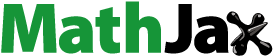
ABSTRACT
Manganese oxide-loaded sludge-derived biochar (Mn-SDBC) was synthesized and used to activate persulfate (PS) for Orange G (OG) degradation. X-ray fluorescence and Fourier transform infrared spectroscopy analysis indicated that the manganese oxides were successfully loaded on the SDBC. The result of batch experiments suggests that OG can be efficiently degraded by PS activated by Mn-SDBC. Metal loading rate, biochar dosage and PS concentration play a crucial role in experiments, whereas pH has a minor effect on OG removal. The free radical quenching experiment was investigated to determine the predominant radical species, and the activation mechanism was also illustrated. The as-synthesized Mn-SDBC can efficiently activate PS and removal OG under a continuous flow condition in a fixed-bed column. Our study suggests that Mn-SDBC would be a promising catalyst for the remediation of dye-contaminated wastewater.
1. Introduction
Advanced oxidation processes that are based on the generation of active radicals such as hydroxyl radicals and sulfate radicals derived from oxidant directly or indirectly have been given growing attention to the degradation of refractory organic pollutants in soil and water [Citation1,Citation2]. Compared to hydroxyl radicals, sulfate radicals have received more attention in recent years since the sulfate radicals have higher redox potential (, 2.5–3.1 V) than that of hydroxyl radicals (
, 1.9–2.7 V), higher stability that facilitates storage and transportation, wide reactivity with contaminants, relatively long lifetime of generated
and relatively lower cost demand, which benefit its efficient and sustainable degradation of contaminants [Citation3,Citation4]. Sulfate radicals can be generated via activation of persulfate (PS) or peroxymonosulfate (PMS) along with other radicals such as hydroxyl radical by a series of physiochemical processes such as thermal, UV light, transition metals, base and other means. Finding a better means to activate PS for oxidative species generation and manipulate the oxidative properties for effective degradation of pollutant has been recognized as the main challenge.
Among these initiators, transition metal catalysts have been recognized as one of the most effective activators in PS activation due to its lower consumption of both energy and chemicals. Metallic iron (Fe)-based materials that can provide Fe2+ are most wildly utilized transitional metal in the environmental application. Nevertheless, drawbacks such as excessive Fe2+ will react with as-formed in a short time, which would significantly lower contaminant degradation efficiency. In addition, the low-valence Fe is easily deactivated; thus the catalytic iron oxides alone is not sufficient to efficiently activate PS [Citation5]. Alternately, theheterogeneous catalyst was recognized as more effective catalyst compared to homogeneous catalyst since in most cases, oxidative radicals are generated on the surface of catalysts [Citation6].
Sewage sludge, a semi-solid residual material that contains various metals, is an inevitable by-product generated during municipal or industrial wastewater treatment. Disposal of huge quantity of sludge in an economically and environmentally tolerable manner has become a major challenge in many countries. Among various treatment methods, pyrolysis has been considered as an excellent alternative to achieve reduction of sewage volume as well as convert sludge into value-added functional material (biochar) with abundant active sites for multiple applications such as pollutant mitigation and soil amendment [Citation7,Citation8]. The sludge-derived biochar (SDBC) is a carbon-mineral adsorbent with abundant mineral oxides such as iron oxides, which could be a potential effective material for PS activation. A few previous works that investigate the efficiency of PS activation by SDBC have demonstrated that the activation mechanism of PS by SDBC was mainly attributed to metals such as iron oxides and presence of oxygen-containing functional groups such as semiquinone [Citation4,Citation9,Citation10]. However, the relatively poor performance of SDBC and pursuit of more efficient catalysts promote further researches to improve the catalytic activity of SDBC by facile means.
Manganese oxides are wildly present in the natural environment and have the merit of lower toxicity compared with some other transition metals such as cobalt and copper [Citation11]. However, studies on exploring manganese-based catalysts are quite rare compared to iron, cobalt and copper. With different valences, manganese oxides and its composites can be effective catalysts that can be applied in environmental remediation activation of PS. It has been reported that bimetallic catalysts could efficaciously boost the performance of catalysts from various perspectives [Citation12]. In municipal sludge and SDBC, iron occupies a large proportion of the total metals, while the amount of manganese is relatively rare [Citation13]. By incorporating manganese oxides in SDBC to fabricate heterogeneous catalysts, the modified biochar could have a synergic effect that shows a more desirable catalytic efficiency. Moreover, previous works mainly focus on a batch experiment to investigate PS activation mechanism as well as contaminant degradation pathway, with little effort devoted to demonstrate the catalyst long-term performance, which is essential information for its field application.
Consequently, in this work, a novel heterogeneous catalyst was synthesized by loading manganese oxides into SDBC and its performance of PS activation for Orange G (OG) degradation in both batch and column study was investigated. OG, a typical and poisonous azo dye which widely existed in textile wastewater, was chosen as our target model pollutant [Citation13]. The effect of several experiment parameters on the degradation efficiency was investigated. The physiochemical property of the synthesized biochar was determined, and the PS activation mechanism was illustrated. Additionally, a column packed with modified biochar was investigated to determine its long-term activation efficiency.
2. Materials and methods
2.1. Materials
Sewage sludge collected from the secondary sedimentation tank of a local municipal wastewater treatment plant in Wuhan, China, was chosen as the feedstock biomass for SDBC production. Manganese(II) chloride tetrahydrate, sodium PS, methanol, tert-butanol (TBA), OG, sodium nitrate, sodium hydroxide and nitric acid were purchased from Sinopharm Chemical Reagent Co., Ltd. (Shanghai, China).
2.2. Biochar production
The collected sludge was oven dried for 48 h and grounded to pass through a 0.8 mm sieve. The SDBC was produced through slow pyrolysis of the sludge granule at 600°C for 2 h in a muffle furnace under N2 atmosphere. The obtained SDBC was washed with deionized (DI) water for several minutes to remove ash and impurities and dried at 60°C overnight. The manganese oxide-loaded SDBC was prepared by immersing 20 g SDBC in 1L MnCl2 solution ranging from 0 to 60mmol/L and agitated for 2 h using a magnetic stirrer. The suspension was oven dried, pyrolyzed at 600°C for an additional 30 min. The obtained modified biochar was washed with DI water thoroughly, oven dried at 60°C and stored in an airtight container till further use.
2.3. Biochar characterization
The metal content was determined by X-ray fluorescence spectroscopy (XRF, S4 PIONEER, Bruker AXS GmbH, Karlsruhe, Germany). The functional groups in the surface of the biochar were identified using a Fourier Transform Infrared spectroscopy (FTIR, Nicolet 6700, Thermo Electron Scientific Inc., Waltham, USA), by measuring the absorbance from 400 to 4000 cm−1 with a combined 40 scans.
2.4. Batch experiments and column study
The batch experiments were carried out in 150 ml flasks in a water bath vibrator that shakes at 120 rpm and 20°C. Since the dye wastewater is typically characterized by high levels of COD and color, a high concentration of OG was chosen in this study [Citation14,Citation15]. The effect of metal loading rate, biochar dosage, PS concentration as well as pH was investigated. For batch experiments, 50 mL OG solution with a concentration of 1500 mg/L was added first. Then, biochar ranging from 0.02 to 0.11 g was added into the flask. Finally, a 5 mL PS solution with a desired concentration ranging from 20 to 80 mM was added. In some experiments, initial pH (3–9) was adjusted with 0.1 M HNO3 and 0.1 M NaOH. Methanol (MeOH) and TBA were adopted as radical scavengers in this experiment. OG was analyzed using UV/Visible spectrophotometer (UV-1100, Mapada Instruments Co., Ltd, Shanghai, China) at 478 nm. Degradation kinetics was calculated followed pseudo-first-order kinetic model:
where and
are the concentrations of OG at time 0 and t, respectively. The pseudo-first-order rate constant (
) can be obtained by linear regression of ln (
/
) versus degradation time.
The column study was conducted on a plastic column with an internal diameter of 1 cm and a height of 5 cm. The manganese oxide-modified SDBC was packed into the column between two permeable layers. An amount of 0.5 g biochar was added into the column with a bed height of 1 cm. The OG and PS concentration for column study is 1500 mg/L and 3 mmol/L, respectively. The up-flow rate was set at 1.2 mL/min using a precise peristaltic pump (BT1004-1, Leadfluid CO., Ltd, Baoding, China). The effluent was sampled at a fixed time interval and analyzed for OG concentration.
3. Results and discussion
3.1. Effect of metal loading rate
shows the effect of different metal loading rate on OG degradation experiments. A slow degradation with a 34% removal rate in 720 min was observed by pristine SDBC without metal loading, which can be attributed to the abundant metal content such as iron that can potentially activate PS in the SDBC [Citation4]. The OG removal efficiency increased from 34.15% to 90.28% as the Mn oxide loading rate increased from 0 to 40 mM. This strongly positive relationship between metal loading rate and degradation efficiency demonstrates that the loaded manganese oxides could significantly strengthen the PS activation and thus result in a higher and quicker degrade rate. However, further increasing the loading rate of manganese oxides from 40 to 60 mM did not efficiently promote OG degradation. Although a slightly higher removal rate was achieved with 60 mM loading rate in 720 min than 40 mM loading rate, its degradation rate in the first few hours was actually slower than 40 mM loading rate. With a higher loading rate, a higher content of oxides occupied and aggregated on the surface of SDBC and potentially decreased its surface area [Citation16]. And a smaller available surface area would inevitably decrease its OG degradation rate. In addition, a slower degradation rate could also result from species self-scavenging and PS consumed owing to the relative high concentration of
in the solution [Citation17]. A slight higher degradation removal rate in the next few hours may stems from the fact that more catalysis component became available in the slow degradation stage because of higher manganese content. The loading rate of 40 mM was identified as the most suitable for SDBC modification to activate PS for OG degradation in our experiments. The effect of adsorption was also examined by the same experiments with PS omitted. Less than 3% of OG was removed from the solution by the same biochar in 720 min. Because the SDBC is enriched with metal content and little carbon content, its affinity of SDBC towards OG was weak. With a high concentration of OG as 1500 mg/L, the contribution of sorption in this degradation experiments became negligible. This result clearly indicates that PS activation was the main contributor of OG degradation in our experiments.
3.2. Effect of pH
The solubility and stability of metals oxides and the oxidation effect of oxidation processes could be greatly influenced by the changes of pH. However, as shown in , the removal efficiency only decreased a little from 95.32% to 88.42% when pH increased from 3 to 9. This result indicates that although an acidic condition does facilitate the oxidation processes, such influence is not significant. The final pH was measured and presented in . It is notable that the final pH for all the set is around 2.8, which suggests the consumption of OH− and generation of H+ during the oxidation of OH−/H2O into by
. The reaction could be expressed as Equations (2) and (3). This unrelated final pH to initial pH could help explain the similar removal efficiency in different initial pH setup. Compared to Fenton-like reactions that have a strict demand for pH condition, PS activated by modified SDBC is able to work in a wide range of pH with high removal efficiency. In addition, in another test, the final pH for initial pH 3 was 3.12 when the PS dosage was cut in half (data not shown in the figure), which can attribute to the buffering capacity of modified SDBC which is rich in metal oxides.
3.3. Effect of biochar dosage
shows that the degradation efficiency of OG is apparently contingent on the catalyst dosage. The OG removal rate increases with an increasing biochar dosage. At 0.5 g/L, 75.28% removal rate can be achieved in 720 min. When catalyst dosage was amount to 1.0, 1.5 and 2.0 g/L, the OG removal efficiency increased notably to 90.28%, 94.22% and 95.94%, respectively. This result indicates that with additional dosage, more active sites become available for PS activation, which generates more radicals and results in a higher OG removal efficiency. When the dosage is low (e.g., 0.5 g/L), the almost constant rate of OG degradation was observed in the first 360 min. Since the PS dosage for all the set was the same, lower removal efficiency and slower rate of degradation between 360 and 720 min suggest a reduced amount of active site in biochar. As mentioned in Section 3.2, the final pH decreased to around 2.8. In this acidic condition, manganese oxides and other metal oxides that could serve as activation sites are more readily dissolute into the solution, and thus the degradation rate slowed down regardless of the excessive PS in solution, which leads to a lower removal efficiency in 720 min compared to higher dosage [Citation18].
3.4. Effect of PS concentration
Since PS was the source of high oxidative radicals in the degradation processes, the effect of PS concentration in the solution was investigated at different PS concentrations from 1 mM to 7mM. As depicted in , an increase in the level of PS concentration consequently enhanced the OG removal efficiency, which was promoted from 55.82% to 97.91%, with PS concentration increasing from 1 mM to 5 mM. As more PS was added into the solution, a quicker reaction and more complete degradation was observed, which indicates that more active species were produced and higher catalytic efficiency is resulted. However, a further increase in PS concentration has a minor effect on the OG degradation from 5 mM to 7 mM, which mainly results from saturation of all activation sites by excessive PS. Moreover, several works reported that an excessive amount of PS may generate a negative effect on the degradation efficiency, since excess PS would consume the reactive oxidation species to form other less oxidative compounds [Citation19]. Considering the cost and degradation efficiency, the PS concentration was set as 3 mM for later experiments.
3.5. Characterization
In order to determine the compound formula and its relative concentration, XRF analysis was carried out, and the results are listed in . The SDBC has abundant inorganic component, and Fe (4.3%) takes up a considerable proportion of all the metals while a small proportion of Mn (0.193%) was detected. After loading manganese oxides into the SDBC, Mn-SDBC shows a notable component of Mn (5.912%) compared to original SDBC, which suggests that the manganese oxides were successfully loaded on SDBC. The sample of post-reaction Mn-SDBC was also examined. The manganese oxides were found significantly reduced compared to fresh Mn-SDBC (0.717% vs. 5.912%). However, Fe component slightly increased after reaction, which may own to the loss of other easily dissolved components. In fact, Fe minerals are more stable than Mn minerals in an acidic environment and are less likely dissolved into the solution [Citation20].
Table 1. Compound formula and concentration of SDBC, Mn-SDBC and Mn-SDBC after the reaction.
FTIR measurements were carried out to probe into the surface layer composition and examine the functional groups in the biochar sample. As shown in , two peaks around 1504 and 1350 cm−1 that are assigned to C = O stretching vibration of -COOH group and single- and double-bond species of -OH groups appeared in both SDBC and Mn-SDBC, respectively [Citation21]. Previous work reported that, by releasing proton, functional groups such as C-OH and -COOH transformed into and
and activated PS to generate sulfate free radicals [Citation22]. The peak disappeared after the reaction, which indicates those functional groups also contributed to the PS activation reaction. Two peaks around 628–580 cm−1 that can be assigned to Mn-O appeared in Mn-SDBC, while such peaks were not found in SDBC and Mn-SDBC after reaction, which indicates that the manganese oxides were successfully loaded in the surface of SDBC and these oxides were dissolved into the solution during the experiment and cause Mn loss [Citation18,Citation21]. This result is well-correlated with the results of XRF.
3.6. Activation mechanism
To identify the oxidative radical species in the PS activation process, scavenger studies were conducted. The relative contributions of sulfate and hydroxyl radicals were distinguished through the chemical quenching studies by using excess MeOH and TBA as the radical scavengers. Methanol acts as scavenger of both and
at rate constants of
and
, whereas TBA is often used as an
scavenger since the rate constant of TBA with
is approximately 1000 times that of TBA with
[Citation23]. As shown in , both MeOH and TBA would inhibit the Kobs of OG degradation. The Kobs obviously decreased much faster with an increase in the MeOH/PS molar ratio compared to an increasing ratio of TBA/PS. These results suggested that
and OH were both predominant radical species in the present work. As mentioned in Section 3.2,
could react with OH− and H2O and generate
. The result of scavenger study is well-correlated with the result of the effect of pH.
Figure 6. Effect of scavengers. Metal loading rate = 40 mM, initial pH = 6, biochar dosage = 1 g/L, PS concentration = 3mM.
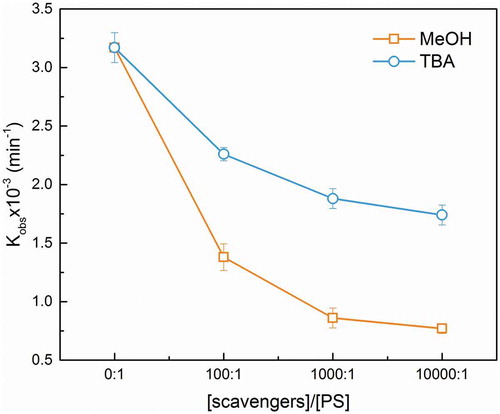
Sulfate radicals are generated via the PS activation by electron transfer between redox pairs on the surface of Mn-SDBC, where Mn and Fe are likely to play a crucial role. The result of metal loading rate clearly indicates that the loaded manganese oxide was the main contributor of the PS activation. With a 40 mM loading rate, the capacity of Mn-loaded SDBC to activate PS for OG degradation is three times higher than pristine SDBC. In addition to loaded manganese component, other mineral contents such as iron could also activate PS for OG degradation, as previous work suggested [Citation4,Citation10]. The potential activation mechanism can be expressed as below [Citation24]:
As mentioned in XRF analysis, the Mn component was significantly reduced after reaction, whereas the Fe components largely remain existed in the SDBC. The lower degradation efficiency in the latter stage of reaction suggests a declined PS activation efficiency, which could be attributed to the loss of Mn component and depletion of Fe2+ species that was transformed into Fe3+ [Citation4,Citation25]. Another work concludes that the transformation between Fe2+ to Fe3+ and Fe3+ to Fe2+ could slowly activate PS (Equation (5)) and would show a slow pollutant degradation stage after the quick degradation stage [Citation26]. Moreover, as suggested in FTIR analysis, functional groups may also contribute to the PS activation reaction. The mechanism can be expressed as below [Citation24]:
3.7. Column study
To investigate the feasibility of Mn-SDBC activate PS in a continuous flow condition, the dynamic of OG removal in a fixed-bed column was studied. As depicted in , with a dosage of 0.5 g Mn-SDBC, the column achieves a remarkable OG degradation efficiency. In the first 0.5 h, the OG concentration of effluent is 0, which can be attributed to the complete degradation of OG and interception by Mn-SDBC bed. After 0.5 h, the concentration of OG in effluent gradually increased as a function of time and the degradation efficiency becomes less than 20% after 80 h, which can be attributed to the loss of active site because of Mn loss as well as depletion of Fe2+ species. The latter stage is prolonged with minor degradation efficiency. About 10% degradation effect was maintained in 113 h, further demonstrating the activation of PS by Fe3+ as mentioned in Section 3.6. Interestingly, before the column was shut down in 113 h, the flow rate was adjusted to 0.4 mL/min and the degradation efficiency of effluent greatly enhanced to about 60% compared to 10% before adjusting. This result further confirmed the loss of reactive site during the PS activation process, where more time is required for effectively PS activation and OG degradation. It also suggests that if the flow rate was carefully controlled according to the OG concentration in the effluent, a desirable removal rate can be achieved. It is conceivable that with higher bed height as well as adjusting the flow rate according to the OG concentration in the effluent, the fixed column pack with Mn-SDBC could treat actual wastewater efficiently.
4. Conclusion
In this study, the Mn-SDBC was successfully synthesized and characterized. The factors that controlled the degradation of OG by Mn-SDBC activating PS were explored. The pH has a minor effect on the degradation efficiency, whereas the biochar dosage as well as PS concentration could greatly influence the efficiency. The result of XRF reveals that the Mn component was successfully loaded in the Mn-SDBC, and the Mn component significantly reduced after PS activation reaction, whereas Fe component still remained in the SDBC. The result of FTIR suggests that the functional groups also contributed to the PS activation. The free radical quenching experiment suggests the predominant free radical species were and
. The fixed-bed column packed with Mn-SDBC could activate and degrade OG efficiently. This study showed that the Mn-SDBC beads have great potential as efficient catalyst for the treatment of wastewater polluted with OG in a continuous operation mode.
Disclosure statement
No potential conflict of interest was reported by the authors.
Additional information
Funding
References
- Oturan MA, Aaron J-J. Advanced oxidation processes in water/wastewater treatment: principles and applications. A review. Crit Rev Environ Sci Technol. 2014;44:2577–2641.
- Wang JL, Xu LJ. Advanced oxidation processes for wastewater treatment: formation of hydroxyl radical and application. Crit Rev Environ Sci Technol. 2012;42:251–325.
- Fang G, Gao J, Dionysiou DD, et al. Activation of persulfate by quinones: free radical reactions and implication for the degradation of PCBs. Environ Sci Technol. 2013;47:4605–4611.
- Wang J, Liao Z, Ifthikar J, et al. Treatment of refractory contaminants by sludge-derived biochar/persulfate system via both adsorption and advanced oxidation process. Chemosphere. 2017;185:754–763.
- Liu Y, Zhou A, Gan Y, et al. Roles of hydroxyl and sulfate radicals in degradation of trichloroethene by persulfate activated with Fe2+ and zero-valent iron: insights from carbon isotope fractionation. J Hazard Mater. 2018;344:98–103.
- Lee YC, Lo SL, Kuo J, et al. Promoted degradation of perfluorooctanic acid by persulfate when adding activated carbon. J Hazard Mater. 2013;261:463–469.
- Li M, Tang Y, Lu XY, et al. Phosphorus speciation in sewage sludge and the sludge-derived biochar by a combination of experimental methods and theoretical simulation. Water Res. 2018;140:90–99.
- Feng H, Zheng M, Dong H, et al. Three-dimensional honeycomb-like hierarchically structured carbon for high-performance supercapacitors derived from high-ash-content sewage sludge. J Mater Chem A. 2015;3:15225–15234.
- Wang S, Wang J. Activation of peroxymonosulfate by sludge-derived biochar for the degradation of triclosan in water and wastewater. Chem Eng J. 2019;356:350–358.
- Wang X, Gu L, Zhou P, et al. Pyrolytic temperature dependent conversion of sewage sludge to carbon catalyst and their performance in persulfate degradation of 2-Naphthol. Chem Eng J. 2017;324:203–215.
- Wang Y, Sun H, Ang HM, et al. 3D-hierarchically structured MnO2 for catalytic oxidation of phenol solutions by activation of peroxymonosulfate: structure dependence and mechanism. Appl Catal B. 2015;164:159–167.
- Dong Z, Zhang Q, Chen B-Y, et al. Oxidation of bisphenol A by persulfate via Fe3O4-α-MnO2 nanoflower-like catalyst: mechanism and efficiency. Chem Eng J. 2019;357:337–347.
- Chen T, Zhang Y, Wang H, et al. Influence of pyrolysis temperature on characteristics and heavy metal adsorptive performance of biochar derived from municipal sewage sludge. Bioresour Technol. 2014;164:47–54.
- Yu C, Li G, Wei L, et al. Fabrication, characterization of β-MnO2 microrod catalysts and their performance in rapid degradation of dyes of high concentration. Catal Today. 2014;224:154–162.
- Xiong Y, Strunk PJ, Xia H, et al. Treatment of dye wastewater containing acid orange II using a cell with three-phase three-dimensional electrode. Water Res. 2001;35:4226–4230.
- Song Z, Lian F, Yu Z, et al. Synthesis and characterization of a novel MnOx-loaded biochar and its adsorption properties for Cu2+ in aqueous solution. Chem Eng J. 2014;242:36–42.
- Yan J, Han L, Gao W, et al. Biochar supported nanoscale zerovalent iron composite used as persulfate activator for removing trichloroethylene. Bioresour Technol. 2015;175:269–274.
- Fan Z, Zhang Q, Li M, et al. Research, investigating the sorption behavior of cadmium from aqueous solution by potassium permanganate-modified biochar: quantify mechanism and evaluate the modification method. Environ Sci Pollut Res Int. 2018;25:8330–8339.
- Sun C, Zhou R, Jianan E, et al. Magnetic CuO@Fe3O4 nanocomposite as a highly active heterogeneous catalyst of persulfate for 2,4-dichlorophenol degradation in aqueous solution. RSC Adv. 2015;5:57058–57066.
- Wadhawan AR, Livi KJ, Stone AT, et al. Influence of oxygenation on chromium redox reactions with manganese sulfide (MnS(s)). Environ Sci Technol. 2015;49:3523–3531.
- Ahmed MB, Zhou JL, Ngo HH, et al. Progress in the preparation and application of modified biochar for improved contaminant removal from water and wastewater. Bioresour Technol. 2016;214:836–851.
- Ouyang D, Yan J, Qian L, et al. Degradation of 1,4-dioxane by biochar supported nano magnetite particles activating persulfate. Chemosphere. 2017;184:609–617.
- Pang Y, Lei H. Degradation of p-nitrophenol through microwave-assisted heterogeneous activation of peroxymonosulfate by manganese ferrite. Chem Eng J. 2016;287:585–592.
- Li Y, Yang Z, Zhang H, et al. Fabrication of sewage sludge-derived magnetic nanocomposites as heterogeneous catalyst for persulfate activation of Orange G degradation. Colloids Surf A Physicochem Eng Asp. 2017;529:856–863.
- Wang J, Liao Z, Ifthikar J, et al. One-step preparation and application of magnetic sludge-derived biochar on acid orange 7 removal via both adsorption and persulfate based oxidation. RSC Adv. 2017;7:18696–18706.
- Kim C, Ahn JY, Kim TY, et al. Activation of persulfate by Nanosized Zero-Valent Iron (NZVI): mechanisms and transformation products of NZVI. Environ Sci Technol. 2018;52:3625–3633.