ABSTRACT
Cancer tissues are a heterogeneously multifaceted assembly. Understanding the relationship of tumors with their microenvironment is also required to understand the tumor progression and metastasis better. Like tumors, the tumor microenvironment (TME) is heterogeneous, offering numerous mechanobiological, mechanochemical, and mechanophysical cues. Biomaterials impersonating extracellular matrix (ECM) properties must provide the mechanical cues cells get from their 3D extracellular environment. Pore size is one imperative yet less studied ECM factor implicated in the invasion and migration of the tumor. Several techniques are used to control the pore size of biomaterials constructed for a distinct tissue. Electrospinning is one of the most steadfast techniques for producing scaffolds with the preferred pore size. A comprehensive interpretation of ECM pore size would contribute toward a better understanding of the reciprocal interaction between pore size and tumor progression and can be used as a promising target for cancer treatments. In this review, we abridged the knowledge pertaining to (1) ECM and pore size, (2) the importance of pore size and its interplay with cancer, and (3) current advancement in the field of biomaterials to study pore size. Overall, this review will cover the effect of pore size on tumor cell behavior concerning electrospinning.
ABSTRACT
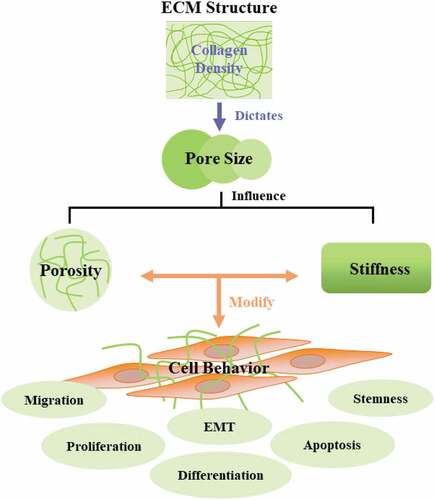
1. Introduction
Globally 19.3 million cases and 10 million cancer-related mortalities have been reported in 2020 [Citation1]. Cancer cell migration is a complex biological process influenced by the properties of migrating cells and their environment [Citation2]. Tumor prognostic methods are based on the clinicopathological features; tumor (T), node (N), metastasis (M) TNM grading. It is an effective prognostic strategy, yet the patients with the same prognosis showed varied overall survival [Citation3]. Despite significant breakthroughs in prognosis and treatment, higher tumor heterogeneity demands further exploration of tumor and its associated factors. There is still a need to understand better the role of major molecular players involved in tumors and their clinical relevance.
Extracellular Matrix (ECM) is one of the critical biological frameworks responsible for tissue homeostasis and plays a role in carcinogenesis. ECM is a non-cellular entity and maintains tissues’ biochemical and physical permanence. ECM and cells exhibit bidirectional interactions and are responsible for cell adhesion and migrations [Citation4]. Given the importance of ECM, in the last decade, extensive studies have been carried out to understand the fundamental properties of ECM; dimensionality (2 dimensional 2D or 3 dimensional 3D), cell polarity, shape, cell adhesion surface, area, nano-topography, viscoelasticity, stiffness, porosity, static and deformation applied to matrix and pore size. All these constraints have influenced stem cell choices [Citation5], and stem cell is an indispensable factor in tumor progression.
Tissue engineering intends to use 3D scaffolds to provide a suitable microenvironment for investigating cell behavior [Citation6] and incorporate growth factors for tissue engineering. The scaffolds mimic the actual in vivo 3D microenvironment and provide suitable cell interaction and behavior cues. Hence material properties of the scaffolds are essential in determining the cellular behavior [Citation7]. The pore size and porosity of 3D scaffolds have direct implications for their biomedical applications. Pores are essential for cell nutrition, tissue vascularization, proliferation, and migration [Citation8]. A previous study demonstrated that permeability increases with increasing pore size due to a reduction in the specific surface area [Citation9]. If the pore size is small, it will restrict the cell migration, diffusion of nutrients, and removal of waste metabolites. If the pore size is too large, it will reduce the surface area for cell attachments. Despite the importance of pore size, the relationship between cell behavior and scaffold pore size is poorly understood, resulting in conflicting reports within the literature on the optimal pore size for studying cell activity. Herein, we critically assess the available literature for the optimal pore size and techniques being used or can be used to study the relationship between pore size and tumor cell behavior.
2. Extracellular matrix in tumor microenvironment and cancer
2.1 ECM in tumor
ECM, a part of the tumor microenvironment (TME), is a highly intricate, complex dynamic structure constantly remodeled and differs from the normal organs. ECM controls the intratumoral signaling, metabolism, oxygenation, transport mechanisms, and immunogenicity. ECM regulations influence malignancy, tumor growth, and response to therapy. It generates the mechanical and biochemical properties of the organs, such as elasticity and tensile strength, extracellular homeostasis, water retention, binding of growth factors, signal transductions, and gene transcription regulations. Biomechanical, biochemical, and organizational properties of ECM differ between normal and cancerous tissues, as shown in . Therefore, understanding the idiosyncrasies of ECM in tumors is essential to understanding the role of ECM in tumor progression and treatments [Citation10,Citation11]. Components of ECM link to give stable composite structures for mechanical properties of tissues. ECM is vital in controlling the behavior and characteristic of cells, such as adhesion, migration, proliferation, differentiation, polarity, and apoptosis [Citation12,Citation13].
Figure 1. Schematic of healthy and diseased tissues. The BM serves as a structural barrier to cancer cell invasion, intravasation, and extravasation derived from [Citation14].
![Figure 1. Schematic of healthy and diseased tissues. The BM serves as a structural barrier to cancer cell invasion, intravasation, and extravasation derived from [Citation14].](/cms/asset/76742525-5b3f-457f-9023-7e912614b707/tapx_a_2153624_f0001_oc.jpg)
Since ECM is necessary, in the last decade, extensive studies have been carried out to understand the fundamental properties of ECM; dimensionality (2D or 3D), cell polarity, shape, cell adhesion surface, area, nanotopography, viscoelasticity, stiffness, porosity, static and deformation applied to matrix and pore size. All these parameters have been shown to influence stem cell choices [Citation5], and stem cell is an essential factor in tumor progression. The mechanical properties of ECM have emerged as a critical cell fate regulator. These properties of ECM are significant in tumorigenesis, but the underlying mechanisms of these physical factors are still far from understood.
The development of material-based biomaterial mimic ECM conditions have been used to understand the role of physical properties of ECM factors in cell fate decision. Nevertheless, it is difficult to isolate each factor and study it independently. New biomaterials are being designed to study substrate mechanics in more complex ECM [Citation15]. Although all the physical factors of ECM have their role in tumor progression, pore size is crucial in tumor migration. Migration of tumor cells through the ECM depends more on pore size than on stiffness, with small and large pore sizes favoring protease-dependent and -independent migration, respectively [Citation16].
2.2. Pore size; significant parameter in ECM and cell fate determination
ECM proteins are fibrous proteins (collagen, fibronectin, laminin, and fibronectin) and glycosaminoglycan (hyaluronic acid, keratan, chondroitin, and heparan sulfate). These molecules exhibit intricate mesh-like crosslinked distribution [Citation17]. Pore size is the gap between collagen fibrils or within the basement membrane [Citation18]. Pore size is an essential factor determining cell phenotype, cell behavior, and secretions on ECM on scaffold surfaces [Citation19]. Macropores with a pore size of >50 μm can determine cell proliferation and colonization, whereas micropores with a size <50 μm influence the adsorption of proteins on scaffolds [Citation20]. Rapid angiogenesis was observed in large pore sizes of 160–270 μm [Citation21]. The pore size of 0.849 μm with 30% porosity promotes metabolic activities and cell proliferation, whereas the pore size of 0.896 μm with 39% porosity showed higher cell aggregations and differentiation [Citation22]. Similarly, porosity is vital in neovascularization during tissue engineering survival after transplantation and is well documented [Citation23]. Suggesting pore size is an essential contributing factor in tumor development and progression. Despite recent studies, there are still conflicting reports about the optimum pore size and the relationship of pore size with cell behavior.
Since ECM is disorganized in a tumor, which presents physical barriers for the moving cells, this barrier can either oppose or facilitate the motility of tumor cells depending on the pore size. Cells navigating through these pores remodel their cytoskeleton or ECM. Stiff cytoplasmic organelles and relatively large nuclei limit the migrating cells’ capacity to squeeze through ECM pores and physical barriers [Citation24]. The nucleus dictates the cellular movements [Citation25] and negotiates how ECM will be remodeled. Nuclear structural proteins lamin-A and lamin-C are significant factors in sensing nucleoskeletal stiffness. The expression of these proteins correlates with the cell navigating ability through ECM pores [Citation26]. Overabounded collagen leads to smaller pore size [Citation27,Citation28]. Invading cancer cells through these tiny pores squeezes these physical barriers and undergoes more physical damage [Citation29]. This squeezing would result in the isolation of nuclear proteins involved in DNA repair; hence this pore size contributes to the genomic instability of cells [Citation30]. Pore size is a decisive factor in cell trafficking. If the pore size is too smaller than nuclear dimensions, it will provide an impassable barrier to cells.
On the other hand, if the pore size is larger than nuclear dimensions, the physical barrier will no longer exist, and the cell will undergo invasion [Citation31]. Tumor cells bypass these barriers either by; 1. Proteolytic remodeling of ECM or 2. Non-proteolytic remodeling or matrix plasticity [Citation32] is shown in . The above serves the Importance of ECM and the role of pores in tumorigenesis. Despite the growing focus on studying biomaterial synthesis to study the individual roles of pores in tumor development and progression, there are certain areas of neglect. Since ECM is a complex biological process, studying single factors is technically challenging. Similarly, technical limitations indicate that some scaffolds are insufficiently defined or not measured rigorously. The following section will discuss the porous materials and their physiochemical understandings in biomedicine.
Figure 2. A. Schematic of the effect of pore size on the tumor. ECM pore size is one of the key factors in inducing ECM stiffness and ultimately leads to the downstream cascade in tumor progression. B. Migration of cells in the confined environment. Left panel: Shows the established and reported models of cell migration in the confined environment, including squeezing through micropores or protease degradation dependent on pore size confinement. The middle panel shows how cells modify the ECM by proteolytic degradation and migration. Right panel: it is a new model floated by wisdom et al., If the pore size is larger than 3 μm, it can still help migration through squeezing without protease degradation of ECM. This model of cell migration is a plasticity-mediated model independent of protease activity. If the membrane is smaller than 3 μm and has plasticity, cells progressively make their way by widening pores in the tissue matrix [Citation32]. (Image taken from the open-source paper with proper citation).
![Figure 2. A. Schematic of the effect of pore size on the tumor. ECM pore size is one of the key factors in inducing ECM stiffness and ultimately leads to the downstream cascade in tumor progression. B. Migration of cells in the confined environment. Left panel: Shows the established and reported models of cell migration in the confined environment, including squeezing through micropores or protease degradation dependent on pore size confinement. The middle panel shows how cells modify the ECM by proteolytic degradation and migration. Right panel: it is a new model floated by wisdom et al., If the pore size is larger than 3 μm, it can still help migration through squeezing without protease degradation of ECM. This model of cell migration is a plasticity-mediated model independent of protease activity. If the membrane is smaller than 3 μm and has plasticity, cells progressively make their way by widening pores in the tissue matrix [Citation32]. (Image taken from the open-source paper with proper citation).](/cms/asset/b0ae4677-895a-4d39-acd9-3952420b403d/tapx_a_2153624_f0002_oc.jpg)
2.3. History and techniques for biomaterials with controlled Porosity
2.3.1 Techniques for controlled porosity
Tissue engineering applications encompass 3D scaffolds to provide a suitable microenvironment for incorporating cells or growth factors to regenerate damaged tissues or organs. These scaffolds mimic the actual in vivo microenvironment where cells interact and behave according to the mechanical cues obtained from the surrounding 3D environment. Hence, the material properties of the scaffolds are vital in determining cellular response and fate [Citation7]. There have been rapid advances in bone tissue engineering with the development of porous, biocompatible, 3D scaffolds. Regardless of the application, the scaffold should be biocompatible and imitate both the physical and biological functions of the native ECM. The ECM provides a substrate with specific ligands for cell adhesion and physical support [Citation33].
Current tissue engineering techniques involve using controlled porous 3D templates to study the main biological functions. The development of new techniques enabled us to design customized biomaterials with controlled porosity. Currently, used techniques are listed in .
Table 1. Techniques used to design Biomaterials.
2.3.2 Factors affecting pore size
Under stiffer conditions, the pore size of the ECM is stiffer [Citation27]; there are several factors influencing pore size. The primary factor is the overabundance deposition of ECM protein [Citation28]. Collagen fiber diameter, concentration, gelation temperature, and pH are the main factors manipulated to tune the pore size of biomedical materials to study the biological implications of these matrix pores in tumorigenesis [Citation51] [Citation52,Citation53].
2.4. Pore size with biological response
Since pore size is an essential factor in ECM and governing biological processes, the design of 3D scaffolds with controllable multiscale pore size architecture currently represents a significant challenge. In recent years biomaterials have been manipulated to achieve optimal templates of scaffolds to study their impact on cell activities. Accurate control of the pore size is hugely demanded as the property of a material not to compromise the mechanical stabilities and properties of the scaffold [Citation54]. depicts the pore size of various scaffolds required for various cellular activities for their biomedical use.
Figure 3. Pore Size of various scaffolds required for various cellular activities. Metabolite exchange [Citation19,Citation55]; Angiogenesis [Citation21]; adipogenesis [Citation56,Citation57]; cell proliferation [Citation58]; Chondrogenesis [Citation59] [Citation60],; Hepatogenesis [Citation61]; Osteogenesis [Citation62,Citation63]; Proliferation [Citation64]; Skin regeneration [Citation65]; smooth muscle differentiation [Citation66]; Osteoblast [Citation67]; Odontoblast [Citation68]; BC Invasion [Citation69].
![Figure 3. Pore Size of various scaffolds required for various cellular activities. Metabolite exchange [Citation19,Citation55]; Angiogenesis [Citation21]; adipogenesis [Citation56,Citation57]; cell proliferation [Citation58]; Chondrogenesis [Citation59] [Citation60],; Hepatogenesis [Citation61]; Osteogenesis [Citation62,Citation63]; Proliferation [Citation64]; Skin regeneration [Citation65]; smooth muscle differentiation [Citation66]; Osteoblast [Citation67]; Odontoblast [Citation68]; BC Invasion [Citation69].](/cms/asset/462c904d-dfbf-43e8-bfd7-70deaede4289/tapx_a_2153624_f0003_oc.jpg)
2.5. Electrospun 3D scaffolds to mimic ECM
Various techniques have been used for the fabrication of 3D scaffolds. Approaches that mimic how tissues are formed in the body and their nanofibrous structure can be found in modular assembly and electrospinning methods. William Gilbert was the first to observe that liquid droplets will deform when electrically charged amber is brought closer to the droplet [Citation70]. This later became ‘Taylor Cone’ and was the first recorded electrospraying observation. Rayleigh, in 1897 was the first to observe the amount of charge required for droplet deformation, and the first fabricated artificial was patented in 1934 by Fromhals [Citation71].
Electrospinning is a versatile fiber fabrication technique, as demonstrated [Citation72]. It has become the most reliable way to fabricate continuous fibers scaled from nanometer to micrometer, much smaller than the fibers obtained by the conventional techniques, such as phase separation and self-assembly. The electrospun fibers have high porosities and a high surface-to-volume ratio [Citation73], and they can perfectly mimic the native ECM. Electrospun fibrous scaffolds have been investigated as potential scaffolds for biomedical applications [Citation74,Citation75].
When designing scaffolds for any tissue engineering application, the mean pore size is a significant consideration. Scaffolds must be permeable with interconnecting pores to facilitate cell growth, migration, and nutrient flow. A previous study demonstrated that permeability increases with increasing pore size due to a reduction in a specific surface area [Citation9]. If pores are too small, cell migration is limited, resulting in the formation of a cellular capsule around the edges of the scaffold. This, in turn, can limit the distribution of nutrients and removal of waste products resulting in necrotic regions within the construct.
Conversely, if pores are too large, there is a decrease in a specific surface area [Citation76]. Initial cell adhesion mediates all subsequent events such as proliferation, migration, and differentiation within the scaffold. As a result, the mean pore size within a scaffold affects cell adhesion and ensuing proliferation, migration, and infiltration. Therefore, maintaining a balance between the optimal pore size for cell migration and the specific surface area for cell attachment is essential [Citation33].
As the ECM matrix is a complicated structure with multivariate mechanophysical factors influencing the response of cells to ECM, the relationship between scaffold pore size and cell activity is not extensively studied or fully comprehended. As a result, there have been conflicting reports on the optimal pore size over the years. highlights the studies investigating the behavior of cells in response to matrix pore size, and summarizes the behavior of cells cultured on electrospun with varying pore sizes.
Figure 4. Timeline for the effect of varying matrix pore size of electrospun on the tumor cellular behavior. BC, Breast Cancer; LC, Lung Cancer; CRC, Colorectal cancer; FB, Fibroblast Cells.
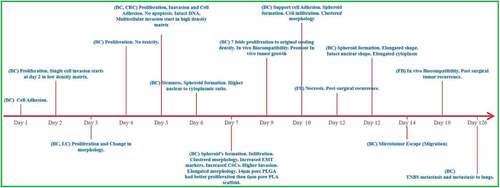
Table 2. The behavior of cells cultured on electrospun with varying pore sizes.
3. Biologically engineered ECM a way forward? Future perspectives
Since cancer is a combination of more than 100 diseases, ECM combines complex mechanophysical characteristics that provide tumor development and progression cues. ECM is an active 3D structure continuously undergoing remodeling to facilitate tissue homeostasis. It is a remarkably active 3D structure continuously undergoing remodeling to regulate tissue homeostasis [Citation96]. Our understandings are far from comprehension regarding the role of ECM in tumor progression. A better comprehension of ECM remodeling’s effect on cancer progression and development may improve understanding of tumor and therapeutic strategies.
Over the past several decades’ 2D culture models have been widely used for 3D tumor microenvironment in research. 2D culture models provide limited success [Citation97], failing most therapeutic drugs in clinical. One primary reason for the failure was to use the inappropriate culture model, which could not recapitulate the accurate tumor microenvironment [Citation96].
Compelling evidence suggests the use of 3D systems is the better biological and clinical model for understanding cancer. 3D culture systems provide more diverse phenotypes and heterogeneous growth and mimic a better microenvironment for gene and protein expression and metastatic and drug-resistant models. Recent years have seen more focus on understanding how the 3D microenvironment affects cell behavior and how it is different from the cell behavior studies in 2D culture models [Citation2,Citation98–100].
ECM is a 3D culture system comprised of various physical and biological cues that constitute its overall properties and effect on tissue homeostasis. Emerging technologies can better design and mimic the native tumor microenvironment for cancer studies [Citation101]. Such technologies/platforms can structure cancer-specific architecture with relevant stromal-cancer cell interaction, physiological cues, molecular signaling, vascular and immune components, and cell-Cancer interactions [Citation97] mimicking patient tumors.
Significant studies proposed that ECM stiffness promotes invasion; the limitation lies in the pore size is the current hypothesis [Citation102]. Pore size smaller than 5 μm (smaller than the epithelial cell nucleus) is a significant barrier and a limiting factor in cell migration/invasion. Tumor progression and ECM porosity is another area of research that may provide better insight into tumor progression and metastasis. It is reasoned that increased ECM deposition decreases the porosity of tumors [Citation103]. Porosity is the ability of the matrix to provide homeostasis [Citation104]. The effect of tumor pore size has yet to be evaluated alone.
Currently, tuneable engineered natural biomaterials are being used to investigate the role of ECM physical properties, especially pore size. However, the current 3D cultural systems are defective and do not mimic the optimal biophysical and biochemical signals for cell survival and growth. A greater comprehension of ECM pore size regulation and its function in the development of cancer progression may lead to improved understanding, drug discovery, and treatments [Citation96].
A summary highlighting the engineered system with pore properties, pore size and potential cellular behavior observed by various approaches, is presented in . However, until now, there is no single biomaterial accepted for all the applications, and there is no single material fabrication approach that can potentially fill all the aspects of tissue bioengineering scaffolds.
Table 3. Engineered system to manipulate ECM components for studying the tumor cell behavior.
3. Conclusions
Highly remodeled ECM provides a variety of physical characteristics that can either oppose or facilitate the moving cells. Tumor cells have enormous potential to adapt to that mechanobiological plasticity of ECM and migrate by well-defined locomotive strategies. ECM properties are essential, and they should be studied in 3D cultural models rather than 2D cultural models. ECM pore size governs the escape of human breast cancer (MDA-MB231 cells). More studies are needed to significantly comprehend the importance of pore size tumor metastasis and invasion. Tunable engineered cultural models can provide more accurate and fateful models to identify the role of ECM pore size in tumorigenesis.
Article highlights
Tumors can remodel ECM components.
ECM component collagen density defines matrix pore size.
Pore size dictates a variety of cell behavior.
ECM and pore size interaction can be important in understanding cancer and therapeutics.
Acknowledgments
This work was financially supported by the National Natural Science Foundation of China (32071332, 31971318, 21876205), the Key-Area Research and Development Program of Guangdong Province (2020B0101020001), the Shenzhen Science and Technology Innovation Project (JCYJ20170818101220860), CAS Key Laboratory of Biomedical Effects of Nanomaterials and Nanosafety (NSKF202015), the Shenzhen High-end Talent Project (KQRC2017-000244), and donated funding by TransEasy Medical Tech. Co., Ltd. (HX201910082).
Disclosure statement
No potential conflict of interest was reported by the author(s).
Additional information
Funding
References
- Sung H, Ferlay J, Siegel RL, et al. Global cancer statistics 2020: GLOBOCAN estimates of incidence and mortality worldwide for 36 cancers in 185 countries. CA: a Cancer Journal for Clinicians. 2021;71:209–22.
- Charras G, Sahai E. Physical influences of the extracellular environment on cell migration. Nat Rev Mol Cell Biol. 2014Dec; 15: 813–824 PubMed PMID: 25355506; eng
- Wei J-H, Haddad A, K-J W, et al. A CpG-methylation-based assay to predict survival in clear cell renal cell carcinoma. Nature Communications. 2015;6:1–11.
- Geiger B, KMJCSHpib Y. Molecular architecture and function of matrix adhesions. Cold Spring Harbor Perspectives in Biology. 2011;3:a005033.
- Akhmanova M, Osidak E, Domogatsky S, et al. Physical, spatial, and molecular aspects of extracellular matrix of in vivo niches and artificial scaffolds relevant to stem cells research. Stem Cells Int. 2015;2015:167025. PubMed PMID: 26351461; eng.
- Eiselt P, Yeh J, Latvala RK, et al. Porous carriers for biomedical applications based on alginate hydrogels. Biomaterials. 2000;21:1921–1927.
- Loh QL, Choong C. Three-dimensional scaffolds for tissue engineering applications: role of porosity and pore size. Tissue Eng Part B Rev. 2013;19:485–502. PubMed PMID: 23672709; eng.
- Salerno A, Di Maio E, Iannace S, et al. Tailoring the pore structure of PCL scaffolds for tissue engineering prepared via gas foaming of multi-phase blends. Journal of Porous Materials. 2012;19:181–188.
- O’Brien FJ, Harley BA, Waller MA, et al. The effect of pore size on permeability and cell attachment in collagen scaffolds for tissue engineering. Technology and Health Care: Official Journal of the European Society for Engineering and Medicine. 2007;15:3–17.
- Frantz C, Stewart KM, Weaver VM. The extracellular matrix at a glance. J Cell Sci. 2010;123:4195–4200. PubMed PMID: 21123617; eng.
- Henke E, Nandigama R, Ergun S. Extracellular matrix in the tumor microenvironment and its impact on cancer therapy. Front Mol Biosci. 2019;6:160. PubMed PMID: 32118030; PubMed Central PMCID: PMCPMC7025524.
- Lu P, Takai K, Weaver VM, et al. Extracellular matrix degradation and remodeling in development and disease. Cold Spring Harbor Perspectives in Biology. 2011;3:a005058.
- Mecham RP. Overview of extracellular matrix. Curr Protoc Cell Biol. 2012 Dec; 57 Chapter 10:Unit 10 1. 10.1002/0471143030.cb1001s57. PubMed PMID: 23208544.
- Trappmann B, Chen CS. How cells sense extracellular matrix stiffness: a material’s perspective. Curr Opin Biotechnol. 2013;24:948–953. PubMed PMID: 23611564; eng.
- Petrie RJ, Harlin HM, Korsak LIT, et al. Activating the nuclear piston mechanism of 3D migration in tumor cells. The Journal of Cell Biology. 2017;216:93–100.
- Guimarães CF, Gasperini L, Marques AP, et al. The stiffness of living tissues and its implications for tissue engineering. Nat Rev Mater. 2020;5:351–370. 2020 May 01.
- Gospodarowicz D, Greenburg G, Birdwell CR. Determination of cellular shape by the extracellular matrix and its correlation with the control of cellular growth. Cancer Res. 1978 Nov;38:4155–4171. PubMed PMID: 359133; eng.
- Caswell PT, TJTicb Z. Actin-based cell protrusion in a 3D matrix. Trends in Cell Biology. 2018;28:823–834.
- Oliviero O, Ventre M, PJAb N. Functional porous hydrogels to study angiogenesis under the effect of controlled release of vascular endothelial growth factor. Acta biomaterialia. 2012;8:3294–3301.
- Perez RA, Gjms M, E C. Role of pore size and morphology in musculo-skeletal tissue regeneration. Materials Science & Engineering. C, Materials for Biological Applications. 2016;61:922–939.
- Artel A, Mehdizadeh H, Chiu Y-C, et al. An agent-based model for the investigation of neovascularization within porous scaffolds. Tissue Engineering. Part A. 2011;17:2133–2141.
- Ma T, Li Y, Yang ST, et al. Effects of pore size in 3‐D fibrous matrix on human trophoblast tissue development. Biotechnology and Bioengineering. 2000;70:606–618.
- Yin Y, X-T H, Wang J, et al. Pore size-mediated macrophage M1-to-M2 transition influences new vessel formation within the compartment of a scaffold. Applied Materialstoday. 2020;18:100466.
- Yamada KM, Sixt M. Mechanisms of 3D cell migration. Nat Rev Mol Cell Biol. 2019 2019 Dec 01;20:738–752.
- Khatau SB, Bloom RJ, Bajpai S, et al. The distinct roles of the nucleus and nucleus-cytoskeleton connections in three-dimensional cell migration. Sci Rep. 2012;2:488. PubMed PMID: 22761994; PubMed Central PMCID: PMCPMC3388469. eng.
- Renkawitz J, Kopf A, Stopp J, et al. Nuclear positioning facilitates amoeboid migration along the path of least resistance. Nature. 2019 Apr;568:546–550. PubMed PMID: 30944468; PubMed Central PMCID: PMCPMC7217284. eng
- Yang YL, Leone LM, Kaufman LJ. Elastic moduli of collagen gels can be predicted from two-dimensional confocal microscopy. Biophys J. 2009 Oct 7;97:2051–2060. PubMed PMID: 19804737; PubMed Central PMCID: PMCPMC2756380. eng.
- Swift J, Ivanovska IL, Buxboim A, et al. Nuclear lamin-A scales with tissue stiffness and enhances matrix-directed differentiation. Science (New York, NY). 2013 Aug 30;341:1240104. PubMed PMID: 23990565; PubMed Central PMCID: PMCPMC3976548. eng.
- Harada T, Swift J, Irianto J, et al. Nuclear lamin stiffness is a barrier to 3D migration, but softness can limit survival. J Cell Biol. 2014 Mar 3;204:669–682. PubMed PMID: 24567359; PubMed Central PMCID: PMCPMC3941057. eng.
- Irianto J, Pfeifer CR, Bennett RR, et al. Nuclear constriction segregates mobile nuclear proteins away from chromatin. Mol Biol Cell. 2016 Dec 15;27:4011–4020. PubMed PMID: 27798234; PubMed Central PMCID: PMCPMC5156542. eng.
- Wolf K, Te Lindert M, Krause M, et al. Physical limits of cell migration: control by ECM space and nuclear deformation and tuning by proteolysis and traction force. J Cell Biol. 2013 Jun 24;201:1069–1084. PubMed PMID: 23798731; PubMed Central PMCID: PMCPMC3691458. eng.
- Wisdom KM, Adebowale K, Chang J, et al. Matrix mechanical plasticity regulates cancer cell migration through confining microenvironments. Nat Commun. 2018 Oct 8;9:4144. PubMed PMID: 30297715; PubMed Central PMCID: PMCPMC6175826. eng.
- Ma Z, Kotaki M, Inai R, et al. Potential of nanofiber matrix as tissue-engineering scaffolds. Tissue Engineering. 2005;11:101–109.
- Guarino V, Ambrosio L. Electrofluidodynamics: exploring a new toolbox to design biomaterials for tissue regeneration and degeneration. Future Med. 2016; 11(12). doi:10.2217/nnm-2016-0108 .
- Guarino V, ME AL, Part H. Journal of Engineering in Medicine. Temperature-driven processing techniques for manufacturing fully interconnected porous scaffolds in bone tissue engineering. Proceedings of the Institution of Mechanical Engineers. Part H, Journal of Engineering in Medicine. 2010;224:1389–1400.
- Liu X, Pxjb M. Phase separation, pore structure, and properties of nanofibrous gelatin scaffolds. Biomaterials. 2009;30:4094–4103.
- Granados‐Hernández MV, Serrano‐Bello J, Montesinos JJ, et al. In vitro and in vivo biological characterization of poly (lactic acid) fiber scaffolds synthesized by air jet spinning. Journal of Biomedical Materials Research. Part B, Applied Biomaterials. 2018;106:2435–2446.
- Hou Q, Grijpma DW, Feijen JJB. Porous polymeric structures for tissue engineering prepared by a coagulation, compression moulding and salt leaching technique. Biomaterials. 2003;24:1937–1947.
- Yoon JJ, Song SH, Lee DS, et al. Immobilization of cell adhesive RGD peptide onto the surface of highly porous biodegradable polymer scaffolds fabricated by a gas foaming/salt leaching method. Biomaterials. 2004;25:5613–5620.
- Kim TK, Yoon JJ, Lee DS, et al. Gas foamed open porous biodegradable polymeric microspheres. Biomaterials. 2006;27:152–159.
- Seck TM, Melchels FP, Feijen J, et al. Designed biodegradable hydrogel structures prepared by stereolithography using poly (ethylene glycol)/poly. Journal of Controlled Release: Official Journal of the Controlled Release Society. 2010 D, L-lactide)-based resins;148:34–41.
- Elomaa L, Teixeira S, Hakala R, et al. Preparation of poly (ε-caprolactone)-based tissue engineering scaffolds by stereolithography. Acta biomaterialia. 2011;7:3850–3856.
- Yan M, Tian X, Peng G, et al. Hierarchically porous materials prepared by selective laser sintering. Materials & Design. 2017;135:62–68.
- Xiong Z, Yan Y, Zhang R, et al. Fabrication of porous poly (L-lactic acid) scaffolds for bone tissue engineering via precise extrusion. Scripta Materialia. 2001;45:773–779.
- Soo Kim B, Ji Kim E, Suk Choi J, et al. Human collagen‐based multilayer scaffolds for tendon‐to‐bone interface tissue engineering. Journal of Biomedical Materials Research. Part A. 2014;102:4044–4054.
- Sultana N, Wang M. Fabrication of HA/PHBV composite scaffolds through the emulsion freezing/freeze-drying process and characterisation of the scaffolds. Journal of Materials Science. Materials in Medicine. 2008;19:2555.
- Chiu Y-C, Larson JC, Isom JA, et al. Generation of porous poly (ethylene glycol) hydrogels by salt leaching. Tissue Engineering. Part C, Methods. 2010;16:905–912.
- De Nardo L, Bertoldi S, Cigada A, et al. Preparation and characterization of shape memory polymer scaffolds via solvent casting/particulate leaching. Journal of Applied Biomaterials & Functional Materials. 2012;10:119–126.
- Intranuovo F, Gristina R, Brun F, et al. Plasma modification of PCL porous scaffolds fabricated by solvent‐casting/particulate‐leaching for tissue engineering. Plasma Processes and Polymers. 2014;11:184–195.
- Gu Z, Fu J, Lin H, et al. Development of 3D bioprinting: from printing methods to biomedical applications. Asian J Pharm Sci. 2020;15:529–557. 2020 Sep 01.
- Mickel W, Münster S, Jawerth LM, et al. Robust pore size analysis of filamentous networks from three-dimensional confocal microscopy. Biophys J. 2008 Dec 15;95:6072–6080. PubMed PMID: 18835899; PubMed Central PMCID: PMCPMC2599830. eng.
- Raub CB, Unruh J, Suresh V, et al. Image correlation spectroscopy of multiphoton images correlates with collagen mechanical properties. Biophys J. 2008 Mar 15;94:2361–2373. PubMed PMID: 18065452; PubMed Central PMCID: PMCPMC2257909. eng.
- Yang YL, Motte S, Kaufman LJ. Pore size variable type I collagen gels and their interaction with glioma cells. Biomaterials. 2010Jul; 31: 5678–5688 PubMed PMID: 20430434; eng
- Chanes-Cuevas OA, Perez-Soria A, Cruz-Maya I, et al. Macro-, micro-and mesoporous materials for tissue engineering applications. AIMS Materials Science. 2018;5:1124–1140.
- Madden LR, Mortisen DJ, Sussman EM, et al. Proangiogenic scaffolds as functional templates for cardiac tissue engineering. Proceedings of the National Academy of Sciences of the United States of America. 2010;107:15211–15216.
- Murphy CM, O’Brien FJ. O”Brien FJJCa, migration. Understanding the effect of mean pore size on cell activity in collagen-glycosaminoglycan scaffolds. Cell Adhesion & Migration. 2010;4:377–381.
- Mandal BB, Scjb K. Osteogenic and adipogenic differentiation of rat bone marrow cells on non-mulberry and mulberry silk gland fibroin 3D scaffolds. Biomaterials. 2009;30:5019–5030.
- Rnjak-Kovacina J, Wise SG, Li Z, et al. Tailoring the porosity and pore size of electrospun synthetic human elastin scaffolds for dermal tissue engineering. Biomaterials. 2011;32:6729–6736.
- Griffon DJ, Sedighi MR, Schaeffer DV, et al. Chitosan scaffolds: interconnective pore size and cartilage engineering. Acta biomaterialia. 2006;2:313–320.
- Uematsu K, Hattori K, Ishimoto Y, et al. Cartilage regeneration using mesenchymal stem cells and a three-dimensional poly-lactic-glycolic acid (PLGA) scaffold. Biomaterials. 2005;26:4273–4279.
- Wang M, Pei H, Zhang L, et al. Hepatogenesis of adipose-derived stem cells on poly-lactide-co-glycolide scaffolds: in vitro and in vivo studies. Tissue Engineering. Part C, Methods. 2010;16:1041–1050.
- Kasten P, Beyen I, Niemeyer P, et al. Porosity and pore size of β-tricalcium phosphate scaffold can influence protein production and osteogenic differentiation of human mesenchymal stem cells: an in vitro and in vivo study. Acta Biomaterialia. 2008;4:1904–1915.
- Kuboki Y, Jin Q, Takita HJJ. Geometry of carriers controlling phenotypic expression in BMP-induced osteogenesis and chondrogenesis. The Journal of Bone and Joint Surgery. American Volume. 2001;83:S105–S115.
- Takahashi Y, BS TY, Edition P. Effect of the fiber diameter and porosity of non-woven PET fabrics on the osteogenic differentiation of mesenchymal stem cells. Journal of Biomaterials Science. Polymer Edition. 2004;15:41–57.
- Yannas I, Lee E, Orgill DP, et al. Synthesis and characterization of a model extracellular matrix that induces partial regeneration of adult mammalian skin. Proceedings of the National Academy of Sciences of the United States of America. 1989;86:933–937.
- Cho S-W, Kim I-K, Lim SH, et al. Smooth muscle-like tissues engineered with bone marrow stromal cells. Biomaterials. 2004;25:2979–2986.
- Murphy CM, Haugh MG, O’Brien FJ. The effect of mean pore size on cell attachment, proliferation and migration in collagen–glycosaminoglycan scaffolds for bone tissue engineering. Biomaterials. 2010 2010 Jan 01;31:461–466.
- Conde CM, Demarco FF, Casagrande L, et al. Influence of poly-L-lactic acid scaffold’s pore size on the proliferation and differentiation of dental pulp stem cells. Brazilian Dental Journal. 2015;26:93–98.
- Geiger F, Rüdiger D, Zahler S, et al. Fiber stiffness, pore size and adhesion control migratory phenotype of MDA-MB-231 cells in collagen gels. PloS one. 2019;14:e0225215.
- Kleivaitė V, RJArj M. Electrospinning-100 years of investigations and still open questions of web structure estimination. AUTEX Research Journal. 2018;18:398–404.
- Anton F. Process and apparatus for preparing artificial threads. Google Patents; 1934.
- Li D, YJAm X. Electrospinning of nanofibers: reinventing the wheel?. Advanced Materials. 2004;16:1151–1170.
- Liang D, Hsiao BS, BJAddr C. Functional electrospun nanofibrous scaffolds for biomedical applications. Advanced Drug Delivery Reviews. 2007;59:1392–1412.
- Nisbet DR, Forsythe JS, Shen W, et al. A review of the cellular response on electrospun nanofibers for tissue engineering. Journal of Biomaterials Applications. 2009;24:7–29.
- Agarwal S, Wendorff JH, Greiner AJP. Use of electrospinning technique for biomedical applications. Polymer. 2008;49:5603–5621.
- IJCm Y. Tissue regeneration by use of collagen-glycosaminoglycan copolymers. Clinical Materials. 1992;9:179–187.
- Girard YK, Wang C, Ravi S, et al. A 3D fibrous scaffold inducing tumoroids: a platform for anticancer drug development. PloS one. 2013;8:e75345.
- Foroni L, Vasuri F, Valente S, et al. The role of 3D microenvironmental organization in MCF-7 epithelial–mesenchymal transition after 7 culture days. Exp Cell Res. 2013;319:1515–1522. 2013 Jun 10.
- Vashisth P, Sharma M, Nikhil K, et al. Antiproliferative activity of ferulic acid-encapsulated electrospun PLGA/PEO nanofibers against MCF-7 human breast carcinoma cells. 3 Biotech. 2015;5:303–315. 2015 Jun 01.
- Sahoo SK, Panda AK, Labhasetwar V. Characterization of porous PLGA/PLA microparticles as a scaffold for three dimensional growth of breast cancer cells. Biomacromolecules. 2005 2005 Mar 01;6:1132–1139.
- Wang Y, Qian J, Liu T, et al. Electrospun PBLG/PLA nanofiber membrane for constructing in vitro 3D model of melanoma. Mater Sci Eng C. 2017;76:313–318. 2017 Jul 01.
- Russo V, Tammaro L, Di Marcantonio L, et al. Amniotic epithelial stem cell biocompatibility for electrospun poly (lactide-co-glycolide), poly (ε-caprolactone), poly (lactic acid) scaffolds. Materials Science & Engineering. C, Materials for Biological Applications. 2016;69:321–329.
- Almajhdi FN, Fouad H, Khalil KA, et al. In-vitro anticancer and antimicrobial activities of PLGA/silver nanofiber composites prepared by electrospinning. J Mater Sci. 2014;25:1045–1053. 2014 Apr 01.
- Aboutalebi Anaraki N, Roshanfekr Rad L, Irani M, et al. Fabrication of PLA/PEG/MWCNT electrospun nanofibrous scaffolds for anticancer drug delivery. Journal of Applied Polymer Science. 2015;132.
- Sampath M, Lakra R, Korrapati P, et al. Curcumin loaded poly (lactic-co-glycolic) acid nanofiber for the treatment of carcinoma. Colloids Surf B Biointerfaces. 2014;117:128–134. 2014 May 01.
- Ye C, Zhao J, Zheng Y, et al. Preparation of poly (lactic‐co‐glycolic acid)‐based composite microfibers for postoperative treatment of tumor in NIR I and NIR II biowindows. Macromolecular Biosciences. 2018;18:1800206.
- Prieto E, Mojares E, Cortez J, et al. Electrospun nanofiber scaffolds for the propagation and analysis of breast cancer stem cells in vitro. Biomedical Materials (Bristol, England). 2021;16:035004.
- Rabionet M, Yeste M, Puig T, et al. Electrospinning PCL scaffolds manufacture for three-dimensional breast cancer cell culture. Polymers. 2017;9:328.
- Saha S, Duan X, Wu L, et al. Electrospun fibrous scaffolds promote breast cancer cell alignment and epithelial-mesenchymal transition. Langmuir. 2012;28:2028–2034. PubMed PMID: 22182057; eng.
- Obayemi JD, Danyuo Y, Dozie-Nwachukwu S, et al. PLGA-based microparticles loaded with bacterial-synthesized prodigiosin for anticancer drug release: effects of particle size on drug release kinetics and cell viability. Mater Sci Eng C. 2016;66:51–65. 2016 Sep 01.
- Jusu SM, Obayemi JD, Salifu AA, et al. Drug-encapsulated blend of PLGA-PEG microspheres: in vitro and in vivo study of the effects of localized/targeted drug delivery on the treatment of triple-negative breast cancer. Sci Rep. 2020;10:14188. PubMed PMID: 32843673; eng.
- Kim T-E, Kim CG, Kim JS, et al. Three-dimensional culture and interaction of cancer cells and dendritic cells in an electrospun nano-submicron hybrid fibrous scaffold. International Journal of Nanomedicine. 2016;11:823.
- Xie X, Zheng X, Han Z, et al. A biodegradable stent with surface functionalization of combined‐therapy drugs for colorectal cancer. Advanced Healthcare Materials . 2018;7:1801213.
- Domura R, Sasaki R, Okamoto M, et al. Comprehensive study on cellular morphologies, proliferation, motility, and epithelial–mesenchymal transition of breast cancer cells incubated on electrospun polymeric fiber substrates. Journal of Materials Chemistry. B. 2017;5:2588–2600.
- Permlid AM, Roci P, Fredlund E, et al. Unique animal friendly 3D culturing of human cancer and normal cells. Toxicology in Vitro . 2019;60:51–60.
- Sonbol HS. Extracellular matrix remodeling in human disease. J Microsc Ultrastruct. 2018Jul-Sep; 6: 123–128 PubMed PMID: 30221137; eng
- Belgodere JA, King CT, Bursavich JB, et al. Engineering breast cancer microenvironments and 3D bioprinting [Review]. Frontiers in Bioengineering and Biotechnology. 2018; 6: English 2018 May 24 10.3389/fbioe.2018.00066.
- Rubashkin MG, Ou G, Vmjb W. Deconstructing signaling in three dimensions. Biochemistry. 2014;53:2078–2090.
- Caswell PT, Zech T. Actin-based cell protrusion in a 3D matrix. Trends Cell Biol. 2018Oct; 28: 823–834 PubMed PMID: 29970282; PubMed Central PMCID: PMCPMC6158345. eng
- Ruprecht V, Monzo P, Ravasio A, et al. How cells respond to environmental cues - insights from bio-functionalized substrates. J Cell Sci. 2017 Jan 1;130:51–61. PubMed PMID: 27856508; eng.
- Hirt C, Papadimitropoulos A, Muraro MG, et al. Bioreactor-engineered cancer tissue-like structures mimic phenotypes, gene expression profiles and drug resistance patterns observed “in vivo”. Biomaterials. 2015 Sep;62:138–146. PubMed PMID: 26051518; eng
- Micalet A, Moeendarbary E, Ujabs C, et al. 3D in vitro models for investigating the role of stiffness in cancer invasion. ACS Biomaterials Science & Engineering. 2021. 10.1021/acsbiomaterials.0c01530.
- Haeger A, Krause M, Wolf K, et al. Cell jamming: collective invasion of mesenchymal tumor cells imposed by tissue confinement. Biochimica et biophysica acta. 2014;1840:2386–2395.
- Qazi TH, Mooney DJ, Duda GN, et al. Biomaterials that promote cell-cell interactions enhance the paracrine function of MSCs. Biomaterials. 2017;140:103–114.
- Boyden S. THE CHEMOTACTIC EFFECT OF MIXTURES OF ANTIBODY AND ANTIGEN ON POLYMORPHONUCLEAR LEUCOCYTES. J Exp Med. 1962;115:453–466.
- Kleinman HK, McGarvey ML, Liotta LA, et al. Isolation and characterization of type IV procollagen, laminin, and heparan sulfate proteoglycan from the EHS sarcoma. Biochemistry. 1982;21:6188–6193.
- Barcellos-Hoff M, Aggeler J, Ram T, et al. Functional differentiation and alveolar morphogenesis of primary mammary cultures on reconstituted basement membrane. Development (Cambridge, England). 1989;105:223–235.
- Le Berre M, Aubertin J, Piel M. Fine control of nuclear confinement identifies a threshold deformation leading to lamina rupture and induction of specific genes. Integr Biol. 2012;4:1406–1414.
- Liu YJ, Le Berre M, Lautenschlaeger F, et al. Confinement and low adhesion induce fast amoeboid migration of slow mesenchymal cells. Cell. 2015 Feb 12;160:659–672. PubMed PMID: 25679760; eng.
- Davidson PM, Denais C, Bakshi MC, et al. Nuclear deformability constitutes a rate-limiting step during cell migration in 3-D environments. Cell Mol Bioeng. 2014 Sep 1;7:293–306. PubMed PMID: 25436017; PubMed Central PMCID: PMCPMC4243304. eng.
- Holle AW, Govindan Kutty Devi N, Clar K, et al. Cancer cells invade confined microchannels via a self-directed mesenchymal-to-amoeboid transition. Nano Lett. 2019; 19, 2280–2290. 2019 Apr PubMed PMID: 30775927; eng
- Nath B, Raza A, Sethi V, et al. Understanding flow dynamics, viability and metastatic potency of cervical cancer (HeLa) cells through constricted microchannel. Sci Rep. 2018;8:17357. 2018 Nov 26.
- Wolf K, Alexander S, Schacht V, et al. Collagen-based cell migration models in vitro and in vivo. Semin Cell Dev Biol. 2009;20:931–941. 2009 Oct 01.
- Qian W, Zhang Y, Chen W. Capturing cancer: emerging microfluidic technologies for the capture and characterization of circulating tumor cells. Small (Weinheim an der Bergstrasse, Germany). 2015;11:3850–3872.
- Chen MB, Whisler JA, Fröse J, et al. On-chip human microvasculature assay for visualization and quantification of tumor cell extravasation dynamics. Nat Protoc. 2017 May;12:865–880. PubMed PMID: 28358393; PubMed Central PMCID: PMCPMC5509465. eng
- Coughlin MF, Kamm RD. The use of microfluidic platforms to probe the mechanism of cancer cell extravasation Advanced Healthcare Materials . 2020;9:1901410.
- Naderi S, Khayat Zadeh J, Mahdavi Shahri N, et al. Three-dimensional scaffold from decellularized human gingiva for cell cultures: glycoconjugates and cell behavior. Cell J. 2013; Summer 15: 166–175. PubMed PMID: 23862119; PubMed Central PMCID: PMCPMC3712778. eng
- Leiva MC, Garre E, Gustafsson A, et al. Breast cancer patient-derived scaffolds as a tool to monitor chemotherapy responses in human tumor microenvironments. Journal of Cellular Physiology. 2021;236:4709–4724.
- Rijal G, Li W. A versatile 3D tissue matrix scaffold system for tumor modeling and drug screening. Sci Adv. 2017Sep; 3: e1700764 PubMed PMID: 28924608; PubMed Central PMCID: PMCPMC5597314. eng
- Ehrmann RL, Gey GO. The growth of cells on a transparent gel of reconstituted rat-tail collagen2. Jnci. 1956;16:1375–1403.
- Raub CB, Unruh J, Suresh V, et al. Image correlation spectroscopy of multiphoton images correlates with collagen mechanical properties. Biophys J. 2008;94:2361–2373. 2008 Mar 15.
- Raub CB, Suresh V, Krasieva T, et al. Noninvasive assessment of collagen gel microstructure and mechanics using multiphoton microscopy. Biophys J. 2007;92:2212–2222. 2007 Mar 15.
- Gobeaux F, Mosser G, Anglo A, et al. Fibrillogenesis in dense collagen solutions: a physicochemical study. J Mol Biol. 2008;376:1509–1522. 2008 Mar 07.
- Pinner S, Sahai E. Imaging amoeboid cancer cell motility in vivo. J Microsc. 2008Sep; 231: 441–445 PubMed PMID: 18754999; eng
- Laronda M, Rutz A, Xiao S, et al. A bioprosthetic ovary created using 3D printed microporous scaffolds restores ovarian function in sterilized mice. Nat Commun. 2017;8:15261.
- Lewis DM, Tang V, Jain N, et al. Collagen fiber architecture regulates hypoxic sarcoma cell migration. ACS Biomaterials Science & Engineering. 2018;4:400–409.