opt Lett. 2013;38:5338.
- Wabnitz S, Wetzel B. Instability and noise-induced thermalization of fermi–pasta–ulam recurrence in the nonlinear schrödinger equation. Phys Lett A. 2014;378:2750.
- Sugavanam S, Sorokina M, Churkin DV. Spectral correlations in a random distributed feedback fibre laser. Nat Commun. 2017;8. DOI:10.1038/ncomms15514
- Robert P, Fourcade-Dutin C, Fourcade-Dutin C, et al. Spectral correlation of four-wave mixing generated in a photonic crystal fiber pumped by a chirped pulse. Opt Lett. 2020;45:4148.
- Godin T, Wetzel B, Sylvestre T, et al. Real time noise and wavelength correlations in octave-spanning supercontinuum generation. Opt Express. 2013;21:18452.
- Lei L, Huh J, Cortés LR, et al. Observation of spectral self-imaging by nonlinear parabolic cross-phase modulation. Opt Lett. 2015;40:5403.
- Fernández MP, Fernández MP, Fernández MP, et al. Nonlinear time-lens with improved power efficiency through a discrete multilevel pump. Opt Lett. 2020;45:3557.
- Asghari MH, Jalali B. Anamorphic transformation and its application to time-bandwidth compression. Appl Opt. 2013;52:6735.
- Jalali B, Chan J, Asghari MH. Time-Bandwidth engineering. Optica. 2014;1:23.
- Närhi M, Salmela L, Toivonen J, et al. Machine learning analysis of extreme events in optical fibre modulation instability. Nat Commun. 2018;9. DOI:10.1038/s41467-018-07355-y
- Dudley JM, Genty G, Coen S. Supercontinuum generation in photonic crystal fiber. Rev Mod Phys. 2006;78:1135.
- Salmela L, Lapre C, Dudley JM, et al. Machine learning analysis of rogue solitons in supercontinuum generation. Sci Rep. 2020;10. DOI:10.1038/s41598-020-66308-y
- Toenger S, Godin T, Billet C, et al. Emergent rogue wave structures and statistics in spontaneous modulation instability. Sci Rep. 2015;5. DOI:10.1038/srep10380
- Wetzel B, Bongiovanni D, Kues M, et al. Experimental generation of Riemann waves in optics: a route to shock wave control. Phys Rev Lett. 2016;117:073902.
- Bongiovanni D, Bongiovanni D, Wetzel B, et al. Third-order Riemann pulses in optical fibers. Opt Express. 2020;28:39827.
- Närhi M, Wetzel B, Billet C, et al. Real-time measurements of spontaneous breathers and rogue wave events in optical fibre modulation instability. Nat Commun. 2016;7:13675.
- Suret P, Koussaifi RE, Tikan A, et al. Single-shot observation of optical rogue waves in integrable turbulence using time microscopy. Nat Commun. 2016;7. DOI:10.1038/ncomms13136
- Randoux S, Gustave F, Suret P, et al. Optical random Riemann waves in integrable turbulence. Phys Rev Lett. 2017;118:233901.
- Kolner BH. Space-time duality and the theory of temporal imaging. IEEE J Quantum Electron. 1994;30:1951.
- Salem R, Foster MA, Turner AC, et al. Optical time lens based on four-wave mixing on a silicon chip. Opt Lett. 2008;33:1047.
- Foster MA, Salem R, Geraghty DF, et al. Silicon-chip-based ultrafast optical oscilloscope. Nature. 2008;456:7218.
- Salem R, Foster MA, Turner-Foster AC, et al. High-speed optical sampling using a silicon-chip temporal magnifier. Opt Express. 2009;17:4324.
- Pasquazi A, Park Y, Chu ST, et al. Time-lens measurement of subpicosecond optical pulses in CMOS compatible high-index glass waveguides. IEEE J Sel Top Quantum Electron. 2012;18:629.
- Bessin F, Copie F, Conforti M, et al. Real-time characterization of period-doubling dynamics in uniform and dispersion oscillating fiber ring cavities. Phys Rev X. 2019;9:041030.
- Ryczkowski P, Närhi M, Billet C, et al. Real-time full-field characterization of transient dissipative soliton dynamics in a mode-locked laser. Nat Photonics. 2018;12:221–227.
- Dorrer C. Single-shot measurement of the electric field of optical waveforms by use of time magnification and heterodyning. Opt Lett. 2006;31:540.
- Tikan A, Bielawski S, Szwaj C, et al. Single-shot measurement of phase and amplitude by using a heterodyne time-lens system and ultrafast digital time-holography. Nat Photonics. 2018;12:228–234.
- Lebel A, Tikan A, Tikan A, et al. Single-shot observation of breathers from noise-induced modulation instability using heterodyne temporal imaging. Opt Lett. 2021;46:298.
- Crockett B, Romero Cortés L, Konatham SR, et al. Full recovery of ultrafast waveforms lost under noise. Nat Commun. 2021;12. DOI:10.1038/s41467-021-22716-w
- Herink G, Jalali B, Ropers C, et al. Resolving the build-up of femtosecond mode-locking with single-shot spectroscopy at 90 MHz frame rate. Nat Photonics. 2016;10:321–326.
- Peng J, Sorokina M, Sugavanam S, et al. Real-time observation of dissipative soliton formation in nonlinear polarization rotation mode-locked fibre lasers. Commun Phys. 2018;1. DOI:10.1038/s42005-018-0022-7
- Liu X, Popa D, Akhmediev N. Revealing the transition dynamics from Q switching to mode locking in a soliton laser. Phys Rev Lett. 2019;123:093901.
- Meng F, Lapre C, Billet C, et al. Intracavity incoherent supercontinuum dynamics and rogue waves in a broadband dissipative soliton laser. Nat Commun. 2021;12:5567.
- Lapre C, Billet C, Meng F, et al. Real-time characterization of spectral instabilities in a mode-locked fibre laser exhibiting soliton-similariton dynamics. Sci Rep. 2019;9. DOI:10.1038/s41598-019-50022-5
- Liu X, Yao X, Cui Y. Real-time observation of the buildup of soliton molecules. Phys Rev Lett. 2018;121:023905.
- Runge AFJ, Aguergaray C, Broderick NGR, et al. Coherence and shot-to-shot spectral fluctuations in noise-like ultrafast fiber lasers. Opt Lett. 2013;38:4327.
- Runge AFJ, Broderick NGR, Erkintalo M. Observation of soliton explosions in a passively mode-locked fiber laser. Optica. 2015;2(36):36.
- Grelu P, Akhmediev N. Dissipative solitons for mode-locked lasers. Nat Photonics. 2012;6:84–92.
- Herink G, Kurtz F, Jalali B, et al. Real-time spectral interferometry probes the internal dynamics of femtosecond soliton molecules. Science. 2017;356:50.
- Krupa K, Nithyanandan K, Andral U, et al. Real-time observation of internal motion within ultrafast dissipative optical soliton molecules. Phys Rev Lett. 2017;118:243901.
- Sugavanam S, Fabbri S, Le ST, et al. Real-time high-resolution heterodyne-based measurements of spectral dynamics in fibre lasers. Sci Rep. 2016;6. DOI:10.1038/srep23152
- Peng J, Boscolo S, Zhao Z, et al. Breathing dissipative solitons in mode-locked fiber lasers. Sci Adv. n.d.;5:eaax1110.
- Krupa K, Nithyanandan K, Grelu P. Vector dynamics of incoherent dissipative optical solitons. Optica. 2017;4:1239.
- Kurtz F, Ropers C, Herink G. Resonant excitation and all-optical switching of femtosecond soliton molecules. Nat Photonics. 2020;14:9–13.
- Runge AFJ, Aguergaray C, Broderick NGR, et al. Raman rogue waves in a partially mode-locked fiber laser. Opt Lett. 2014;39:319.
- Chernysheva M, Sugavanam S, Turitsyn S. Real-time observation of the optical Sagnac effect in ultrafast bidirectional fibre lasers. APL Photonics. 2020;5:016104.
- Descloux D, Laporte C, Dherbecourt J-B, et al. Spectrotemporal dynamics of a picosecond OPO based on chirped quasi-phase-matching. Opt Lett. 2015;40:280.
- Ivanauskienė K, Stasevičius I, Vengris M, et al. Pulse-to-pulse instabilities in synchronously pumped femtosecond optical parametric oscillator. JOSA B. 2019;36:131.
- Chen X, Yang S, Ding S, et al. Instantaneous dynamics of a fiber optical parametric oscillator within its initiating process. IEEE Photonics Technol Lett. 2019;31:1088.
- Touil M, Becheker R, Godin T, et al. Spectral Correlations in a Fiber-Optical Parametric Oscillator. Phys Rev A. 2021;103:043503.
- Bao H, Cooper A, Rowley M, et al. Laser cavity-soliton microcombs. Nat Photonics. 2019;13:384.
- Hanzard PH, Rowley M, Cutrona A, et al., Real-time study of coexisting states in laser cavity solitons, in conference on lasers and electro-optics (2021), Paper FM4H.7 San Jose, California, United States (Optical Society of America), p. FM4H.7.
- Liang J, Wang LV. Single-Shot ultrafast optical imaging. Optica. 2018;5:1113.
- Qi D, Zhang S, Yang C, et al. Single-shot compressed ultrafast photography: a review. Adv Photonics. 2020;2:014003.
- Touil M, Idlahcen S, Becheker R, et al. Acousto-optically driven lensless single-shot ultrafast optical imaging. Light Sci. Appl. 2022;11. DOI:10.1038/s41377-022-00759-y
- Hanzard P-H, Godin T, Idlahcen S, et al. Real-time tracking of single shockwaves via amplified time-stretch imaging. Appl Phys Lett. 2018;112:161106.
- Kolenderska SM, Kolenderska SM, Vanholsbeeck F, et al. Quantum-Inspired detection for spectral domain optical coherence tomography. Opt Lett. 2020;45:3443.
- Avenhaus M, Eckstein A, Mosley PJ, et al. Fiber-Assisted single-photon spectrograph. Opt Lett. 2009;34:2873.
- Eckstein A, Boucher G, Lemaître A, et al. High-resolution spectral characterization of two photon states via classical measurements. Laser Photonics Rev. 2014;8:L76.
- Khodadad Kashi A, Sader L, Haldar R, et al. Frequency-to-time mapping technique for direct spectral characterization of biphoton states from pulsed spontaneous parametric processes. Front. Photonics. 2022;3:834065.
- Johnsen KD, Kolenderski P, Scarcella C, et al. Time and spectrum-resolving multiphoton correlator for 300–900 nm. J Appl Phys. 2014;116:143101.
- Kaneda F, Oikawa J, Yabuno M, et al. Spectral characterization of photon-pair sources via classical sum-frequency generation. Opt Express. 2020;28:38993.
- Krupa K, Tonello A, Barthélémy A, et al. Multimode nonlinear fiber optics, a spatiotemporal avenue. APL Photonics. 2019;4:110901.
- Wei X, Shen Y, Jing JC, et al. Real-time frequency-encoded spatiotemporal focusing through scattering media using a programmable 2D ultrafine optical frequency comb. Sci Adv. 2020;6:eaay1192.
- Xu X, Tan M, Corcoran B, et al. 11 TOPS photonic convolutional accelerator for optical neural networks. Nature. 2021;589:7840.
- Wetzel B, Kues M, Roztocki P, et al. Customizing supercontinuum generation via on-chip adaptive temporal pulse-splitting. Nat Commun. 2018;9. DOI:10.1038/s41467-018-07141-w
- Genty G, Salmela L, Dudley JM, et al. Machine learning and applications in ultrafast photonics. Nat Photonics. 2020;15:91.