Abstract
Due to the scarcity of water resources and the harmful effects of sewage on water bodies, water purification becomes an important issue. The application of ultrasonic waves is a novel technique developed for water purification. This technology works as an advanced method of oxidation, contributing to the elimination of various types of contaminants. The basic principle of ultrasound is based on the destruction of both bacterial cells and difficult to- degrade organics. A brief overview of water purification methods, application of ultrasound, ultrasound waves theory and the benefits and drawbacks of using ultrasound waves in water purification will be discussed in this article. In addition, the role of ultrasound waves in the degradation of various organic contaminants, microbial pollutant disinfection, and other pollutants removal.
1. Introduction
The word “water quality” defines water conditions, including water's physical, chemical and microbiological properties. Generally these properties contribute to its suitability and the water's quality for a specific application. Water suitable for domestic use (drinking water) must meet specific requirements. The most important condition is that it must be safe to drink.
Unfortunately, all water resource aren't sufficient for drinking purposes, and some treatment techniques must be used to make the water quality for domestic use, so we are facing in generating adequate drinking water (Ackah, Agyemang, & Anim, Citation2011; Tiwari, Citation2011; Zhang, Jiang, & Ou, Citation2011; Zhang, Zhang, & Fan, Citation2009).
The main water treatment process involves flocculation, sedimentation and media filtration to eliminate colloidal and suspended solids, exchange of ions, carbon adsorption and membrane processes to extract dissolved solids, and finally a microbial inactivation disinfection that is often carried out by chlorination, ozonation and ultraviolet radiation (UV) (Tansel, Citation2008).
Any drinking water system that faces some purification problems and applications, such as high cost, ineffective in removing some contaminants, operational problems, and secondary toxic pollutants generation (Gaya & Abdullah, Citation2008). Key factors in the development and implementation of water treatment systems were the identification of new rarer pollutants, the adoption of new water quality standards and costs. Different studies have been carried out to remove this constraint by applying groundbreaking techniques such as Biosorption (Mittal, Mittal, Malviya, & Gupta, Citation2010; Saleh & Gupta, Citation2014; Gupta, JainbImran, Chandr, & Agarwal, Citation2002, Gupta, Jain, Nayak, Agarwal, & Shrivastava, Citation2011, Gupta, Nayak, & Agarwal, Citation2015; Gupta & Saleh, Citation2013) photocatalytic oxidation (Gupta et al., Citation2011; Rajendran et al., Citation2016; Saleh and Gupta, Citation2012a; Saravanan et al., Citation2013), advanced oxidation with nanocoposite membranes (Saleh & Gupta, Citation2011; Saravanan et al., Citation2015a, Citation2015b); semi-conductor catalysts, reverse osmoses, and magnetic purification (Ambashta & Sillanpaa, Citation2010; Chong, Jin, Chow, & Saint, Citation2010; Khani, Rofouei, Arab, Gupta, & Vafaei, Citation2010; Kumar, Chauhan, Goyalb, Kesri, & Behari, Citation2015; Saravanan, Gupta, Mosquera, & Gracia, Citation2014; Saravanan et al., Citation2016; Zhang et al., Citation2019). Ultrasound is one such recent technique found to be substantially effective in the treatment of wastewater (Nikolopoulos, Igglessi-Markopoulou, & Papayannakos, Citation2006). With the discovery of the piezoelectric effect by the Curies (Gogate, Citation2007), the base for the current generation of ultrasound was developed. Thornycrof and Sidney (Citation1895) first described and documented the cavitation phenomenon. Harvey and Loomis (Citation1928) published their first study on the use of ultrasound as a disinfectant in the late 1920s. At the early of 1945 the understanding of the cavitation phenomenon evolved in tandem with significant developments in electronic circuitry and transducers (i.e., machines which transform electrical to mechanical signals and vice versa). Many studies have focused on the use of ultrasound to extract organic xenobiotics from water since 1990 (Entezari, Petrier, & Devidal, Citation2003; Kruus, Burk, Entezari, & Otson, Citation1997; Petrier, Jeunet, Luche, & Reverdy, Citation1992). As a result, the application of power ultrasound to chemical processes has been rapidly expanded, a topic which has become known as “sonochemistry” (Drakopoulou, Terzakis, Fountoulakis, Mantzavinos, & Manios, Citation2009). Consequently, the aim of this chapter is to examine the advantages and disadvantages of ultrasound technology, applications; disinfection mechanism also briefly discussed.
2. Sound theory
The sound can be represented as a mechanical energy transmitted in gas, liquid or solid form by pressurized waves. This general definition includes all sound forms, including audible sound, seismic (infrasound) low-frequency waves, and ultrasound. Ultrasound is a cyclic sound stress with a frequency greater than the human hearing upper limit (). It is about 20 kilohertz (20,000 hertz) (Leighton, Citation1994).
In a transmission medium such as a gas, liquid or solid, sound can be represented as mechanical energy transmitted by pressure waves. Sound can therefore be defined as a mechanical energy. This overall definition encompasses all forms of noise, including visible, low frequency seismic and ultrasound waves. Ultrasound is cyclic sound pressure, which reaches the upper limit of human hearing (); the frequency is about 20 kilohertz.
US could be generate by two main techniques, the first is called “magnetostrictive” in which electrical energy is converted to mechanical energy (or vibration) with a magnetic coil attached to vibrating piece like nickel and Terfenol-D. The second is piezoelectric technique, the electrical energy is converted to high frequency electric energy with piezoelectric crystals attached to the vibrating piece (sonotrode, probe or horn) (Gogate, Citation2007; Pilli et al., Citation2011).
The majority of modern ultrasonic devices rely on piezoelectric materials transducers. These materials react with a small change in size to the application of an electric potential on opposite sides. That's the opposite of the piezoelectric effect. The crystal translates electrical energy into mechanical vibration (sound) energy when it alternates at high frequencies. High frequency sound (ultrasound) is produced at sufficiently high alternating potential. A lower frequency ultrasound can cause chemical changes for a system as a result of acoustically induced cavity (Suslick, Citation1994; Zhou, Lau, Wu, & Shung, Citation2011). Ultrasound (US) wave application with or above a frequency of 20,000 Hz called “sonication”. This frequency is above the sonic range (20 Hz to 20 kHz) at which humans are able to listen and below the mega-sonic zone (>600 kHz) (Deymier, Vasseur, Khelif, & Raghavan, Citation2001; Wong, Citation2002).
The motion of the molecules in the setting in which the wave spreads (Bello, Angelis, & Domingos, Citation2005) is transmitted energy by US waves. Ultrasound has ranges of approximately 10 to 10 cm in successive waves of compression. The molecular dimension is not comparable (). Based on this fact, the ultrasound's chemical effects can not result from direct sound contact with molecular species (Chong et al., Citation2010; Suslick, Citation1994).
3. Cavitation
Cavitation is the formation, growth and eventual dissolution of cavities releasing enormous amounts of energy from the conversion of kinetic energy from the motion of fluids into heating of bubble contents. Through generating hydroxyl radicals and also hydrogen peroxide, it also produces heavy oxidizing conditions (Hammitt, Citation1980). The chemical behavior is similar to Advanced Process Oxidation (Warade, Gaikwad, Sapkal, & Sapkal, Citation2016). Cavitation is a complex process that depends on the volume and structure of bubbles and cavities over a continuous time period. The pacing is in line with milliseconds. After the fluid has passed into regions that have reached critical pressure, sudden implosions in bubbles and cavities are much smaller than milliseconds in time, resulting in regional rises in pressure in several parts of the area. The pressures in the fluid can locally reach hundreds of mega-Pascal, even thousands. The hydrodynamic, electrical, acoustic, chemical, thermal and even electrostatic effects related with cavitation bubble implosions are common (Gogate, Citation2007).
Cavitation occurs if a threshold of 0.06–0.1 MPa exceeds acoustic pressure amplitude for distilled water, and when driving frequencies of about 20 kHz are used (Eskin & Eskin, Citation2014).
Increased Acoustic Pressure means that thousands of micro bubbles develop in a transition to an evolving cavity network. During the rarefaction cycle these bubbles were extended and collapsed rapidly throughout ultrasound compression, producing high-speed (300–1000 m/s) jets and, in principle, when the bubble collapsed, pressure could reach between 500 and 10,000 atm and temperature range 3000–5000 K (Tzanakis, Lebon, Eskin, & Pericleous, Citation2017). Hydroxyl (OH−) and hydrogen (H+) radicals would therefore be produced and advanced oxidization processes would be produced in these extreme conditions, with oxidized organic dissolved compounds. The outcome of OH− and HO2 radical recombination is hydrogen peroxide (H2O2) (Doulah, Citation1977).
In addition to, chemical and thermal processes are of major role in microbial disinfection (Mason, Joyce, Phull, & Lorimer, Citation2003). The main mechanism is the beginning of turbo, which causes vortexes, shock waves and high shear stresses, throughout the whole fluid (Doulah, Citation1977). Cavitation mechanisms in water disinfection (Gogate, Citation2007; Gogate & Pandit, Citation2008; Koval, Shevchuk, & Starchevsky, Citation2011). The selection of frequency and result, of the size of cavitative bubbles, is very important both for physical and chemical effects as ultrasound is a mechanical wave. Specifically, the low frequency driving frequencies (i.e., 20 kHz), associated with large and rapidly collapsing bubbles are the main cause of mechanical effects such as surface deformation, erosion and decomposition while MHz driving frequencies, are associated with a shorter life cycle of small cavitation bubbles which affect the MHz range (Mason, Cobley, Graves, & Morgan, Citation2011).
3.1. Types of acoustic cavitation
Acoustic cavitation includes the formation, growth, pulsation and collapse of the microblasts of fluids under ultrasound waves of high intensity. There are two main types: transient and (or controlled) stable acoustic cavitation. It is a mechanism that separates “stable” and “transient” cavitation from the collapse of a cavitation bubble. Noltingk and Neppiras (Citation1950) differentiated a particular kind of cavitation whereby small bubbles expand to many times their original size in strong enough sound areas and subsequently collapse rapidly. The rising process includes an isothermal first approach and an adiabatic collapse stage, so that the bubble concentrates acoustic energy. The collapse mechanism depends on the amount of energy produced by the bubble, the environmental situation and the liquid that surrounds the bubble. In contrast to the less energetic' stable' cavitation, Flynn (Citation1964) differentiated this so‐called' random' cavitation. One of two things happens in the case of balanced cavitation. The bubble contents may not completely dissolve into the surrounding fluid because it collapses, leaving the remaining bubble as the “seed” for a bubbles that oscillates and with the transfer of following sound waves will grow in size. The other option is that the remaining gas of the bubble, which is now filled with air, is enough to float to the liquid surface. That's how the degassing takes place. This refers to the way cavitations bubble with small amplifiers when the frequency of the ultrasound is comparatively low. This can lead to intense flow from or into the bubble, which is called acoustic micro-streaming (Joyce, Mason, Phull, & Lorimer, Citation2002), through the periodic expansion and shrinkage of cavitation bubbles. Stable cavitation may also occur if a vapor or gas fills the cavitation bubble and has been released into a cavitation bubble by the differential pressure. The gas or steam contained inside the bubble is a shield for implosion protection (Lauterborn & Ohl, Citation1997).
In transient cavitation, the resulting cavity is called “transient cavitation” which is useful for ultrasound clearing if however the bubble is sufficiently large to contain energy and inertia to resolve the resistance of any vapor or gas it may contain. In a temporary breakdown, energy in the bubble is focused to produce a range of potential local effects including a gas hot spot (Noltingk & Neppiras, Citation1950; Suslick, Hammerton, & Cline, Citation1986) and gas shocks (Flynn, Citation1964), both of which can potentially produce free radicals and other chemical reactive species in bubbles air, which can produce the luminescence in its sound (Cap luminescence) (Capocelli, Prisciandaro, Lancia, & Musmarra, Citation2013; Eskin & Eskin, Citation2014; Tzanakis et al., Citation2017). Low-energy/density sound waves turn the ultrasonic frequencies into collapsing energy/density lamps, causing intermittent sound cavitation. Intermittent acoustic cavitation in more sensitive substrates can cause damaging surface erosion (Joyce et al., Citation2002; Laborde, Bouyer, Caltagirone, & Gérard, Citation1998).
Noltingk and Neppiras (Citation1950) have described a certain cavitation form, where small bubbles develop to their original size, followed by a rapid collapse, in sufficiently strong sound fields. The first isothermal and adiabatic approximation stage of the growth stage is to focus the acoustic energy. The bubble concentrates the acoustic energy. Flynn (Citation1964) further distinguished this so-called' transient' cavitation from the less.
4. Advantages and disadvantages
Ultrasonic cavitation was thoroughly studied during the last decade. Although the technology has been shown to be an advanced, effective and small-scale method for water and wastewater treatment, commercial application remains a challenge because of the process ' high demand for energy. One of the most modern methods to handle harmful contaminants in water, Sono-chemistry has been found.
Ultrasonic technology has the following benefits (Buchholz, Tanis, Macomber, & Farris, Citation1998; Crittenden, Trussell, & Tchobanglouse, Citation2004; Manson & Lorimer, Citation2002) to be achieved:
Simple modular model, low capital cost
Easy upgrade of conventional treatment unit
No ultrasound device additives and not byproducts produced
No anticipated environmental concerns
High performance, given the form of microorganism, of the different types of pathogens from water and wastewater inaction.
Natural organic materials oxidation
US performance can also be increased by increasing turbidity or suspended solids in the processes of chemical degradation• No output of traditional by-products of disinfection (CDMs, etc.)
High synergy effect/efficiency improvements
US On the other hand it should be pointed out that, up to now, the design features of most devices have been developed following empirical data: theoretical criteria are still being developed in order to rationalize the design of these systems as well as their application to larger volumes.
Many of the benefits of this technology in general could be pointed out as follows:
Also evolving development criteria:
Rise in water turbidity
Energy consumption
Maintenance/replacement of the ultrasound probe
Lack of available disinfection power
More operating costs are linked to the maintenance and/or replacement of instruments which continue to be damaged by ultrasonic activity itself.
5. Ultrasound applications
The use of ultrasound as an advanced oxidation method for water treatment has been shown to be of great interest in recent years. A variety of mechanical, physical, chemical and biological modifications occur in a fluid due to acoustic cavitation 45 due to U.S. requirements of irradiation. Some of this important technology could be summarized as:
5.1. Membrane filtration
In drinking water treatment, membrane, a term that applies to a number of different processes using artificial membranes to isolate chemicals, has been recognized as the primary technology for separating pollutants from contaminated sources, thus purifying original waters (Ng et al., Citation2010). Membrane processes play a vital role in complementing conventional water treatment systems to meet ever more rigorous drinking water requirements and maintain public trust (Cho, Amy, & Pellegrino, Citation1999; Nakatsuka, Nakate, & Miyano, Citation1996). Membrane fouling is one of this technology's main drawbacks. Reducing membrane fouling and flux decline has been a critical challenge in membrane applications that can affect the efficiency of water production, membrane existence and operating costs (Lamminen, Walker, & Weaver, Citation2004). In recent years, combining membrane processes with ultrasound (US) is a revolutionary membrane fouling control strategy that can increase membrane activity (Borea et al., Citation2018; Chen, Weavers, & Walker, Citation2006; Kyllönen, Pirkonen, & Nyström, Citation2005).
During membrane filtration, the concept of using US relies primarily on US cavitation effect. Like any sound wave, ultrasound is propagated through a sequence of waves of compression and rarefaction (decompression) caused by the medium's molecules. The rarefaction process will surpass the attractive forces of the liquid molecules at a sufficiently high energy, and cavitation bubbles (empty, gas-and/or vapor-filled bubbles) are formed. As these bubbles form and burst under different pressures, they release energy and cause vibrations that loosen particles on the membrane surface without compromising the efficiency of the procedure. Through splitting the polarization concentration and cake layer on the membrane surface, it increases the flux (Lamminen et al., Citation2004).
The effects of speed, power strength, feed properties, membrane properties, cross-flow rate, temperature and pressure are determined by several parameters. Consideration should be given to the integrity of the membrane during ultrasound treatment. Ultrasonically enhanced membrane filtration is still not commonly advertised. The main reasons for a break-through prevention are the growth of stagnation of membrane filtration transducer technology and membrane erosion control (Kyllönen et al., Citation2005).
Kobayashi, Chai, and Fujii (Citation1999) found that the US increased permeation by increasing the coefficient of mass transfer of water through ceramic membrane microfiltration as well as organic membrane ultrafiltration. US irradiation studies have established prevention of concentration polarization and membrane fouling due to stripping of the cake layer, producing a less compressible fouling sheet, and reducing concentration of solute close to the membrane surface (Kobayashi et al., Citation1999; Muthukumaran et al., Citation2004). American cleaning processes are divided into four distinct pathways: acoustic streaming, microstreaming, microstreaming, and microjets (Lamminen et al., Citation2004). The collapse of cavitation bubbles does not require just acoustic streaming. Ultrasonic waves cause massive movement of water to and from the surface of the membrane, producing forces to push and pull away from the surface. Micro streaming happens as bubbles oscillate in size and with an effective range of bubble diameter produce significant shear forces. The phenomenon of microstreamers is described as the cavitation bubbles that move in ribbon-like paths as these bubbles fly vigorously around the surface of the membrane. When translating to antinodes, they appear to coalesce and scour off particles. Micro jets occur when two asymmetric cavitation bubbles collapse simultaneously or only one bubble collapses near a solid interface and produces a powerful jet of water that scores particles away from the surface of the membrane. Several scientists have demonstrated the efficacy of US-associated membrane cleaning to increase permeates flux and extend the lifetime of the membrane. The US recovered flux with forward flushing on a 0.2 mm nylon membrane filled with an effluent recorded by Li, Sanderson, and Jacobs (Citation2002) from the Kraft paper factory. Fouled membrane supported by US backwashing is more rigorous than traditional backwashing (Matsumoto, Miwa, Nakao, & Kimura, Citation1996).
In some studies ultrasound damage on the membrane surface has been observed (Juang & Lin, Citation2004). In other research, the membranes were even damaged by repeated use of ultrasound (Borea et al., Citation2018; Lamminen et al., Citation2004). Use of the sporadic U.S. procedure will likely be less energized than continuing US radiation and U.S. pulsed layers of bovine serum albumin (BSA) from a cross-flow mf are effectively removed (Yuk & Youm, Citation2003). Nevertheless, the use of continuous application in polymer solutions was more effective than intermittent usage (Simon, Gondrexon, Taha, Cabon, & Dorange, Citation2000).
5.2. Application of ultrasound for turbidity and total suspended solid reduction
Turbidity is a major physical feature of air. The presence of scattered hanging solids such as clay, silt, algae and other microorganisms, organic material and minute particle materials can be detected (EPA, Citation2014). A variety of methods have been used in the water treatment process for reducing turbidity, Total Suspended Solid (TSS) filtration and slow-filtration, ultrafiltration and coagulation/flocking (EPA, Citation2014). American procedure for turbidity reduction (TSS) and advanced oxidation treatment (Manson & Lorimer, Citation2002) has been extensively studied. The US efficiency may improve by increasing turbidity or suspended solids (Manson & Lorimer, Citation2002) compared to conventional methods that are negatively affected by the suspension of effluent solids. Mutiarani and Trisnobudi (Citation2009) studied the use of ultrasound in TSS elimination and found that removal efficiency in the different conditions was extremely high. At a frequency of 28 kHz, 60 Watts of power, the most turbidity reduction (76%) was achieved. At 27,2 kHz at 30 seconds, water turbidity decrease 4 times over (39 NTU) at 27,2 kHz (Stefan & Balan, Citation2011). The results show that TSD reduction was increased at 30 min ultrasound rate, but was unpredictability from 60 to 120 min of irradiation at all power densities for all energy densities. Chua, AdulLatif, and Ibrahim (Citation2009) have studied the impact of power density on TSS reduction. The efficiency was 0,024 W/cm3, 120 min with 84% of output and 60% with a minimum percentage reduction at 0,06 W/cm3 for 60 min. The most effectual result was reducing turbidity of raw water by a precursor to coagulation from 10,3 NTU to 1,48 NTU and by 15 s ultrasonic irradiation at 40 kHz 60 W (Liang, Nan, & He, Citation2009).
5.3. Applications of ultrasound in organic pollutants degradation
POPs are the specific environment polluting group, through high toxicity, persistence and bioaccumulation ability (Ozonek, Citation2012). Persistent organic pollutants (Ozonek). Several research studies have demonstrated applications for the decomposition of different biological pollutants, such as chlorinated hydrocarbon, pesticides, phenol, explosives, such as TNT and esters (Kot-Wasik, Dabrowska, & Namiesnik, Citation2003; Psillakis, Goula, Kalogerakis, & Mantzavinos, Citation2004; Torres, Pétrier, Combet, Carrier, & Pulgarin, Citation2008). It takes minutes to hours to complete degradation (Kot-Wasik et al., Citation2003). Psillakis et al. (Citation2004) examined the degradation of polyaromatic hydrocarbons (naphthalenic and acenaphthylene) by water using ultrasonic machines at initial concentrations of 150, 300 and 450 μg/l at 20 and 40 °C, applied energy of 45, 75 and 150 W and ultrasound fréquences of 24 and 80 °C and found that a complete degradation of water was possible at initial concentrations of 150, 300 and 450 µg/l.
Manousaki, Psillakis, Kalogerakis, and Mantzavinos (Citation2004) investigated the decomposition from ultrasound effects of sodium dodecylbanzenesulfonate (SDBS) under different experimental conditions. Due to the increased temperature, starting solvent concentration and decreasing power and frequency the SDBS degradation has been shown. In addition, the work into the transformation mechanism showed that SDBS decomposed primarily at the bubble-liquid interface and, to a lesser degree, in the liquid bulk by radical reactions. The municipal wastewater phenol is one of the most concentrated toxins (Entezari et al., Citation2003). Their presence in soil, rivers and drinking water attracts attention from the general public (Entezari et al., Citation2003).
Guo and Feng (Citation2008) investigated the evaluation of ultrasonic bisphenol A (BPA) degradation in aqueous solution; suggested that BPA had been degraded by hydroxyl radical advanced oxidation with a frequency of 20 kHz in the presence of CCl4.
Torres et al. (Citation2008) have studied the removal effects of bisphenol A (BPA) on ultrasonic action under different experimental conditions. They showed that the best performance for 118 μmol/L BPA was 300 kHz, 80 W for 90 min, and oxygen as a saturated gas for this solution. Hydroxyl radical reaction is also the main mechanism for phenol removal.
Application of a hybrid advanced oxidation technique (sonoelectro-Fenton process) for the degradation of herbicides from aqueous solutions was studied by Oturan et al. (Citation2008). They suggested that the decomposition of 4, 6-dinitro-o-cresol (DNOC) and 2, 4-dichlorophenoxyacetic acid (2, 4-D) herbicides by sonoelectro-Fenton process is due to the following contributions: (i) enhanced mass transfer rate of reactants towards cathode, (ii) additional generation of OH by sonolysis, and (iii) pyrolysis of organics due to cavitation generated by ultrasound irradiation.
The application of ultrasound in removal of textile dyes was investigated by several researchers. Ghaedi et al. (Citation2015) studied the methylene blue simultaneous removal of Methylene blue (MB) and Safranin-O (SO), as most together hazardous dye in wastewater, from aqueous solution by ultra-sonic-assisted adsorption onto the developed adsorbent i.e., Fe3O4 NPs. Fe3O4 NPs and they concluded that the maximum dyes removal was obtained at pH 6.0, adsorbent dosage 0.01 g, MB concentration, SO concentration 10 and 20 mg/L and contact time 2 min. The very rapid removal of dyes makes this novel adsorbent as a promising tool for wastewater management applications.
Ultrasound-assisted removal of Auramine-O (AO) dye from aqueous solutions using ZnS:Cu nanoparticles loaded on activated carbon (ZnS:Cu-NP-AC) as an adsorbent (Asfaram, Ghaedi, Agarwal, Tyagi, & Gupta, Citation2015). The maximum adsorption capacity of AO by ZnS:Cu-NP-AC was determined as 183.15 mg g−1, suggesting a highly promising potential for ZnS:Cu-NP-AC to be used as a new adsorbent.
The extraction of organic matter from wastewater had been studied by Naseri, Vaezi, Mahvi, Nabizadeh, and Haddadi (Citation2006). Suspended BOD has been removed (about 100 percent), but in some cases soluble BOD has been increased. The most effective organic extraction is done at 130 kHz in 60 min from secondary effluent (Naseri et al., Citation2006). Naffrechoux, Chanoux, Petrier, and Suptil (Citation2000) have investigated the treatment of raw waste by son-|UV (combined sonication and UV irradiation) and found a significant reduction in COD following four hours of sonu-vius treatment.
5.4. Application of ultrasound for water disinfection process
The decontamination of water is critical to the destruction of humanly ill microorganisms. Chlorination, chlorinated dioxide, chloramines, ozone and ultraviolet light (Pozos, Scow, & Wuertz, Citation2004) form part of the main water treatment process. The main forms of chlorine commonly used in the disinfection process are chlorine gas and its derivatives sodium hypo-chlorite (NaClO), or calcium hypo-chlorite (Ca (ClO)2) (Winward, Avery, Stephenson, & Jefferson, Citation2008). All forms of chlore are, however, highly corrosive and poisonous to the foot. DBPs are a serious problem caused by the reaction of the organic matter and the disinfectant, which is the result of the disinfection of the organic material (Liu & Chiou, Citation2005).
Many variables that influence the species and concentrations of DBPs; the form and concentration of the disinfectants used, the size, temperature and pH and when the disinfectant is used in the water treatment system (Petrier, Jiang, & Lamy, Citation1998). The release into water of these toxic DBPs produces major environmental concerns. In this regard, a large number of studies have been carried out to remove the limitation by using the ultrasound (US) method, which constitutes a fairly advanced technique for traditional processing technologies (Dhermendra, Tiwari, & Behari, Citation2008; Khan, Shen, & Lin, Citation2006; Kumar et al., Citation2015). This drawback emphasized the need to explore alternative and new treatment technologies (Gopal, Tripathy, Bersillon, & Dubey, Citation2007).
In the late 20s, Harvey and Loomis (Citation1928) published their first report on the use of ultrasound as a disinfectant. In the early to mid-1970s, numerous researchers explored the use of ultrasound as a disinfectant because of its ability to decrease bacterial sporal heat resistance (Burleson, Murray, & Pollard, Citation1975; Dahi, Citation1976). Ultrasound desinfection is now well documented to be related to cavitation phenomena (Zou & Tang, Citation2019). Many scientists studied the use of US water treatment techniques, alone or joined with other procedures for disinfection (Wu, 2017). US disinfection procedure has also been extensively examined for their parameters ().
Figure 3. Cavitation event and consequences induced by power US in liquid media. (a) Stable cavitation (non-collapsing): bubble expands (low p) and contracts (high p) alternately, oscillating and existing for many acoustic cycles. (b) Transient cavitation: cavity/bubble increases in size, and eventually collapses at an unstable size, creating immense local high T and p up to 5000 K and 2000 atm (1 atm = 1.01 × 105 Pa) (Wu, 2017).
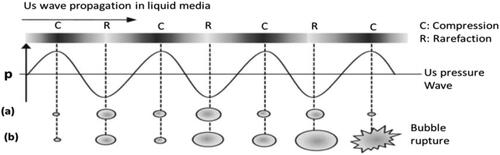
5.4.1. Disinfection mechanisms during ultrasound irradiation
Extensive investigations were conducted on US disinfection techniques (Burzio et al., Citation2020; Doulah, Citation1977; Gogate, Citation2007; Zou & Tang, Citation2019). The energy generated during American irradiation in several combined mechanisms based on acoustic cavitations can inactive microorganism. The primary mechanism suggested is the disruption of turbulences that produces vortexes, shock waves and high shear stresses, which are developed by dissipation of viscaneddia, near the rates of shear that are more than the shear rate throughout the liquid's bulk (Doulah, Citation1977), Second, high bubble-related stress, and falling bubbles would raise fluid temperature to more than 2000 k and increase the pressure by 26 to more than 330 atm (Hua & Thompson, Citation2000); and a chemical attack on US-generated hydroxyl radicals may be a third shear-force mechanism. The “hot-pot hypothesis” indicates the formation of free radicals (e.g., —HO., HO2. and O.) such as these intense temperature and pressure environments. Such radicals enter the chemical structure of the bacterial cell wall and degrade the cell wall to the point of degradation. Thus, the acoustic cavity results in a series of physical, mechanical and chemical processes which can disrupt bacterial and de-agglomerating clusters (Gogate, Citation2007; Joyce, Phull, Lorimer, & Mason, Citation2003). Microbial mortality is largely caused by cell dilution, localization and free radical growth (Butz & Tauscher, Citation2002). Bacterial cell forces are induced by cavitation as a result of the surface resonance. Stresses from the dissolution of gas bubbles approaching and touching the cell wall of a bacterial solution. These effects could be summarized as
Mechanical fatigue induces bacterial cell disruption over a period of time, which depends on frequency 86.
Micro-stray shear forces occur within bacterial cells (Joyce et al., Citation2002).
Chemical attack in the marine environment by free radicals (OH− and H+).
We strike the bacterial cell wall chemistry structure and degrade the cell wall until it disintegrates (Joyce et al., Citation2002; Mason et al., Citation2003).
As a consequence, the processes of disinfection depend on two primary phenomena induced by the cavitation. The first is the break-up of bacterial clusters. Firstly, the death of bacteria (or inactivation), which leads to a reduced reproductive potential for a suspension of the bacteria. The overall effect of ultrasound application is ultimately attributed to a rivalry of solution division and inactivation of bacteria.
Acoustic cavitation is the product of the sono-chemical effects of ultrasound in a fluid. Because ultrasound is a mechanical wave, it can affect acoustic cavitation and removal, including frequency, wavelength and amplitude. These settings and operating parameters that enable large bubbles, prolonged pressure oscillations, and more cavity events are therefore maximizing the disinfection rates. Bacteria die of the violent bubble collapse due to shock waves, stresses and temperature fluctuations (Chemat et al., Citation2017).
5.4.2. Disinfection by ultrasound irradiation
Some researchers have found that the% of inactivation of the microorganism increases with increasing sonication time. Nakanishi, Mukai, Kimata, Iseki, and Maeda (Citation2001) studied the inability to inactivate Cryptosporidium parvum-oocysts in drinking water and observed that about 40% of oocysts lost their kernels, respectively after 2, 10, and 20 min of sonication with 28 kHz, 97% and 99%.
Similar results for the inactivation of E. coli were found by Phull, Newman, Lorimer, Pollet, and Mason (Citation1997). They concluded that maximum inactivation was achieved using a 20 kHz probe at 15 W/cm3, after 15 min (the longest time tested). Sonication intensity is also a key factor in the inactivation of microorganisms. Escherichia coli are one the most used fecal contamination indicator in regulations and guidelines dealing with water and wastewater quality assessment.
E. coli inactivation displayed pseudo-first-order activity and frequency was observed in its duration. The effect of ultrasound intensity on E. coli at a frequency of 20 kHz in the range 270–460 W l−1. Hua and Thompson (Citation2000) studied the inactivation of coli. Our test revealed the magnitude of E. coli inactivation was approximately 2.8 Log at the highest density of 460 W l−1 after 60 min.
Cell disruption mechanism has been improved by (Li et al., Citation2016). They used TEM to obtain distinct images in order to investigate the morphological changes in E. coli cells after ultrasound treatment. According to their micrographs of untreated E. coli cells show that they retained the rod shape with normal cell walls and nuclei (). However, remarkable morphological changes were observed in E. coli cells after sonication for 20 min (). E. coli cells () showed a rough cell wall and a blurry cell membrane, with a decomposing inner structure. Some cells () were disrupted, and the cytoplasmic material was released to the extracellular medium. More strikingly, cell wall fragmentation and plasma membrane rupture were also observed ().
Figure 4. Schematic diagram of disinfection mechanisms and reaction systems during ultrasound irradiation.
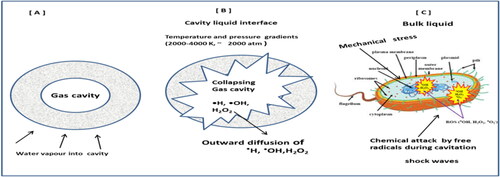
In another study, the yeast survival tested by Thacker (Citation1973) was used for ultrasonic intensities of 1, 2 and 4 W/cm2 and frequencies between 20 and 1 MHz. Survival with increasing frequency of the yeast cells decreased.
Ultrasonic wave frequency plays an essential role in the effectiveness of disinfection.
The influence of US intensity on Bacillus inactivation (Joyce et al., Citation2003). Investigators found that the key effect of high-frequency ultrasound was decline of low-deactivation bacterial agglomerates, whereas low-frequency, higher-powered kills were obtained.
In the majority of studies reviewed, the researchers used low frequency systems that allow microorganism inactivation to achieve best results related with high frequency ultrasonic techniques.
Several researchers have assessed the parameters affected by the phase of ultrasonic water disinfection (Hulsmans et al., Citation2010). While a higher flux speed, greater electrical power, and more specific energy contribute to a faster bacterial removal, a longer treatment time to achieve the same final bacterial level often needs a higher initial bacterial inoculum (Hulsmans et al., Citation2010).
5.4.3. Disinfection by ultrasound irradiation coupled to other processes
In numerous studies, the US procedure, as a pre-therapy or in conjunction with other methods of disinfection, including UV, chlorine, and ozone, examined in various conditions such as alone disinfection processes.
5.4.3.1. Ultrasound and chemicals synergistic effect
Ultrasound has also been used in combination with other advanced oxidation processes. Phull et al. (Citation1997) found a synergistic effect between sonication and chlorination. E. coli suspended in saline solution and raw stream water were used in their experiments. After 5 min of treatment time, the application of 1 mg L−1 chlorine inactivated 43% of the bacteria in the sample stream water and sonication alone inactivated 19%. When sonication was applied followed by chlorination, 86% inactivation of bacteria was achieved. After 20 min, the combined sonicity and chlorination resulted in 100% inactivation of bacteria. The findings also showed that rising bacterial kill by 40% for a 5-min treatment period in chlorine improved the sounding energy of 12 W/cm2 to 21 W/cm2.
Sonication accompanied by chlorination is safer than sonification, which induces a degassing effect and leads to lower concentrations of chlorine (Ayyildiz, Sanik, & Ileri, Citation2011).
Sequentially combined ultrasound and chlorine dioxide were used to improve Escherichia coli and the inactivation of total coliforms in raw wastewater (Nilsun & Belen, Citation2001). A simultaneous application of the ultrasonic density values of 150 or 300 W l−1 and ClO2 of 2 mg L−1 reduced the number of microorganisms by around 2.3–3.5 log reduction in the number of microorganisms, while the sum of log reductions by the individual treatments were 1.4–1.9. This enhancement was attributed to the presence of high concentration of particles in raw wastewater and their influence in improving ultrasonic cavitation effects.
In absence of equal mass concentrations of ceramic, metallic zinc, and activated carbon, Nilsun and Belen (Citation2001) investigated the efficacy of the power ultrasound for the destruction of Escherichia coli and Total coliform. They reported that ultrasound disinfection is accelerated in the order carbon > ceramic > metallic zinc with solids. Moreover, the model showed that the process rate in the presence of activated carbon was first order and the contact time required to perform 50% kill was 2.8, 2.4 nd 4 times less than in zinc-catalyzed, ceramic-catalyzed and non-catalyzed systems.
Ultimately, the synergistic interaction between sonication and H2O2 between Jyoti and Pandit (Citation2004) improved the coliform Escherichia, complete coliform and F. Bore well water output of streptococci inactivation. We concluded with a 5 mg L−1 H2O2 addition to the ultrasonic bath that the disinfection capacity of acoustic cavitation was increased ().
Figure 5. TEM photographs of E. coli cells. (a) Untreated bacteria. (b, c, and d) Bacteria treated with ultrasound for 20 min (Li et al., Citation2016).
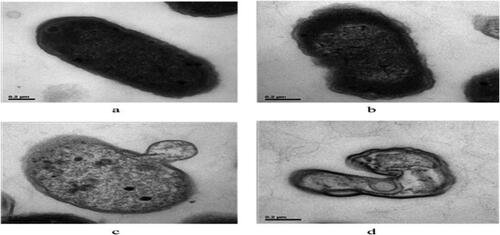
5.4.3.2. Sonication and ozonation synergistic effect
Use ultrasound with an ozone-powerful oxidant improves water and wastewater treatment through the production of emulsion from both suspended and dispersed particles, and through the prevention of ozone bubble coalescenzy, thus ensuring the total oxidation bubble area (Zou & Tang, Citation2019).
A study was conducted on the inactivation of E. coli when subjected to ozonation alone, simultaneous ozonation and sonication, and sonication followed by ozonation (Von Gunten, Citation2003). The results showed that with the same given disinfection time, ozonation alone provided the least inactivation of E. coli compared to the combination of ozonation and sonication. Ultrasonic waves indicated increased microbial inactivation with ozone, and processes of chemical oxidation caused by free ozone radicals.
The inactivation of Total coliforms, Fecal coliforms and Fecal streptococci in the bore well water also demonstrated the synergistic effect between sonication and ozonation (Jyoti & Pandit, Citation2004). They concluded that the combination of ozonation and sonication reduced to half or one quarter, depending on microorganism form the concentration of ozone needed for disinfection.
The major inconveniences in the treatment of water and sewage are the high costs for processing ozone and high energy usage for generating ultrasound. Nevertheless, both ozone and ultrasound power can be minimized in combination, thus reducing operating costs. The reduction of harmful by-products associated with ozone responses and in particular oxidation of organic and halogen materials (U.S. EPA, Citation1999) are additional advantage of the combination of ozone and therefore decreased ozone exposure (U.S. EPA, Citation1999).
5.4.3.3. Ultrasound and ultraviolet synergistic effect
Ultraviolet (UV) irradiation is a wave with a space between the ray-X and visible ray 100–400 nanometer (nm) (U.S. EPA, Citation1999). A low pressurized light (253.7 nm) or medium pressure (180–1.370 nm) for UV disinfection is used. The brightness of medium pressure lamps is much greater than that of low pressure lamps. Paleologou et al. (Citation2007) have investigated the application of ultrasound to improve the wastewater disinfection system as a pre-treatment phase combined with ultraviolet and have found that the US allowed the reaction time needed to complete removal of UV radiation from Total coliforms to be halved alone. The combined use of ultrasound and UV to enhance the disinfection of fecal coliform was investigated by Joyce, Mason, and Lorimer (Citation2006). A UV reactor with an ultrasound power density of 0.05 W/cm3 is placed within an ultrasound bath during their experiments. We found that the simultaneous use of ultrasound and UV in the UV reactor increases the overall disinfection substantially, resulting in complete inactivation in 30 minutes.
A variety of experimental studies have reported synergistic effects between sonics, UV and metal ions. Their results show that, when successive and hybrid methods are used, the efficiency of bacterial inactivation was increased and the disadvantages eliminated (Gemici, Karel, Karaer, & Koparal, Citation2018). They also demonstrated that hybrid application of water disinfection with lower environmental risk and increasing individual drawbacks of disinfection methods could effectively occur with antibacterial column, ultrasound and UV.
5.4.3.4. Ultrasound and electrolysis disinfection efficiency
Combining acoustic therapies with electrolysis is far stronger than sonication or electrolysis alone (Joyce et al., Citation2003). Joyce et al. (Citation2002) tested the efficiency of the combined process as a treatment of disinfection to inactivate Klebsiella pneumonia in ultrasound and electrolysis coupling. In an ultrasonic bath controlled at a frequency of 40 kHz, testing was carried out with electrodes immersed in the liquid phase. Carbon, copper and steel rods were the electrodes. They were made of felts and graphite. Combined therapy has been shown to be more effective than individually administered ultrasound or electrolysis. Disinfection takes place through the development of hydrogen peroxide, hydroxyl radicals, and hypo-chlorous acid, as a result of metal ions entering microbial cells. Klebsiella pneumonia was disabled 50% after 2 min, at 40 kHz and a density of 50 W l−1.
The hybrid ultrasound and electrolytic disinfection systems that include an electrolytic cell with electrodes, which is part of flow tube or open channel through which liquid or wastewater travel, were recently patentable by Schlager and Gorski (Citation2004). They are made out of iron ions, carbon, or copper. They are connected to a voltage ranging from 20 to 100 V and are powered in the range of 1 to 6 A. An ultrasound transducer is attached to the electrodes, and then increases the output of radical hydroxyl.
Disclosure statement
No potential conflict of interest was reported by the author(s).
References
- Ackah, M., Agyemang, O., & Anim, A. K. (2011). Assessment of groundwater quality for drinking and irrigation: The case study of Teiman-Oyarifa Community, Ga East Municipality, Ghana. Proceedings of the International Academy of Ecology and Environmental Sciences, 1(3–4), 186–194.
- Ambashta, R. D., & Sillanpaa, M. (2010). Water purification using magnetic assistance: A review. Journal of Hazardous Materials, 180(1–3), 38–49. doi:10.1016/j.jhazmat.2010.04.105
- Asfaram, A., Ghaedi, M., Agarwal, S., Tyagi, I., & Gupta, V. K. (2015). Removal of basic dye Auramine-O by ZnS:Cu nanoparticles loaded on activated carbon: Optimization of parameters using response surface methodology with central composite design. RSC Advances, 5(24), 18438–18450.
- Ayyildiz, O., Sanik, S., & Ileri, B. (2011). Effect of ultrasonic pretreatment on chlorine dioxide disinfection efficiency. Ultrasonics Sonochemistry, 18(2), 683–688. doi:10.1016/j.ultsonch.2010.08.008
- Bello, A. R. C., Angelis, D. F., & Domingos, R. N. (2005). Ultrasound efficiency in relation to sodium hypochlorite and filtration adsorption in microbial elimination in a water treatment plant. Brazilian Archives of Biology and Technology, 48(5), 739–745. doi:10.1590/S1516-89132005000600009
- Borea, L., Naddeo, V., Shalaby, M. S., Zarra, T., Belgiorno, V., Abdalla, H., & Shaban, A. M. (2018). Wastewater treatment by membrane ultrafiltration enhanced with ultrasound: Effect of membrane flux and ultrasonic frequency. Ultrasonics, 83, 42–47. doi:10.1016/j.ultras.2017.06.013
- Buchholz, K., Tanis, D., Macomber, S., & Farris, E. (1998). Ballast water treatment technology review. Retrieved from www.NEMW.ORGBALSURV3_US.HTM.
- Burleson, G. R., Murray, T. M., & Pollard, M. (1975). Inactivation of viruses and bacteria by ozone, with and without sonication. Applied Microbiology, 29(3), 340–344. doi:10.1128/AEM.29.3.340-344.1975
- Burzio, E., Bersanib, F., Caridia, G. C. A., Vesipaa, R., Ridolfia, L., & Manesa, C. (2020). Water disinfection by orifice-induced hydrodynamic cavitation. Ultrasonics—Sonochemistry, 60, 1–8. doi:10.1016/j.ultsonch.2019.104740
- Butz, P., & Tauscher, B. (2002). Emerging technologies: Chemical aspects. Food Research International, 35 (2–3), 279–284. doi:10.1016/S0963-9969(01)00197-1
- Capocelli, M., Prisciandaro, M., Lancia, A., & Musmarra, D. (2013). Modeling of cavitation as an advanced wastewater treatment. Desalination and Water Treatment, 51(7–9), 1609–1614. doi:10.1080/19443994.2012.705094
- Chemat, F., Rombaut, N., Sicaire, A.-G., Meullemiestre, A., Fabiano-Tixier, A.-S., & Abert-Vian, M. (2017). Ultrasound assisted extraction of food and natural products. Mechanisms, techniques, combinations, protocols and applications. A review. Ultrasonics Sonochemistry, 34, 540–560. doi:10.1016/j.ultsonch.2016.06.035
- Chen, D., Weavers, L. K., & Walker, H. W. (2006). Ultrasonic control of ceramic membrane fouling by particles: Effect of ultrasonic factors. Ultrasonics Sonochemistry, 13(5), 379–387. doi:10.1016/j.ultsonch.2005.07.004
- Cho, J. W., Amy, G., & Pellegrino, J. (1999). Membrane filtration of natural organic matter: Initial comparison of rejection and flux decline characteristics with ultrafiltration and nanofiltration membrane. Water Research, 33(11), 2517–2526. doi:10.1016/S0043-1354(98)00498-9
- Chong, M. N., Jin, B., Chow, C. W.K., & Saint, C. (2010). Recent developments in photocatalytic water treatment technology: A review. Water Research, 44(10), 2997–3027. doi:10.1016/j.watres.2010.02.039
- Chua, S. Y., AdulLatif, P., & Ibrahim, S. (2009). Effect of ultrasonic irradiation on landfill leachate. Proceedings of Postgraduate Qolloquium Semester 1.
- Crittenden, J. C., Trussell, R. R. H., & Tchobanglouse, G. (2004). Water treatment principles and design (2nd ed.). Hoboken, NJ: Wiley.
- Dahi, E. (1976). Physicochemical aspects of disinfection of water by means of ultrasound and ozone. Water Research, 10, 677–684.
- Deymier, P. A., Vasseur, J. O., Khelif, A., & Raghavan, S. (2001). Second-order sound field during megasonic cleaning of patterned silicon wafers: Application to ridges and trenches. Journal of Applied Physics, 90(8), 4211–4218. doi:10.1063/1.1398595
- Dhermendra, K., Tiwari, J., & Behari, P. S. (2008). Application of nanoparticles in waste water treatment. World Applied Sciences Journal, 3, 417–433.
- Doulah, M. S. (1977). Mechanism of disintegration of biological cells in ultrasonic cavitation. Biotechnology and Bioengineering, 19, 649–660. doi:10.1002/bit.260190504
- Drakopoulou, S., Terzakis, S., Fountoulakis, M. S., Mantzavinos, D., & Manios, T. (2009). Ultrasound-induced inactivation of gram-negative and gram-positive bacteria in secondary treated municipal wastewater. Ultrasonics Sonochemistry, 16(5), 629–634. doi:10.1016/j.ultsonch.2008.11.011
- Entezari, M. H., Petrier, C., & Devidal, P. (2003). Sonochemical degradation of phenol in water: A comparison of classical equipment with a new cylindrical reactor. Ultrasonics Sonochemistry, 10(2), 103–108. doi:10.1016/S1350-4177(02)00136-0
- EPA. (2014). Turbidity. In Water: Monitoring & Assessment. Retrieved from http://water.epa.gov/type/rsl/monitoring/vms55.cfmPerlman.
- Eskin, G. I., & Eskin, D. G. (2014). Ultrasonic treatment of light alloy melts (2nd ed.). Series: Advances in metallic alloys. Boca Raton: CRC Press.
- Flynn, H. G. (1964). Physics of acoustic cavitation in liquids. In Mason, W. P. (Ed.), Physical acoustics Part B (Vol. 1, pp. 57–172). New York, USA: Academic Press.
- Gaya, U. I., & Abdullah, A. H. (2008). Heterogeneous photocatalytic degradation of organic contaminants over titanium dioxide: A review of fundamentals, progress and problems. Journal of Photochemistry and Photobiology C: Photochemistry Reviews, 9(1), 1–12. doi:10.1016/j.jphotochemrev.2007.12.003
- Ghaedi, M., Hajjati, S., Mahmudi, Z., Tyagi, I., Agarwal, S., Maity, A., & Gupta, V. K. (2015). Modeling of competitive ultrasonic assisted removal of the dyes—Methylene blue and Safranin-O using Fe3O4 nanoparticles. Chemical Engineering Journal, 268, 28–37. doi:10.1016/j.cej.2014.12.090
- Gemici, B. T., Karel, F. B., Karaer, F., & Koparal, A. S. (2018). Water disinfection with advanced methods: Successive and hybrid application of antibacterial column with silver, ultrasound and UV radiation. Applied Ecology and Environmental Research, 16(4), 4667–4680. doi:10.15666/aeer/1604_46674680
- Gogate, P. R. (2007). Application of cavitational reactors for water disinfection: Current status and pathforward. Journal of Environmental Management, 85(4), 801–815. doi:10.1016/j.jenvman.2007.07.001
- Gogate, P. R., & Pandit, A. B. (2008). Application of cavitational reactors for cell disruption for recovery of intracellular enzymes. Journal of Chemical Technology & Biotechnology, 83(8), 1083–1093. doi:10.1002/jctb.1898
- Gopal, K., Tripathy, S. S., Bersillon, J. L., & Dubey, S. P. (2007). Chlorination byproducts, theritoxicodynamics and removal from drinking water. Journal of Hazardous Materials, 140(1–2), 1–6. doi:10.1016/j.jhazmat.2006.10.063
- Guo, Z., & Feng, R. (2008). Ultrasonic irradiation-induced degradation of low-concentration bisphenol A in aqueous solution. Journal of Hazardous Materials, 163(2–3), 855–860. doi:10.1016/j.jhazmat.07.03865
- Gupta, V. K., JainbImran, C. K., Chandr, A., & Agarwal, S. (2002). Removal of lindane and malathion from wastewater using bagasse fly ash—a sugar industry waste. Water Research, 36 (10), 2483–2490. doi:10.1016/S0043-1354(01)00474-2
- Gupta, V. K., Jain, R., Nayak, A., Agarwal, S., & Shrivastava, M. (2011). Removal of the hazardous dye—Tartrazine by photodegradation on titanium dioxide surface. Materials Science and Engineering: C, 31(5), 1062–1067. doi:10.1016/j.msec.2011.03.006
- Gupta, V. K., & Saleh, T. A. (2013). Sorption of pollutants by porous carbon, carbon nanotubes and fullerene- An overview. Environmental Science and Pollution Research, 20(5), 2828–2843. doi:10.1007/s11356-013-1524-1
- Gupta, V. K., Nayak, A., & Agarwal, S. (2015). Bioadsorbents for remediation of heavy metals: current status and their future prospects. Environmental Engineering Research, 20(1), 1–18. doi:10.4491/eer.2015.018
- Hammitt, F. G. (1980). Cavitation and multiphase flow phenomena. USA: McGraw-Hill.
- Harvey, E. N., & Loomis, L. (1928). High frequency sound waves of small intensity and their biological effects. Nature, 121(3051), 622–624. doi:10.1038/121622a0
- Hua, I., & Thompson, J. E. (2000). Inactivation of Escherichia coli by sonication at discete ultrasonic frequencies. Water Research, 34(15), 3888–3893. doi:10.1016/S0043-1354(00)00121-4
- Hulsmans, A., Joris, K., Lambert, N., Rediers, H., Declerck, P., Delaedt, Y., … Liers, S. (2010). Evaluation of process parameters of ultrasonic treatment of bacterial suspensions in a pilot scale water disinfection system. Ultrasonics Sonochemistry, 17(6), 1004–1009. doi:10.1016/j.ultsonch.2009.10.013
- Joyce, E., Mason, T. J., Phull, S. S., & Lorimer, J. P. (2002). The development and evaluation of ultrasound for the treatment of bacterial suspension. Ultrasonics Sonochemistry, 10, 315–318.
- Joyce, E., Phull, S. S., Lorimer, J. P., & Mason, T. J. (2003). The development and evaluation of ultrasound for the treatment of bacterial suspensions. A study of frequency, power and sonication time on cultured Bacillus species. Ultrasonics Sonochemistry, 10(6), 315–318. doi:10.1016/S1350-4177(03)00101-9
- Joyce, E. M., Mason, T. J., & Lorimer, J. P. (2006). Application of UV radiation or electrochemistry in conjunction with power ultrasound for the disinfection of water. International Journal of Environment and Pollution, 27(1/2/3), 222–230. doi:10.1504/IJEP.2006.010465
- Juang, R. S., & Lin, K. H. (2004). Flux recovery in the ultrafiltration of suspended solutions with ultrasound. Journal of Membrane Science, 243(1–2), 115–124. doi:10.1016/j.memsci.2004.06.013
- Jyoti, K. K., & Pandit, A. B. (2001). Water disinfection by acoustic and hydrodynamic cavitation. Biochemical Engineering Journal, 7(3), 201–212. doi:10.1016/S1369-703X(00)00128-5
- Jyoti, K. K., & Pandit, A. B. (2004). Effect of cavitation on chemical disinfection efficiency. Water Research, 38(9), 2249–2219. doi:10.1016/j.watres.2004.02.012
- Khan, E., Shen, C., & Lin, H. (2006). Use of low-frequency sonication for the production of biodegradable dissolved organic carbon in water. Environmental Engineering Science, 23(2), 367–371. doi:10.1089/ees.2006.23.367
- Khani, H., Rofouei, M. K., Arab, P., Gupta, V. K., & Vafaei, Z. (2010). Multi-walled carbon nanotubes-ionic liquid-carbon paste electrode as a super selectivity sensor: Application to potentiometric monitoring of mercury ion(II). Journal of Hazardous Materials, 183 (1–3), 402–409. doi:10.1016/j.jhazmat.2010.07.039
- Kobayashi, T., Chai, X., & Fujii, N. (1999). Ultrasound enhanced cross-flow membrane filtration. Separation and Purification Technology, 17(1), 31–40. doi:10.1016/S1383-5866(99)00023-4
- Kot-Wasik, A., Dabrowska, D., & Namiesnik, J. (2003). Degradacjazwii^zkoworganicznych w srodowisku, NoweHoryzonty i wyzwania w analityceimonitoringusrodowiskowym. Centrum DoskonaloscianalitykiiMonitoringu Srodowiskowego, Gdansk, 700–722.
- Koval, I., Shevchuk, L., & Starchevsky, V. (2011). Ultrasonic intensification of the natural water and sewage disinfection. Chemical Engineering Transactions, 24, 1315–1320.
- Kruus, P., Burk, R. C., Entezari, M. H., & Otson, R. (1997). Sonication of aqueous solutions of chlorobenzene. Ultrasonics Sonochemistry, 4(3), 229–233. doi:10.1016/S1350-4177(97)00023-0
- Kumar, R., Chauhan, A., Goyalb, M. K., Kesri, K. K., & Behari, J. (2015). Screening the effect of ultrasonic wave on effluent treatment. Journal of Bioremediation & Biodegredation, 6 (3), 1. doi:10.4172/2155-6199.1000287
- Kyllönen, H. M., Pirkonen, P., & Nyström, M. (2005). Membrane filtration enhanced by ultrasound: A review. Desalination, 181(1–3), 319–335. doi:10.1016/j.desal.2005.06.003
- Laborde, J.-L., Bouyer, C., Caltagirone, J.-P., & Gérard, A. (1998). Acoustic cavitation field prediction at low and high frequency ultrasounds. Ultrasonics, 36(1–5), 581–587. doi:10.1016/S0041-624X(97)00106-6
- Lamminen, M. O., Walker, H. W., & Weaver, L. K. (2004). Mechanisms and factors influencing the ultrasonic cleaning of particle-fouled ceramic membranes. Journal of Membrane Science, 237(1–2), 213–223. doi:10.1016/j.memsci.2004.02.031
- Lauterborn, W., & Ohl, C. D. (1997). Cavitation bubble dynamics. Ultrasonics Sonochemistry, 4(2), 65–75. 1997, doi:10.1016/S1350-4177(97)00009-6
- Leighton, T. G. (1994). The acoustic bubble. San Diego, USA: Academic Press.
- Li, J., Ahn, J., Liu, D., Chen, S., Ye, X., & Ding, T. (2016). Evaluation of ultrasound-induced damage to Escherichia coli and Staphylococcusaureus by flow cytometry and transmission electron microscopy. Applied and Environmental Microbiology, 82(6), 1828–1837. doi:10.1128/AEM.03080-15
- Li, J., Sanderson, R. D., & Jacobs, E. P. (2002). Ultrasonic cleaning of nylon microfiltration membrane fouled by Kraft paper mill effluent. Journal of Membrane Science, 205(1–2), 247–257. doi:10.1016/S0376-7388(02)00121-7
- Liang, H., Nan, J., & He, W. (2009). Algae removal by ultrasonic irradiation-coagulation. Desalination, 239, 191–197.
- Liu, H. L., & Chiou, Y. R. (2005). Optimal decolorization efficiency of Reactive Red 239 by UV/TiO2 photocatalytic process coupled with response surface methodology. Chemical Engineering Journal, 112(1–3), 173–179. doi:10.1016/j.cej.2005.07.012
- Manousaki, E., Psillakis, E., Kalogerakis, N., & Mantzavinos, D. (2004). Degradation of sodium dodecylbenzenesulfonate in water by ultrasonic irradiation. Water Research, 38(17), 3751–3759. doi:10.1016/j.watres.2004.06.002
- Manson, T., & Lorimer, J. (2002). App Sonochemistry: Uses in chemistry and processing. Weinheim: Wiley-VCH.
- Mason, T. J., Cobley, A. J., Graves, J. E., & Morgan, D. (2011). New evidence for the inverse dependence of mechanical and chemical effects on the frequency of ultrasound. Ultrasonics Sonochemistry, 18(1), 226–230. doi:10.1016/j.ultsonch.2010.05.008
- Mason, T. J., Joyce, E., Phull, S. S., & Lorimer, J. P. (2003). Potential uses of ultrasound in the biological decontamination of water. Ultrasonics Sonochemistry, 10(6), 319–323. doi:10.1016/S1350-4177(03)00102-0
- Matsumoto, Y., Miwa, T., Nakao, S. I., & Kimura, S. (1996). Improvement of membrane permeation performance by ultrasonic microfiltration. Journal of Chemical Engineering of Japan, 29(4), 561–567. doi:10.1252/jcej.29.561
- Mittal, A., Mittal, J., Malviya, A., & Gupta, V. K. (2010). Removal and recovery of Chrysoidine Y from aqueous solutions by waste materials. Journal of Colloid and Interface Science, 344(2), 497–507. doi:10.1016/j.jcis.2010.01.007
- Muthukumaran, S., Yang, K., Seuren, A., Kentish, S., Ashokkumar, M., Steven, G. W., & Grieser, F. (2004). The use of ultrasonic cleaning for ultrafiltration membranes in the dairy industry. Separation and Purification Technology, 39(1–2), 99–107. doi:10.1016/j.seppur.2003.12.013
- Mutiarani, M., & Trisnobudi, A. (2009). Ultrasonic irradiation in decreasing water turbidity.
- Naffrechoux, E., Chanoux, S., Petrier, C., & Suptil, J. (2000). Sonochemical and photochemical oxidation of organic matter. Ultrasonics Sonochemistry, 7(4), 255–259. doi:10.1016/S1350-4177(00)00054-7
- Nakanishi, M., Mukai, S., Kimata, I., Iseki, M., & Maeda, Y. (2001). Inactivation of Cryptosporidium parvumOocysts in drinking water by high-intensity ultrasonic waves. In Proceedings of the American Water Works Association, 2001 Annual Conference (pp. 17–21). Washington DC.
- Nakatsuka, S., Nakate, I., & Miyano, T. (1996). Drinking water treatment by using ultrafiltration hollow fiber membranes. Desalination, 106(1–3), 55–61. doi:10.1016/S0011-9164(96)00092-6
- Naseri, S., Vaezi, F., Mahvi, A. H., Nabizadeh, R., & Haddadi, S. (2006). Determination of the ultrasonic effectiveness in advanced wastewater treatment. Iranian Journal of Environmental Health Science & Engineering, 3, 109–116.
- Ng, K. K., Lin, C. F., Lateef, S. K., Panchangam, S. C., Hong, P. K. A., & Yang, P. Y. (2010). The effect of soluble microbial products in a fixed carrier biological system. Separation and Purification Technology, 72(1), 98–104.
- Nikolopoulos, A. N., Igglessi-Markopoulou, O., & Papayannakos, N. (2006). Ultrasound assisted catalytic wet peroxide oxidation of phenol: Kinetics and intraparticle diffusion effects. Ultrasonics Sonochemistry, 13(1), 92–97. doi:10.1016/j.ultsonch.2004.10.001
- Nilsun, H. I., & Belen, R. (2001). Aqueous phase disinfection with power ultrasound. Process Kinetics and Effect of Solid Catalysts Environmental Science & Technology, 35 (9), 1885–1888. doi:10.1021/es000157
- Noltingk, B. E., & Neppiras, E. A. (1950). Cavitation produced by ultrasonics. Proceedings of the Physical Society. Section B, 63(9), 674–685. doi:10.1088/0370-1301/63/9/305
- Oturan, M. A., Sirés, I., Oturan, N., Pérocheau, S., Laborde, J. L., & Trévin, S. (2008). Sonoelectro- Fenton process: A novel hybrid techniquefor the destruction of organic pollutants in water, Journal of Electroanalytical Chemistry, 624(1–2), 329–332.
- Ozonek, J. (2012). Application of hydrodynamic cavitation in environmental engineering. London: Taylor & Francis Group.
- Paleologou, A., Marakas, H., Xekoukoulotakis, N. P., Moya, A., Vergara, Y., Kalogerakis, N., … Mantzavinosa, D. (2007). Disinfection of water and wastewater by TiO2 photocatalysis, sonolysis and UV-C irradiation. Catalysis Today, 129(1–2), 136–142. doi:10.1016/j.cattod.2007.06.059
- Petrier, C., Jeunet, A., Luche, J. L., & Reverdy, G. (1992). Unexpected frequency effects on the rate of oxidative processes induced by ultrasound. Journal of the American Chemical Society, 114(8), 3148–3150. doi:10.1021/ja00034a077
- Petrier, C., Jiang, Y., & Lamy, M. F. (1998). Ultrasound and environment: Sonochemical destruction of chloroaromatic derivatives. Environmental Science & Technology, 32(9), 1316–1318. doi:10.1021/es970662x
- Phull, S. S., Newman, A. P., Lorimer, J. P., Pollet, B., & Mason, T. J. (1997). The development and evaluation of ultrasound in the biocidal treatment of water. Ultrasonics Sonochemistry, 4(2), 157–164. doi:10.1016/S1350-4177(97)00029-1
- Pilli, S., Bhunia, P., Yan, S., LeBlanc, R. J., Tyagi, R. D., & Surampalli, R. Y. (2011). Ultrasonic pretreatment of sludge: A review. Ultrasonics Sonochemistry, 18(1), 1–18. doi:10.1016/j.ultsonch.2010.02.014
- Pozos, N., Scow, K., Wuertz, S., (2004). UV disinfection in a model distribution system: Biofilm growth and microbial community. Water Resources, 38, 3083–3091 10.
- Psillakis, E., Goula, G., Kalogerakis, N., & Mantzavinos, D. (2004). Degradation of polycyclicaromatic hydrocarbons in aqueous solutions by ultrasonic irradiation. Journal of Hazardous Materials, 108, 95102.
- Rajendran, S., Khan, M. M., Gracia, F., Qin, J., Gupta, V. K., & Arumainathan, S. (2016). Ce3+-ion-induced visible-light photocatalytic degradation and electrochemical activity of ZnO/CeO2 nanocomposite. Scientific Reports, 6(1), 31641. doi:10.1038/srep31641
- Saleh, T. A., & Gupta, V. K. (2011). Functionalization of tungsten oxide into MWCNT and its application for sunlight-induced degradation of rhodamine B. Journal of Colloid and Interface Science, 362 (2), 337–344. doi:10.1016/j.jcis.2011.06.081
- Saleh, T. A., & Gupta, V. K. (2012a). Photo-catalyzed degradation of hazardous dye methyl orange by use of a composite catalyst consisting of multi-walled carbon nanotubes and titanium dioxid. Journal of Colloid and Interface Science, 371(1), 101–106. doi:10.1016/j.jcis.2011.12.038
- Saleh, T. A., & Gupta, V. K. (2014). Processing methods, characteristics and adsorption behavior of tire derived carbons: A review. Advances in Colloid and Interface Science, 211, 93–101. doi:10.1016/j.cis.2014.06.006
- Saravanan, R., Karthikeyan, S., Gupta, V. K., Sekaran, G., Narayanan, V., & Stephen, A. (2013). photocatalytic activity of ZnO/CuO nanocomposite for the degradation of textile dye on visible light illumination. Materials Science and Engineering: C, 33(1), 91–98. doi:10.1016/j.msec.2012.08.011
- Saravanan, R., Gupta, V. K., Mosquera, E., & Gracia, F. (2014). Preparation and characterization of V2O5/ZnO nanocomposite system for photocatalytic application. Journal of Molecular Liquids, 198, 409–412. doi:10.1016/j.molliq.2014.07.030
- Saravanan, R., Mansoob Khan, M., Gupta, V. K., Mosquera, E., Gracia, F., Narayanan, V., & Stephen, A. (2015a). ZnO/Ag/CdO nanocomposite for visible light-induced photocatalytic degradation of industrial textile effluents. Journal of Colloid and Interface Science, 452, 126–133. doi:10.1016/j.jcis.2015.04.035
- Saravanan, R., Khan, M. M., Gupta, V. K., Mosquera, E., Gracia, F., Narayanan, V., & Stephen, A. (2015b). ZnO/Ag/Mn2O3 nanocomposite for visible light-induced industrial textile effluent degradation, uric acid and ascorbic acid sensing and antimicrobial activity. RSC Advances, 5(44), 34645–34651. doi:10.1039/C5RA02557E
- Saravanan, R., Sacari, E., Gracia, F., Khan, M. M., Mosquera, E., & Gupta, V. K. (2016). Conducting PANI stimulated ZnO system for visible light photocatalytic degradation of coloured dyes. Journal of Molecular Liquids, 221, 1029–1033. doi:10.1016/j.molliq.2016.06.074
- Schlager, K. J., & Gorski, S. H. (2004). Electronic water disinfection apparatus.US Patents. N° 6780306.
- Simon, A., Gondrexon, N., Taha, S., Cabon, J., & Dorange, G. (2000). Low frequency ultrasound to improve dead-end ultrafiltration performance. Separation Science and Technology, 35(16), 2619–2637. doi:10.1081/SS-100102359
- Stefan, A., & Balan, G. (2011). The chemistry of the raw water treated by air-jet ultrasound generator. Rev. Roum. Sci. Tech. Mec. Appl, 56(1), 85–92.
- Suslick, K. S. (1994). The chemistry of ultrasound (pp. 138–155). Chicago: Encyclopedia Britannica.
- Suslick, K. S., Hammerton, D. A., & Cline, R. E. J. (1986). The sonochemical hot-spot. Journal of the American Chemical Society, 108(18), 5641–5642. doi:10.1021/ja00278a055
- Tansel, B. (2008). New technologies for water and wastewater treatment: A survey of recent patents. Recent Patents on Chemical Engineering, 1(1), 17–26. doi:10.2174/2211334710801010017
- Thacker, J. (1973). An approach to the mechanism of killing of cells in suspension by ultrasound. Biochimica et Biophysica Acta (BBA)—General Subjects, 304(2), 240–248. doi:10.1016/0304-4165(73)90241-9
- Thornycrof, J., & Sidney, B. (1895). Torpedo boat destroyers. ProcInst Civil Engineers, 122, 51.
- Tiwari, R. N. (2011). Assessment of groundwater quality and pollution potential of Jawa Block Rewa District, Madhya Pradesh, India. Proceedings of the International Academy of Ecology and Environmental Sciences, 1(3–4), 202–212.
- Torres, R. A., Pétrier, C., Combet, E., Carrier, M., & Pulgarin, C. (2008). Ultrasonic cavitation applied to the treatment of bisphenol A. Ultrasonics Sonochemistry, 15(4), 605–611. doi:10.1016/j.ultsonch.2007.07.003
- Tzanakis, I., Lebon, G. S. B., Eskin, D. G., & Pericleous, K. A. (2017). Characterizing the cavitation development and acoustic spectrum in various liquids. Ultrasonics Sonochemistry, 34, 651–662. doi:10.1016/j.ultsonch.2016.06.034
- U.S. Environmental Protection Agency (U.S. EPA). (1999). Office of Water. UV Irradiation. Alternative disinfectants and oxidants guidance manual; EPA 815-R-99-014.
- Von Gunten, U. (2003). Ozonation of drinking water: Part II. Disinfection and by-product formation in presence of bromide, iodide and chlorine. Water Research, 37(7), 1469–1487. doi:10.1016/S0043-1354(02)00458-X
- Warade, A. R., Gaikwad, R. W., Sapkal, R. S., & Sapkal, V. S. (2016). Review on wastewater treatment by hydrodynamic cavitation. IOSR Journal of Environmental Science, Toxicology and Food Technology (IOSR-JESTFT), 10 (12), 67–72.
- Winward, G. P., Avery, L. M., Stephenson, T., & Jefferson, B. (2008). Chlorine disinfection of grey water for reuse: Effect of organics and particles. Water Research, 42(1–2), 483–491. doi:10.1016/j.watres.2007.07.042
- Wong, K. Y. K. (2002). Ultrasound as a sole or synergistic disinfectant in drinking water (Master Thesis). Worcester Polytechnic Institute, USA.
- Yuk, Y. J., & Youm, K. H. (2003). Enhancement of ultrafiltration performance using ultrasound. Memburein, 13, 283–290.
- Zhang, G., Zhang, P., & Fan, M. (2009). Ultrasound-enhanced coagulation for Microcystisaeruginosaremoval. Ultrasonics Sonochemistry, 16(3), 334–338. doi:10.1016/j.ultsonch.2008.10.014
- Zhang, W. J., Jiang, F. B., & Ou, J. F. (2011). Global pesticide consumption and pollution: With China as a focus. Proceedings of the International Academy of Ecology and Environmental Sciences, 1(2), 125–144.
- Zhang, F., Wang, X., Liu, H., Liu, C., Wan, Y., Long, Y., & Cai, Z. (2019). Recent advances and applications of semiconductor photocatalytic technology. Applied Sciences, 9(12), 2489. doi:10.3390/app9122489
- Zhou, Q., Lau, S., Wu, D., & Shung, K. K. (2011). Piezoelectric films for high frequency ultrasonic transducers in biomedical applications. Progress in Materials Science, 56(2), 139–174. doi:10.1016/j.pmatsci.2010.09.001
- Zou, H., & Tang, H. (2019). Comparison of different bacteria inactivation by a novel continuous-flow ultrasound/chlorination water treatment system in a pilot scale. Water, 11(2), 258. doi:10.3390/w11020258