Abstract
The present research dealt with the successful viability and practicality of Pleurotus eryngii packed bed column for Pb(II) ions biosorption. To achieve the aim of the project, the impact of different parameters including flow rate, initial concentration of Pb(II) ions and bed height were optimized. The column models, i.e.,Thomas and Bed Depth Service Time (BDST) were investigated to assess the column efficiency towards entrapping targeted ion. The adsorption capacity, rate constant and correlation coefficient related to each model for column sorption were also calculated. The adsorption capacity enhanced by increasing the bed height and decreasing initial Pb(II) metal ion concentration along with the flow rate. The maximum Thomas model adsorption (entrapping) capacity was obtained 3.30 mg g−1 for initial concentration of 20 mg L−1 at a constant flow rate of 1 ml min−1, bed height of 3 cm and pH 7. The experimental results implied and affirmed the suitability of the P. eryngii fungal biosorbent for Pb(II) ion biosorption with its nature being favourable, efficient and environment friendly.
1. Introduction
Heavy metals discharged from untreated industrial and municipal effluents are amongst the main sources of water pollution in the recent past. The acute toxicity and carcinogenicity associated with metal ions discharged into the aquatic system pose a serious threat to the environment (Vilvanathan & Shanthakumar, Citation2017). These heavy metals find their way into the micro-environment, the aquatic flora and fauna and in turn into the food chain exhibiting direct health effects on humans. They are classified as toxic materials due to their non-biodegradability and bioaccumulation tendency in living organisms (Klapiszewski, Bartczak, Szatkowski, & Jesionowski, Citation2017).
Special attention needs to be paid to the metal ions, particularly Pb(II) ions. The lower concentration of Pb(II) ion is essential for the promotion of many biosynthesis reactions in the human body and is also playing an important role as micronutrients for animal and plants, yet it is harmful at a higher level of concentration (Tchounwou, Yedjou, Patlolla, & Sutton, Citation2012; Singh, Gautam, Mishra, & Gupta, Citation2011). According to the standards of WHO, the maximum permissible limit of Pb(II) ions in aqueous system is 0.05 mg L−1 (WHO, Citation2008). If this level of concentration exceeds in water, it causes anaemia and stomach intestinal distress (Sravya, Citation2015). Therefore, growing problem of the presence of metal ions in aqueous system has encouraged scientists to seek alternative methods for the removal of this type of contamination.
To date several techniques such as chemical precipitation, electrocoagulation, flocculation, membrane filtration, adsorption and ion exchange treatment have been employed for the removal of toxic metal ions from aqueous system (Gunatilake, Citation2015); but due to high cost, dispose off issues, formation of by-products, reliability of the technique and its environmental impacts these techniques facing confinements (Barakat, Citation2011).
In this scenario, it is important to opt for an economically feasible and effective treatment method which is free from these limitations and is able to translate the need of removal of heavy metals in terms of eco-friendly approach. Recently, a state-of-the-art technique ‘bioremediation’ is introduced for the removal of heavy metals from wastewater involving the use of natural biomaterials (macro and microbial biomass / adsorbents) (Abdel-Raouf & Abdul-Raheim, Citation2017).
Our previous reported work has demonstrated the potential use of P. eryngii biomass to enhance bioremediation of heavy metals by batch experiments (Amin, Talpur, Balouch, & Afridi, Citation2017). However, the results of batch study may not be directly being applied for the field applications in wastewater treatments.
In this research work, P. eryngii, a fungal biomass commonly called as ‘white-rot fungus,’, is studied here for its biosorptive potential. Considering its relative adequacy and abundant availability, this mycelium can be a potential resource of natural biosorbent. Hence, the aim of present work is to investigate the entrapping capacity of P. eryngii fungal biomass as a biosorbent for Pb(II) ions removal from aqueous system in continuous mode in a fixed-bed column. The biosorption capacity of P. eryngii was investigated under pH controlled conditions by varying the flow rate, initial Pb(II) ion concentration and bed height. The breakthrough data were analyzed using Thomas and Bed Depth Service Time (BDST) models.
2. Material and methods
2.1. Preparation of fungal biomass (P. eryngii)
For sorption studies, P. eryngii mycelium using as biosorbent (biomass) was procured from Edible Fungi Institute, Shanghai Academy of Agricultural Sciences, China. The detail of P. eryngii biomass preparation is described in our previously reported work (Amin, Talpur, Balouch, Surhio, & Bhutto, Citation2015).
2.2. Preparation of standard solutions for understudy sorbate (Pb-metal)
To acquire 1000 mg L−1 stock solution of pure Pb(II) metal, 4.577 g analytical grade salt of Pb(NO3)2 were dissolved in ultra-pure water (conductivity 0.05 μS cm−1) in a volumetric flask. By appropriate dilution of stock solution with ultra-pure water, working standards of metal were prepared freshly from certified and internal standards.
The pH of working solutions were adjusted to the desired values according to subsequent experimental design with 0.1 M NaOH or 0.1 M HCl solutions.
2.3. Continuous (packed column) biosorption experiment
Continuous flow sorption experiments were conducted in a mini glass column (5 mm × 120 mm).
The bottom of the column filled with glass wool followed by a small layer of glass beads at the column base in order to provide a uniform inlet flow of the solution into the column. A known quantity of biosorbent material was packed in the column to yield the desired bed height of the sorbent. At the top of the column, the influent (Pb) solution (20, 30 mg L−1) was pumped through the packed column (1, 2 and 3 cm), at flow rates of 1, 3 and 5 ml min−1, using a peristaltic pump. Samples were collected from the exit of the column at regular time intervals and analyzed for residual concentration at room temperature.
2.4. Analytical instrumentation
The pH metre (InoLab-WTW GmbH; Weilheim, Germany) with glass electrode and an internal reference electrode were used for pH measurements.
Quantification of Pb(II) ions before and after the sorption was carried out by Varian AA 20 spectra atomic absorption spectrometer (Mulgrave, Victoria, Australia) equipped with cathode lamp of respective elements. The process parameters of Flame-AAS are given in Table .
Table 1. Conditions of F-AAS for Pb(II) ions determination.
The flow rate for Pb(II) ion solutions during column studies were optimized by Eyela peristaltic pump (Tokyo Rikakikai Co., Ltd. Japan) and flow system was made of PTFE 0.5 mm i.d.
2.5. Analysis of column data
The total quantity of sorbate (Pb-metal) biosorbed in the column (mad) is calculated from the area above the breakthrough curve multiplied by the flow rate (Foo, Lee, & Hameed, Citation2013).
Dividing the amount of sorbate (mad) by the amount of biosorbent (M) leads to the uptake capacity (Q) of the biomass.
The total amount of Pb(II) ions sent to the column calculated from the following Equation (1):(1)
Where C0 is the inlet Pb(II) ions concentration (mg L−1); F is the volumetric flow rate (mL h−1); and te locate the exhaustion time (h).
Total removal (%) with respect to flow volume can be calculated from the ratio of Pb(II) ions mass adsorbed (mad) to the total amount of Pb(II) ions sent to the column (mtotal) as follows (2):(2)
2.6. Statistical analysis
All continuous experiments were performed in triplicates and the results are presented as means of the triplicates. The linear regression coefficients R2 were determined using Microsoft Excel (Microsoft Inc., WA, U.S.A.) to test the adequacy and accuracy of the linearized forms of the model equations.
2.7. Column models
In process applications, a packed bed column is an effective process for cyclic sorption / desorption, as it makes the best use of the concentration difference known to be a driving force for pollutant sorption and results in a better quality of the effluent (Negrea, Lupa, Ciopec, & Negrea, Citation2011). Two frequently used models i.e., Thomas and BDST were used to analyze the compatibility of experimental data of the tested metals.
2.7.1. Thomas model
The design of an adsorption packed column requires the determination of column parameters such as adsorption capacity and the kinetic parameters (Al Dwairi, Omar, & Al-Harahsheh, Citation2015). The Thomas model can be implemented to analyze the breakthrough curves and adsorption capacity for each sorbent. The linearized form of Thomas model expressed by Equation (3):(3)
where C0 and C are the inlet and the effluent solute concentrations at any time t (m); kTh is the Thomas model constant (mL m−1 mg−1); q0 is the maximum solid-phase concentration of solute (mg g−1); and M is the total mass of the adsorbent (g). The model constants kTh and q0 can be determined from slope and intercept of a plot of ln[(C0/C) – 1] against t, respectively.
2.7.2. BDST model
The BDST model used to predict the column performance of any bed length, if data for some depths are known. It relates the service time of a fixed bed with the height of adsorbent in the bed, hence with its quantity (Babu, Krishnan, & Singh, Citation2010). The measurement of sorbent quantity is more precise than the determination of the respective volume. Therefore, sorbent quantity is being preferably used, instead of the bed height.
The linear form of BDST model expressed as Equation (4):(4)
where t is the service time (h), N0 is the adsorption capacity (mg cm−3), Z is the height of column (cm), Cb is the breakthrough sorbate concentration (mg L−1), ϑ is the linear velocity (cm h−1) and Ka is the rate constant (L mg−1 h−1) at time t.
A plot of t vs. bed depth (Z) should yield a straight line and use to evaluate N0 and Ka, respectively. Application of the BDST model requires specification of the breakthrough time, which was selected arbitrarily.
3. Results and discussion
3.1. Column study
3.1.1. Effect of flow rate
The adsorption columns were operated with different flow rates (1, 3 and 5 mL min−1) at a fixed concentration and bed height until no further Pb removal was observed. The breakthrough curve for a column were determined by plotting the ratio of the Ce/C0 (Ce and C0 are the Pb(II) concentrations of effluent and influent, respectively) against time, as shown in Figure . The column performed well at a lower flow rate (1 mL min−1). Earlier breakthrough (Ce/C0 = 0.05) and exhaustion times were achieved, when the flow rate was increased from 3 to 5 mL min−1. This was due to a decrease in the residence time, which restricted the contact of Pb(II) solution to the fungal biomass. At higher flow rates, the Pb(II) ions did not have enough time to diffuse into the pores of the fungal biomass and they exited the column before equilibrium occurred (Mobasherpour, Salahi, & Asjodi, Citation2014). In literature, similar behaviour of breakthrough for Pb(II) by immobilized fungus Phanerochaete chrysosporium was reported by Pakshirajan and Swaminathan (Citation2010).
3.1.2. Effect of initial Pb(II) ions concentration (Thomas model)
The adsorption breakthrough curves obtained by changing initial Pb(II) concentration (20 and 30 mg L−1) at 1 mL min−1 flow rate as given in Figure . As estimated, a decrease in Pb(II) concentration gave a later breakthrough curve; because the treated volume was greatest at the lowest transport due to a decreased diffusion coefficient or mass transfer coefficient.
Figure 2. Breakthrough curves for different Pb(II) ion concentration (flow rate: 1 mL min−1; bed height: 2 cm).
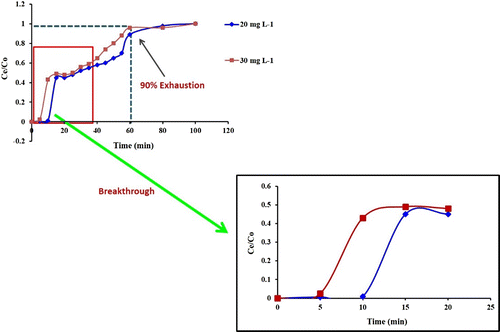
Breakthrough time (Ce/C0 = 0.05) came up after 9.0 min at 20 mg L−1 initial Pb concentration while the breakthrough time was 3.0 min at 30 mg L−1. The breakthrough time decreased with increasing Pb concentration as the binding sites became more quickly saturated in the column.
The Thomas model kinetic parameters calculated from equation 3 reveals a good fit of the experimental data at all concentration examined (Table ). The correlation coefficients greater than 0.905 showed that the external and internal diffusions were not the rate limiting step. The rate constant (kTh) decreased with increasing Pb(II) concentration which indicates that the mass transport resistance increases due to the driving force between Pb(II) concentration and fungal biomass (Negrea et al., Citation2011). Comparable outcomes were also explained by Han et al. (Citation2006) for the biosorption of Cu(II) and Pb(II) ions from aqueous solution by chaff in a fixed-bed column.
Table 2. The Thomas and BDST model parameters for the biosorption of Pb(II) on P. eryngii fungal biomass.
3.1.3. Effect of bed height (BDST model)
The accumulation of Pb(II) ions in a fixed-bed column is dependent on the quantity of biosorbent inside the column. To study the effect of bed height on Pb(II) retention, fungal biomass of three different bed heights. As depicted by Figure , the breakthrough time varies with bed height. The breakthrough time decreased with a decreasing bed depth from 3 to 1 cm, as binding sites were restricted at low bed depths. At low bed depth, the Pb(II) ions do not have enough time to diffuse into the surface of the biomass, and a reduction in breakthrough time occurs. Conversely, with an increase in bed depth, the residence time of Pb(II) solution inside the column was increased, allowing the Pb(II) ions to diffuse deeper into the fungal biomass (Babu et al., Citation2010). The related affinity was also reported in the literature (Biswas & Mishra, Citation2015).
Figure 3. (a) Breakthrough curves for different bed height (flow rate: 1 mL min−1; initial Pb(II) ion concentration: 20 mg L−1) and (b) Bed depth service time plot for the adsorption of Pb(II) ions by fungal biomass in column.
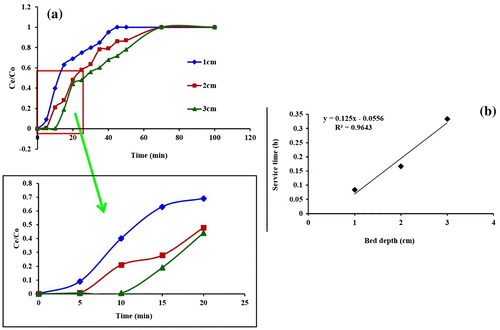
A plot of service time vs. bed depth, at a flow rate of 1 mL min−1 (Figure 4–60b) was linear. The correlation coefficient value (R2=0.964) indicated the validity of the BDST model for the present system. The values of BDST model parameters are presented in Table calculated Equation (4).
The value of Ka characterizes the rate of transfer from the fluid phase to the solid phase. If Ka is large, even a short bed will avoid breakthrough, but as Ka decreases a progressively deeper bed is required to avoid breakthrough. From this model and its obtained constants, feasibility of column structure and its performance were evaluated using sufficient concentration and flow rate for sorption of Pb(II) ions onto fungal biomass without further experimental analysis and data.
3.2. Comparison of Pb(II) ions sorption capacity during batch and column mode
Table represents a good sorption capacity by column, then previously conducted batch mode (Amin et al., Citation2017). It is generally attributed to the more time interaction between the biosorbent and the sorbate surface with the column.
Table 3. Comparison of Pb(II) ions sorption capacity.
3.3. Application of column on real contaminated water samples
The removal of toxic ions from real water samples is a link between laboratory and commercial application of column. In this study, all the laboratory adsorption column conditions developed (flow rate, bed height) are transferred to the normal level. The results obtained are given in Table suggest that Pb(II) ions removed successfully below the permissible limits of WHO drinking water standards.
Table 4. Removal of Pb(II) ions from real water samples via column method.
4. Conclusion
In this work, continuous biosorption operation in a fixed-bed column was performed for Pb(II) ions biosorption by P. eryngii fungal biomass. Thomas and BDST models were used to successfully evaluate the column performance. The breakthrough curves of the continuous flow systems (columns) were adequately fitted with the Thomas model, which provided an estimation of the dynamic adsorption capacities of 3.30 mg g−1 for the 3 cm bed height at a constant flow rate of 1 ml min−1 and pH 7. In the fluidized bed system, increase in the solution flow rate decreased the breakthrough time due to the decrease in the contact time between the adsorbate (Pb) and the adsorbent (biomass); besides, at low flow rates the metal ions had a sufficient contact time to occupy the spaces within the particles. An increase in concentration of Pb(II) ions caused a decrease in the operating time due to the driving force between Pb(II) concentration and fungal biomass. Likewise, it was found that increase in the bed depth of fungal biomass increased the breakthrough time. This study proved that P. eryngii fungal biomass has good potential to be used in sorption of Pb(II) ions from aqueous solution in the fixed bed column. Although the removal of Pb(II) ions in the presence of other ions has not been studied, this is suggested for future scope of work for other researchers.
References
- Abdel-Raouf, M.S., & Abdul-Raheim, A.R.M. (2017). Removal of heavy metals from industrial waste water by biomass-based materials: A review. Journal of Pollution Effects & Control, 5, 180. doi:10.4172/2375-4397.1000180
- Amin, F., Talpur, F.N., Balouch, A., Surhio, M.A., & Bhutto, M.A. (2015). Biosorption of fluoride from aqueous solution by white-rot fungus Pleurotus eryngii ATCC 90888. Environmental Nanotechnology, Monitoring & Management, 3, 30–37.10.1016/j.enmm.2014.11.003
- Amin, F., Talpur, F.N., Balouch, A., & Afridi, H.I. (2017). Eco-efficient fungal biomass for the removal of Pb(II) ions from water system: A sorption process and mechanism. International Journal of Environmental Research, 11, 315–325. doi:10.1007/s41742-017-0029-z
- Babu, J.P.E., Krishnan, R., & Singh, M. (2010). Bed depth service time model for the biosorption of reactive red dye using the Portunus sanguinolentus shell. Asia-Pacific Journal of Chemical Engineering, 5, 791–797.
- Barakat, M.A. (2011). New trends in removing heavy metals from industrial wastewater. Arabian Journal of Chemistry, 4, 361–377.10.1016/j.arabjc.2010.07.019
- Biswas, S., & Mishra, U. (2015). Continuous fixed-bed column study and adsorption modeling: Removal of lead ion from aqueous solution by charcoal originated from chemical carbonization of rubber wood sawdust. Journal of Chemistry, 1–9. doi: 10.1155/2015/907379
- Al Dwairi, R., Omar, W., & Al-Harahsheh, S. (2015). Kinetic modelling for heavy metal adsorption using Jordanian low cost natural zeolite (fixed bed column study). Journal of Water Reuse and Desalination, 231–238. doi: 10.2166/wrd.2014.063
- Foo, K.Y., Lee, L.K., & Hameed, B.H. (2013). Preparation of tamarind fruit seed activated carbon by microwave heating for the adsorptive treatment of landfill leachate: A laboratory column evaluation. Bioresource Technology, 133, 599–605.10.1016/j.biortech.2013.01.097
- Gunatilake, S.K. (2015). Methods of removing heavy metals from industrial wastewater. Journal of Multidisciplinary Engineering Science Studies, 1, 12–18.
- Han, R., Zhang, J., Zou, W., Xiao, H., Shi, J., & Liu, H. (2006). Biosorption of copper(II) and lead(II) from aqueous solution by chaff in a fixed-bed column. Journal of Hazardous Materials, 262–268.10.1016/j.jhazmat.2005.10.019
- Klapiszewski, L., Bartczak, P., Szatkowski, T., & Jesionowski, T. (2017). Removal of lead(II) ions by an adsorption process with the use of an advanced SiO2/lignin biosorbent. Polish Journal of Chemical Technology, 19(1), 48–53.
- Mobasherpour, I., Salahi, E., & Asjodi, A. (2014). Research on the batch and fixed-bed column performance of red mud adsorbents for lead removal. Soil and Water, 2, 83–96.
- Negrea, A., Lupa, L., Ciopec, M., & Negrea, P. (2011). Experimental and modelling studies on As(III) removal from aqueous medium on fixed bed column. Chemical Bulletin of Politehnica, 56, 89–93.
- Pakshirajan, K., & Swaminathan, T. (2010). Biosorption of lead by the immobilised fungus Phanerochaete chrysosporium in a packed bed column. International Journal of Environmental Technology and Management, 12, 214–228.10.1504/IJETM.2010.031529
- Singh, R., Gautam, N., Mishra, A., & Gupta, R. (2011). Heavy metals and living systems: An overview. Indian Journal of Pharmacology, 43, 246–253.10.4103/0253-7613.81505
- Sravya, M. (2015). Lead exposure in children through water and soil. Environmental Management & Risk Assessment (PH 560). Retrieved from http://digitalcommons.wku.edu/pubh_560/1
- Tchounwou, P.B., Yedjou, C.G., Patlolla, A.K., & Sutton, D.J. (2012). Heavy metal toxicity and the environment. In A. Luch (Eds.), Molecular, Clinical and Environmental Toxicology. Experientia Supplementum (Vol. 101). Basel: Springer.
- Vilvanathan, S., & Shanthakumar, S. (2017). Continuous biosorption of nickel from aqueous solution using Chrysanthemum indicum derived biochar in a fixed-bed column. Water Science & Technology, 76, 1895–1906. doi:10.2166/wst.2017.289
- WHO. (2008). Guidelines for Drinking-Water Quality [electronic resource]: Incorporating 1st and 2nd Addenda, Vol. 1, Recommendations. Geneva: Author.