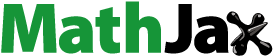
ABSTRACT
The bioconcentration factors and two methods for estimating the bioavailable fraction of Cd and Zn were evaluated to their concentrations in young silage maize under different phosphate and crop management. The DGT technique and the extraction method with NH4NO3 indicated a moderate correlation to Cd levels in maize. After the first crop rotation, Cd bioavailability increased under high-banded P fertilization, indicating a potential accumulation of labile Cd in arable soil in a short period of time. This effect was not visible in the Cd uptake by the following maize crop. P placement strongly affected Zn concentration in maize. A previous legume crop enhanced Cd bioavailability and Cd uptake compared with a wheat crop rotation. Particular attention should be paid to interactions between essential and toxic elements (P, Zn, and Cd), P overfertilization, and high Cd contents in P fertilizers even in the short term to prevent accumulation of labile Cd in soil-maize systems.
Introduction
Silage maize is one of the main crops in Germany, with 2.3 million ha of cultivable land in 2020, and approximately 35% of the harvested maize for biogas production and 65% for livestock feed [Citation1,Citation2]. Phosphorus is a limiting element in crop development due to its low availability in arable soils and its high crop demand [Citation3]. Thus, phosphate-sufficient conditions, usually achieved by P fertilization, are necessary to reach optimal silage maize yields. However, P fertilization can decrease the plant uptake of Zn, an essential micronutrient, and it might incorporate toxic impurities such as Cd in arable land [Citation4,Citation5].
Zn is essential for plant development and survival. It is involved in enzyme activity, gene regulation, and stress tolerance. In agricultural production, cereal crops are often prone to Zn deficiency, reducing the nutrient quality of derived food and feed [Citation6,Citation7]. Conversely, Cd is a hazardous heavy metal without any known metabolic function for living organisms. Its toxicity is enhanced by its high mobility and bioaccumulation potential.
Consequently, high Cd concentrations in crops can decrease leaf size, fresh weight, root and shoot development, leaf photosynthesis, and nutrient absorption, decreasing harvested production [Citation8,Citation9]. In general, soil properties (pH, clay content, organic matter), plant characteristics (species and genotype), crop and fertilizer management (P fertilizer type, P application rate, P placement, and crop rotation) influence the concentration of Cd and Zn in soils and crops [Citation3,Citation10].
Soil measurements, such as the total metal content and pH, can be a helpful tool to assess potential Zn deficiency or hazardous Cd levels for crop uptake [Citation6,Citation11]. Still, not all metal found in soil is available for plants, and its uptake depends mainly on chemical speciation. Evaluating the bioavailable metal forms in soil could be advantageous over measuring only the total metal content [Citation8]. The single extraction methods simulate the plant uptake using chelating agents or neutral salts. Specifically, the extraction with 1 M NH4NO3 solution is an environmentally friendly method employed to determine the readily bioavailable metal fraction in soils and estimate the metal uptake by crops in Germany [Citation12,Citation13].
In soil-plant systems, one of the control mechanisms in metal plant assimilation is the diffusive transport of metals [Citation14,Citation15]. The diffusion gradients in thin films (DGT) technique uses this principle to estimate the bioavailable metal concentration in soils. Several studies have revealed high correlations between the DGT-measured metal levels and the metal concentration in plants [Citation16–19]. Compared to traditional single extraction methods, the DGT devices are user-friendly, with a low amount of lab material and reagents.
Another tool for estimating pollutant exposure and crop uptake is the bioconcentration factor (BCF). The BCF indicates the plant capacity to accumulate a metal into its biomass in comparison to the metal concentration found in soil [Citation20,Citation21]. The BCF for maize has been derived previously, yet its calculation usually focuses on maize grain at mature stages or in polluted sites [Citation22].
Thus, the aims of this study were: i) to assess the bioavailable fraction of Cd and Zn via two different methods (DGT and conventional extraction with 1 M NH4NO3), ii) to analyze the levels of Cd and Zn in silage maize at the leaf development stage and its relation to their bioavailable fraction, and iii) to calculate the BCFs, all under different crop rotations and different P placements and application rates.
Materials and methods
Study design
The study was performed in the framework of a field experiment studying the P fertilizer use efficiency under different P and crop management, with silage maize as the main focus. In 2019, the field experiment was established in Hirrlingen, Baden-Württemberg, Germany (48° 24ʹ N 8° 53ʹ E). The experimental design was a randomized complete block design with 120 plots in total. It included three different crops (silage maize, wheat, and soybean), four replicates (or blocks), two levels of P application rate (low and high) combined with two levels of P placement (broadcasted and banded fertilization), and a control treatment without P fertilization. The low application rate corresponded to 100% of the P required by the crop, and the high application rate was equal to 150% of the P required.
In 2019, 40 out of the 120 plots were sown with soybean (Glycine max cv. Sirelia, n = 20) and summer wheat (Triticum aestivum cv. Quintus, n = 20) as crop rotation. According to the nutrition requirements and the specified P treatments, soybean and summer wheat were fertilized with triple superphosphate (TSP), which had a Cd concentration of 27 mg kg−1, equivalent to 58.69 mg Cd kg−1 P2O5. However, in May 2019, soybean could not recover after hail damage, and winter pea (Pisum sativum cv. James) replaced it in autumn without additional fertilization. For the field season 2020, the following crop rotation, silage maize (Zea mays cv. Ronaldinho) was fertilized with diammonium phosphate (DAP), with a Cd concentration of 22 mg kg−1, corresponding to 47.82 mg Cd kg−1 P2O5. Due to DAP containing N, the application of N as urea was adjusted to adequate levels, depending on the P treatment (). The Cd level in both P fertilizers was close to the limit of 60 mg Cd kg−1 established by the European Union [Citation23].
Table 1. Phosphorus, nitrogen, and potassium requirements for silage maize, field season 2020; a after winter pea cultivation
Soil and maize samples
In 2020, soil and plant samples were collected from the plots where summer wheat and soybean had previously been cultivated (n = 40) (). Before P fertilization and sowing of silage maize, the soil samples were collected, consisting of six randomized core subsamples from each plot at 0 to 30 cm depth. The soil subsamples were mixed, air-dried, and sieved (2 mm) to obtain a homogenous sample of each plot.
Shoots and roots of silage maize (n = 5) were collected from each of the 40 plots at the leaf development stage (BBCH 17). Subsequently, the material was rinsed with H2O (electrical resistance <18.2 MΩ cm−1) and oven-dried at 75 ± 5°C for 48 h (Heraeus UT 6760, Thermo Scientific, Hanau, Germany). The plant samples were pulverized in a mixer mill MM 400 Retsch (Verder Scientific, Haan, Germany), operated with a metal-free jar at 29 Hz for 1.5 min.
Sample pretreatment and analytical measurements
The CaCl2 method for soil pH measurement, the aqua regia extraction for (pseudo) total metal concentration in soil samples, the pretreatment with microwave digestion for total metal concentration in maize samples, and the analytical technique with Inductively Coupled Plasma combined with Mass Spectrometry (ICP-MS) were all performed following standardized methods, using chemical reagents of analytical grade and H2O with an electrical resistance lower than 18.2 MΩ cm−1 by the Core Facility of the University of Hohenheim (CFH), Stuttgart, Germany [Citation24].
For the aqua regia extraction, soil samples (3 g) were moistened with C8H₁₈O in 250 ml digestion tubes. The soil samples were saturated with aqua regia solution (78% HCl and 22% HNO3, 50 ml). Next, the suspension was digested at 140°C for 3 h in a reflux condenser and, later, filtered through a folded filter MN 280 1/4 into 100 ml vessels. The heavy metal concentrations were measured using an ICP-MS NexION 300x (PerkinElmer, Rodgau, Germany).
For the pretreatment with microwave digestion, the plant samples (0.2 g) were saturated with H2O (1 ml) and HNO3 (2.5 ml) and digested for 1.5 h by a Milestone UltraCLAVE III microwave heated digestion reactor (MLS GmbH, Leutkirch, Germany) [Citation25]. After digestion, H2O was added to each sample solution to reach 10 ml for further analytical analysis via ICP-MS.
Metal bioavailability
For the methods assessing the bioavailable fraction, H2O had an electrical resistance lower than 18.2 MΩ cm−1, all chemical reagents were of analytical grade, and all material was previously rinsed with HNO3 and H2O. The first method was the single extraction with 1 M NH4NO3 solution [Citation13,Citation26]. In an Erlenmeyer flask, each soil sample (20 g) was moistened with 1 M NH4NO3 solution (50 ml). The soil-extractant solution was homogenized for 2 h at 120 rpm in a horizontal shaker (GFL® model 2017) and transferred to plastic containers through a 0.45 µm SFCA filter (VWRTMR). After filtration, the solution was stabilized with HNO3 at 1% of the extraction volume. The samples were stored at 4 ± 1°C until the metal analysis via ICP-MS was completed by the CFH.
The DGT technique was the second procedure to estimate the bioavailable metal fraction [Citation16–18]. Each DGT device consisted of a) one filter membrane made of polyethersulphone, b) one binding layer of Chelex gel made of iminodiacetate with a thickness of 0.40 mm, and c) one diffusive gel made of agarose crosslinked polyacrylamide with a thickness of 0.78 mm and exposure area of 2.54 cm2 according to the data provided by DGT Research Ltd. (Lancaster, UK). Before deployment, each soil sample (50 g) was saturated with H2O in a glass container and covered with Parafilm®. After 24 h at room temperature (25°C), the DGT device was pressed onto the saturated paste, with a deployment time of 24 h. Next, the DGT devices were rinsed with H2O, and after removing the Chelex gel from the DGT device with metal-free tweezers, the Cd attached to it was extracted by immersing the gel into a clean tube with 1 M HNO3 solution (1 ml) for 24 h [Citation27]. Subsequently, the gel was removed from the solution, and the samples were stored at 4 ± 1°C until the CFH completed the metal analysis via ICP-MS.
Equations
The time-averaged concentrations of Cd and Zn by the DGT technique were estimated with EquationEquation (1)(1)
(1) [Citation28]:
where is the thickness of the diffusive layer (0.92 mm),
is the diffusion coefficient of Cd and Zn in the diffusive layer (6.09 x 10−6 and 6.08 × 10−6 cm2/s at 25°C, correspondingly),
represents the surface area of the DGT device (2.54 cm2), and
is the deployment time (24 h). The accumulated mass (M) of Cd and Zn was estimated using EquationEquation (2)
(2)
(2) .
where is the metal concentration measured in the eluent (µg l−1),
is the volume of the binding gel (0.20 ml),
is the volume of the eluent (1 ml), and
corresponds to the elution factor with the value of 0.85, according to Devillers et al. [Citation29].
For the BCF, the total concentrations of Cd and Zn in soil and silage maize were employed. The BCF values for roots and shoots for Cd and Zn were calculated with EquationEquation (3)(3)
(3) [Citation20,Citation21]:BCFroots or shoots
Data analysis
The normality of the data distribution, analysis of variance (ANOVA), post-hoc test at 0.05 level of significance, and the Pearson correlations were conducted using the software RStudio® version 1.3.1093. The linear model included the soil measurements, the metal concentration in silage maize, and the BCF as numerically dependent variables. In contrast, the independent variables were block division, previous crop rotation, P rate, and P placement. Although the soil sampling was done before P fertilization, P placement and P rate were part of the model as the previous crop rotation had the same treatments. Furthermore, the interaction between P placement and P rate was considered for the modeling.
Results
Soil pH
Soil pH was on average 5.79 units before the maize growing season, indicating slightly acidic soil conditions. The ANOVA results indicated that the block division and the previous crop rotation affected the soil pH response at a p < 0.01 (). There was a gradient from block I to block IV, with a difference of one pH unit between the first and the last block. The soil where winter pea was previously grown had a significantly lower pH than the soil where summer wheat was grown.
Table 2. Soil measurements by variable (n = 40); significance code for p-value: ***<0.001, **<0.01, *<0.05, ns >0.05; different letters indicate significant differences at p < 0.05 level (Tukey test)
Total metal concentration in soil
The P treatments did not reveal any influence on the total Cd concentration in soil. However, the banded fertilization had a higher Cd level than soil from the other P placements (p = 0.06). Neither the P treatments nor the previous crop rotation significantly influenced the total Zn concentration in soil. The only parameter affecting the total Zn concentration in soil was the block division ().
Bioavailable fraction in soil
Regardless of P placement and P rate not affecting the bioavailable Cd fraction, there was a significant interaction between these at a p < 0.05 for both methods (). The high-banded treatment showed the highest bioavailable Cd concentration, and the lowest was found in the low-banded fertilization (). However, the application rate did not indicate a clear tendency for the broadcasted fertilization and depended mainly on the method used. Concerning the block division, block IV had a significantly lower bioavailable Cd concentration than the rest of the blocks. Moreover, the identity of previously cultivated crops affected the bioavailable Cd fraction. The soil where wheat was grown had a significantly lower Cd concentration as estimated by means of DGT than soil where winter pea was cultivated. However, this difference was not visible in the Cd amount extracted by NH4NO3 ().
Figure 2. Interaction effect between P placement and P rate for bioavailable Cd concentration by (a) Extraction with 1 M NH4NO3 solution and (b) DGT technique
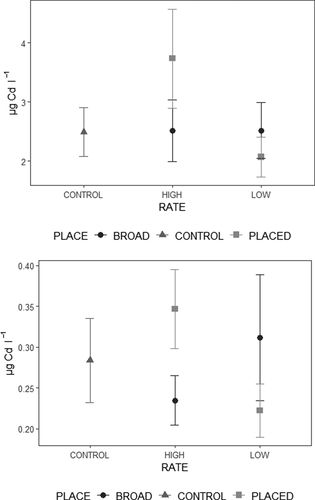
Neither P placement nor P rate impacted the bioavailable Zn concentration in soil. However, the P rate had a probable effect at p < 0.1, with the highest bioavailable Zn concentration determined by DGT in the high application rate. According to the DGT technique, the highest bioavailable Zn concentration occurred in the high application rate and banded fertilization treatment. In contrast, the high-broad treatment had the lowest Zn concentration measured by DGT. For the bioavailable Zn concentration estimated via NH4NO3, no interaction between placement and application rate was observable ().
Silage maize
For the total Cd concentration in the roots, the fertilizer placement had a significant influence at p = 0.05. Although a higher Cd concentration in roots occurred in the broadcasted fertilization than in the other treatments, the post-hoc test did not reveal significant differences between the P placements. For the total Cd concentration in shoots, the differences between the P placements were not significant and marginal ().
Table 3. Amounts of Cd and Zn in young silage maize (mg kg−1 dry weight) by variable (n = 40); significance code for p-value: ***<0.001, **<0.01, *<0.05, ns >0.05; different letters indicate significant differences at p < 0.05 level (Tukey test)
Similar to Cd, the total Zn concentration in silage maize was analyzed in roots and shoots. The P placement led to lower Zn concentration both in roots and shoots in the banded fertilization than in the other treatments. The highest Zn concentration in roots was found in the control treatment, while the highest Zn concentration in shoots was found in the broad placement ().
The crop cultivated before silage maize affected the total Zn concentration in roots and shoots (p < 0.01), with a higher value in maize following summer wheat than in maize following winter pea for both plant fractions. Regarding the block division, block IV had a significantly lower Cd concentration in roots and shoots and a lower Zn concentration in roots than the other blocks.
Pearson correlations
The correlation between the Cd concentration in shoots and the Cd concentration determined by NH4NO3 was higher than the correlation between the Cd concentration found in shoots and the Cd concentration extracted by the DGT technique. Both methods were highly correlated to soil pH. However, the correlation between soil pH and the Cd concentration estimated by NH4NO3 was stronger than that of soil pH and the Cd concentration determined by DGT. The correlation between the Cd concentration in roots and both extraction methods was significant and similar. The Cd concentration estimated by DGT correlated better with the Cd concentration in roots than with the Cd found in shoots. For the Cd concentration in shoots, the correlation with the Cd concentration using NH4NO3 was higher and more significant than the Cd concentration estimated by DGT (). Regarding Cd concentration in silage maize, there was no correlation between the Cd found in roots and shoots. Still, both were significantly associated with soil pH.
Figure 3. Correlation matrix between metal concentration in soil and metal concentration in silage maize of (a) Cd and (b) Zn (n = 40), significant correlation at p-value: ***<0.001, **<0.01, *<0.05
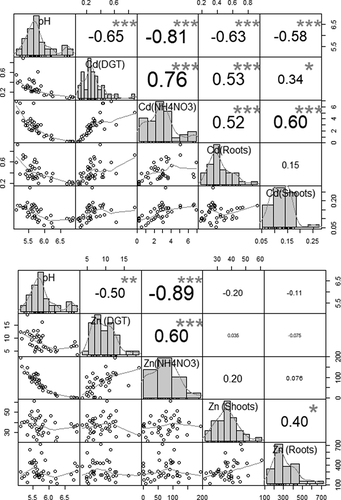
The Zn concentration determined by DGT indicated a high correlation with the Zn concentration measured by the traditional method. However, the correlation of both methods with the Zn found in silage maize was low and insignificant. Similar to Cd, both methods significantly correlated to soil pH ().
BCF
The values for both metals indicated a BCFroots>1. The highest BCFroots of Cd was found in the broadcasted fertilization, while the lowest BCFroots of Cd was visible in the banded fertilization (). The banded fertilization revealed significantly low BCFshoots of Cd in comparison with the other treatments. The P rate affected the BCFshoots of Cd at p < 0.05, yet after running the post hoc test, the differences between the application rates were not significant.
Table 4. BCF of Cd and Zn for young silage maize by field variable (n = 40); significance code for p-value: ***<0.001, **<0.01, *<0.05, ns >0.05; different letters indicate significant differences at p < 0.05 level (Tukey test)
The control treatment had the highest BCFroots of Zn, while the high application rate treatment had a significantly lower BCFroots of Zn than the other treatments. Despite no significant differences between the P placements for the BCFroots of Zn, the banded fertilization had a lower BCFroots value than the other treatments. The BCFshoots of Zn was significantly lower in the banded fertilization than in the control and the broadcasted treatment ().
For the BCFroots, the crop rotation had a significant effect (p < 0.001): maize following winter pea had a higher BCFroots of Cd than maize following wheat. The BCFroots of Zn indicated the opposite behavior, with a higher value in maize following wheat than maize following winter pea. However, for the BCFshoots, higher values for Cd and Zn were found in maize following wheat than in maize following winter pea.
Discussion
Soil pH
The ANOVA results indicated that the previous P fertilization did not impact soil pH, likely due to the lack of influence of TSP in soil pH compared to other P fertilizers containing N or under polluted soil conditions [Citation30]. An increasing soil pH gradient was visible from block I to block IV. This last block had the highest soil pH, the lowest bioavailable Cd fraction, and the lowest Cd concentration in roots and shoots, indicating a significant heterogeneity between blocks [Citation31]. A higher clay or organic matter content in the soil of block IV could explain the high pH and the low Cd mobilization in this soil [Citation22,Citation32]. However, the significant differences, especially between block IV and the rest of the blocks, could have masked P fertilization’s effects on soil pH and the other variables of interest.
The crop rotation caused a lower soil pH when winter pea was previously grown than when wheat was cultivated before. The soybean cultivated in spring and the field pea cultivated in winter 2019 could have decreased soil pH by the nitrification process, causing a higher Cd mobilization and uptake by maize following the legume cultivation, as has also been shown by Yan et al. [Citation33].
Total metal concentration in soil
Although the P placement did not strongly affect the total Cd concentration in soil, the banded treatment had the highest total Cd concentration. In 2019, the background concentration of total Cd in soil was 0.145 ± 0.002 mg kg−1, with a visible increase in 2020. The increase in Cd level could result from P fertilization in plots where the previous crops were fertilized with relatively high Cd-TSP [Citation34,Citation35]. However, the total Cd concentration remained in the range of unpolluted soils (0.06 to 1.10 mg kg−1) and below the limit specified by German standards (0.40 mg Cd kg−1 at pH<6) [Citation10,Citation36].
The average total Zn concentration in soil was 60.84 ± 1.87 mg kg−1, in accordance with the average value for loamy-silty soils in Germany [Citation6]. Like for Cd, neither the P treatments nor the previous crop rotation affected the total Zn concentration in soil. Although the mobile fraction exhibited significant differences between the P treatments, this Zn fraction is usually low compared to the total Zn concentration [Citation7]. In this study, the bioavailable Zn fraction represented only 0.30%, explaining the lack of impact of P fertilization on the total Zn concentration in soil. The same explanation could be valid for the total Cd concentration in soil since the bioavailable Cd concentration identified by NH4NO3 represented only 3.5% of the total Cd concentration, a low percentage compared to the mobile Cd fraction (45.2%) reported by Liu et al. [Citation37].
Bioavailable fraction in soil
Before the field trial, the bioavailable Cd concentration measured by the conventional extraction method was 1.50 ± 0.66 µg l−1 in 2019. The values obtained after the first crop rotation were higher than the background bioavailable Cd concentration. The increase indicated a change in the total and bioavailable Cd levels in the control treatment, possibly resulting from atmospheric Cd deposition [Citation38]. Still, the high rate combined with the banded fertilization led to the highest bioavailable Cd in soil with a p < 0.05, independently of the assessment method. Römkens [Citation34] demonstrated that P fertilizer containing >40 mg Cd kg−1 P2O5 could trigger Cd accumulation in arable land; still, this increase usually takes longer, and changes in the bioavailable fraction are undetectable after one year of cultivation under greenhouse conditions. In contrast, Molina-Roco et al. [Citation35] revealed that the labile Cd found in soil after fertilization corresponds to the labile Cd found in TSP. Additionally, freshly applied Cd derived from TSP fertilization can experience an immediate low uptake by the crop (summer wheat and winter pea), and Cd might stay mobile and potentially bioavailable in the soil for the follow-up crops (silage maize) [Citation5]. Thus, the Cd derived from P fertilizer, combined with the excess of fertilization in the high banded treatment, could explain the fast change in the bioavailable Cd fraction in soil.
The low Cd concentration estimated by DGT in soils where summer wheat was grown compared to winter pea could result from wheat taking up more Cd than other crops [Citation11]. Furthermore, the soybean crop and its following substitution with another legume could have decreased soil pH [Citation22], leading to a higher Cd bioavailability in soil. Interestingly, this crop rotation effect was not visible in the Cd extracted using NH4NO3, a method usually more dependent on soil pH [Citation12].
The bioavailable Zn fraction obtained by DGT was high under high-banded and low-broad fertilization. The antagonistic behavior between P and Zn in nutrient uptake by previous crops could lead to an accumulation of bioavailable Zn in the P fertilized plots [Citation39]. Additionally, the Zn concentration was around 600 mg kg−1 in the TSP applied to soybean and wheat, explaining the high bioavailable Zn concentration estimated by DGT in the high-banded fertilization [Citation10]. However, there was no significant increase in the total Zn concentration in the soil after TSP fertilization.
Silage maize
The Cd concentration in shoots was relatively high but below the limit for feed materials of vegetable origin (1 mg kg−1) [Citation40]. The control treatment had the highest total Cd concentration in shoots, probably due to the lack of P fertilization and dilution effect [Citation41]. The low Cd concentration in shoots in the banded fertilization could result from high P uptake, with a consequently higher biomass, diluting the Cd concentration in the plant [Citation30]. Nevertheless, a relatively high Cd concentration in roots was visible in the same treatment. This high Cd level in roots could be due to the relatively high total and bioavailable Cd concentration in soil detected in the banded fertilization [Citation42].
The Zn concentration in roots was high compared to the Zn concentration in shoots. However, the Zn level in shoots was in the upper range of the optimum Zn concentration in plants (12 to 47 mg kg−1). This distribution might result from maize sensitivity to Zn and accumulating most of it in the roots [Citation6,Citation10]. Although the Zn level in soil and young maize shoots was adequate, the banded fertilization effect on the Zn uptake by roots and shoots of silage maize was strongly evident. Furthermore, the bioavailable Zn concentration estimated by DGT was higher under this type of fertilization. The P fertilization could cause this difference between the bioavailable Zn fraction and the Zn concentration in maize by diluting this micronutrient within the plant or depressing its uptake [Citation4,Citation43].
Regarding the crop rotation, a lower Zn concentration in maize roots and shoots following winter pea than in maize following wheat could be due to winter pea taking up high Zn quantities, causing a lower Zn concentration in the follow-up crop [Citation44]. However, none of the methods assessing the bioavailable Zn fraction indicated lower values after the legume cropping.
The interaction between P application rate and P placement influenced the bioavailable Cd fraction obtained by both methods, suggesting significant changes in the soil after only one crop cultivation under moderate Cd-P fertilizer. Nevertheless, this interaction effect was not yet visible in the maize roots and shoots. The interactions between Cd, Zn, and P in the uptake process and the lack of significant changes for Cd uptake by the crop after one application of P fertilizer could explain the differences between the bioavailable fraction and the maize measurements [Citation5,Citation34].
Pearson correlations
The traditional method indicated a significantly stronger correlation with the Cd concentration in shoots than the DGT technique, probably caused by a more complete extraction with NH4NO3 under acidic soil conditions or due to plant limiting uptake mechanisms rather than diffusion [Citation12,Citation15]. The correlation between the Cd concentration in shoots and the bioavailable Cd fraction estimated by DGT indicated lower values than other studies [Citation16,Citation17,Citation19]. Still, the DGT technique is independent of soil pH resulting in a better correlation with maize uptake under alkaline and neutral soils than the traditional method [Citation45]. Furthermore, the bioavailable Cd concentration measured by DGT had a higher correlation with the Cd concentration in roots than with the Cd found in shoots. This finding agrees with the DGT principle: the diffusive transportation to the plant roots is the regulating mechanism in metal uptake [Citation14].
Since the correlation coefficients were low, none of the bioavailability methods seemed suitable to predict the Zn uptake by silage maize. Our results contradicted the study from Meer et al. [Citation46], where the Zn concentration in bean shoots highly correlated with the Zn extracted by NH4NO3. The P fertilization was performed between the collection of soil samples and the collection of plant samples, explaining the low correlation between the bioavailable Zn fraction and the Zn concentration in silage maize. However, the methods assessing bioavailability might not simulate the suppressant effect of P in Zn uptake by plants [Citation47]. In addition, the DGT method has been proved unsuitable as a surrogate of Zn content in other cereal crops, such as wheat [Citation18].
BCF
The BCFroots from both metals indicated a relatively high accumulation of Cd and Zn in relation to the total metal concentration in the soil with values >1.0. The broad fertilization showed the highest BCFroots of Cd, probably by the combination of relatively low biomass production and the uptake of Cd, derived from P fertilizer. The P fertilization rate significantly affected the BCFroots of Zn, exhibiting lower values in the high application rate. This significant effect of the P rate was not visible in the soil and plant analyses, providing valuable information compared with the soil and maize measurements.
In general, BCFshoots of Cd was lower than 1.0, indicating a weak response of aerial biomass to the metal concentration in the soil. Meanwhile, the BCFroots of Cd was >1.0, suggesting a higher response of the maize roots to the Cd concentration in soil but a low Cd translocation from roots to shoots [Citation9,Citation48]. The lowest BCFshoots of Cd, similar to the BCFroots of Cd, occurred in the banded fertilization, possibly resulting from a dilution effect [Citation41]; or by coupled uptake with Zn that also had the lowest BCF in the banded fertilization [Citation10]. In general, the BCFshoots of Zn were low, and the BCFroots of Zn were high. This behavior might be due to competition between P and Zn in the translocation process from roots to stems. However, Drissi et al. [Citation4] already discarded this hypothesis and attributed the allocation to a dilution effect in silage maize.
The BCFshoots of Cd suggested that the previous summer wheat enabled a higher Cd transfer from the soil to the maize shoots. The BCFshoots of Zn was also higher in maize following wheat, suggesting a synergistic behavior between Cd and Zn [Citation10]. Still, the BCFroots of Cd, the bioavailable Cd fraction, and the Cd uptake by roots were lower in the wheat rotation. The Cd distribution after wheat cultivation could be explained by wheat taking up more Cd than other crops [Citation11], leading to lower Cd bioavailability and lower root uptake for the next crop, e.g. silage maize. At the same time, soil pH was lower under the winter pea rotation, likely by the nitrification process, leading to a higher Cd bioavailability in soil and uptake in maize roots following the cultivation of legumes [Citation33].
Conclusion
The DGT technique and the traditional method indicated a moderately positive correlation with the Cd concentration found in young maize roots. Still, the extraction employing NH4NO3 might be more suitable for predicting Cd levels in young maize shoots. None of the methods indicated a strong correlation between the bioavailable Zn fraction and maize Zn levels.
The P placement strongly affected Zn concentration in maize roots and shoots. Assessing the BCF of Zn provided helpful information about the effects of the P application rate that were not evident by assessing only the metal concentration in silage maize.
The previous crop rotation, the interaction between Cd and Zn, and the P fertilization effect on Zn uptake seem to be critical drivers for Cd uptake by young silage maize. The methods assessing the bioavailable metal fraction might overlook some of these interactions. Still, both methods demonstrated the potential accumulation of labile Cd in arable soil by overfertilization in a relatively short time. Further investigation is needed to understand the interactions between Cd, Zn, and P in soil-maize systems. Special attention should be paid to P fertilizer and crop management and the Cd contents in P fertilizers to avoid labile Cd accumulation in non-polluted soils and maintain adequate Zn levels in silage maize.
Disclosure statement
No potential conflict of interest was reported by the author(s).
Additional information
Funding
References
- Statistisches Bundesamt. Ackerland nach Hauptnutzungsarten und Kulturarten: Feldfrüchte und Grünland; 2020.
- FNR. Bioenergy in Germany Fact and Figures 2020; 2019.
- Niño-Savala AG, Zhuang Z, Ma X, et al. Cadmium pollution from phosphate fertilizers in arable soils and crops: an overview. Front Agric Sci Eng. 2019;6(4):419.
- Drissi S, Houssa A, Bamouh A, et al. Effect of zinc-phosphorus interaction on corn silage grown on sandy soil. Agriculture. 2015;5(4):1047–1059.
- Bracher C, Frossard E, Bigalke M, et al. Tracing the fate of phosphorus fertilizer derived cadmium in soil-fertilizer-wheat systems using enriched stable isotope labeling. Environ Pollut. 2021;287:117314.
- Alloway BJ. Soil factors associated with zinc deficiency in crops and humans. Environ Geochem Health. 2009;31(5):537–548.
- Gupta N, Ram H, Kumar B. Mechanism of Zinc absorption in plants: uptake, transport, translocation and accumulation. Rev Environ Sci Bio/Technol. 2016;15(1):89–109.
- Khan MA, Khan S, Khan A, et al. Soil contamination with cadmium, consequences and remediation using organic amendments. Sci Total Environ. 2017;601-602:1591–1605.
- Bashir S, Bakhsh Gulshan A, Iqbal J, et al. Comparative role of animal manure and vegetable waste induced compost for polluted soil restoration and maize growth. Saudi J Biol Sci. 2021;28(4):2534–2539.
- Kabata-Pendias A, Szteke B. Trace elements in abiotic and biotic environments: cadmium [Cd, 48]; Zinc [Zn, 30]. Boca Raton: CRC Press; 2015.
- Zhuang Z, Niño-Savala AG, Mi Z-D, et al. Cadmium accumulation in wheat and maize grains from China: interaction of soil properties, novel enrichment models and soil thresholds. Environ Pollut. 2021;275:116623.
- Adamo P, Agrelli D, Zampella M. Chemical speciation to assess bioavailability, bioaccessibility and geochemical forms of potentially toxic metals (PTMs) in polluted soils. de Vivo B, Belkin HE, Lima A editors, Environmental geochemistry: site characterization, data analysis and case histories 2nd, Amsterdam, the Netherlands: Elsevier, 2018. 153–194. DOI:https://doi.org/10.1016/B978-0-444-63763-5.00010-0
- Deutsches Institut für Normung e.V. DIN ISO 19730 Bodenbeschaffenheit – extraktion von Spurenelementen aus Böden mit Ammoniumnitratlösung (ISO 19730:2008); Berlin: Beuth Verlag GmbH, 2009.
- Degryse F, Smolders E, Zhang H, et al. Predicting availability of mineral elements to plants with the DGT technique: a review of experimental data and interpretation by modelling. Environ Chem. 2009;6(3):198.
- Li C, Ding S, Yang L, et al. Diffusive gradients in thin films: devices, materials and applications. Environ Chem Lett. 2019;17(2):801–831.
- Pérez AL, Anderson KA. DGT estimates cadmium accumulation in wheat and potato from phosphate fertilizer applications. Sci Total Environ. 2009;407(18):5096–5103.
- Yao Y, Sun Q, Wang C, et al. The combination of DGT technique and traditional chemical methods for evaluation of cadmium bioavailability in contaminated soils with organic amendment. Int J Environ Res Public Health. 2016;13:6.
- Grüter R, Costerousse B, Mayer J, et al. Long-term organic matter application reduces cadmium but not zinc concentrations in wheat. Sci Total Environ. 2019;669:608–620.
- Luo H, Du P, Shi J, et al. DGT methodology is more sensitive than conventional extraction strategies in assessing amendment-induced soil cadmium availability to rice. Sci Total Environ. 2021;760:143949.
- Retamal-Salgado J, Hirzel J, Walter I, et al. Bioabsorption and bioaccumulation of cadmium in the straw and grain of maize (Zea mays L.) in growing soils contaminated with cadmium in different environment. Int J Environ Res Public Health. 2017;14(11):1399.
- Ramana S, Tripathi AK, Kumar A, et al. Evaluation of Furcraea foetida (L.)Haw. for phytoremediation of cadmium contaminated soils. Environ Sci Pollut Res Int. 2021;28(11):14177–14181.
- Wang S, Wu W, Liu F, et al. Accumulation of heavy metals in soil-crop systems: a review for wheat and corn. Environ Sci Pollut Res Int. 2017;24(18):15209–15225.
- Ulrich AE. Cadmium governance in Europe’s phosphate fertilizers: not so fast? Sci Total Environ. 2019;650(Pt 1):541–545.
- Deutsches Institut für Normung e.V. DIN ISO 11466: 1997-06Bodenbeschaffenheit - Extraktion in Königswasser löslicher Spuren elemente; Berlin: Beuth Verlag GmbH, 1997.
- VDLUFA. Methodenbuch VII Umweltanalytik. 4th ed. Darmstadt: VDLUFA-Verl; 2011.
- Bashir S, Salam A, Chhajro MA, et al. Comparative efficiency of rice husk-derived biochar (RHB) and steel slag (SS) on cadmium (Cd) mobility and its uptake by Chinese cabbage in highly contaminated soil. Int J Phytoremediation. 2018;20(12):1221–1228.
- Yao Y, Sun Q, Wang C, et al. Evaluation of organic amendment on the effect of cadmium bioavailability in contaminated soils using the DGT technique and traditional methods. Environ Sci Pollut Res Int. 2017;24(9):7959–7968.
- DGT research Ltd. LSNM-NP Loaded DGT device for metals (A) in solution; 2019.
- Devillers D, Buzier R, Charriau A, et al. Improving elution strategies for Chelex®-DGT passive samplers. Anal Bioanal Chem. 2017;409(30):7183–7189.
- Sarwar N, Malhi SS, Zia MH, et al. Role of mineral nutrition in minimizing cadmium accumulation by plants. J Sci Food Agric. 2010;90(6):925–937.
- Soriano-Disla JM, Gómez I, Navarro-Pedreño J, et al. The transfer of heavy metals to barley plants from soils amended with sewage sludge with different heavy metal burdens. J Soils Sediments. 2014;14(4):687–696.
- Malik KM, Khan KS, Rukh S, et al. Immobilization of Cd, Pb and Zn through organic amendments in wastewater irrigated soils. Sustainability. 2021;13(4):2392.
- Yan F, Schubert S, Mengel K. Soil pH changes during legume growth and application of plant material. Biol Fertili Soils. 1996;23(3):236–242.
- Römkens P, Rietra R, Kros H, et al. 2018 Impact of cadmium levels in fertilisers on cadmium accumulation in soil and uptake by food crops: Report 2883.
- Molina-Roco M, Escudey M, Antilén M, et al. Distribution of contaminant trace metals inadvertently provided by phosphorus fertilisers: movement, chemical fractions and mass balances in contrasting acidic soils. Environ Geochem Health. 2018;40(6):2491–2509.
- LUFA. Vorsorge-, Prüf-und Maßnahmenwerte für Boden gemäß Bundes-Bodenschutz-und Altlastenverordnung (BBodSchV); 1999.
- Liu G, Tao L, Liu X, et al. Heavy metal speciation and pollution of agricultural soils along Jishui River in non-ferrous metal mine area in Jiangxi Province, China. J Geochem Explor. 2013;132:156–163.
- Ilyin I, Travnikov O, Schütze G, et al. Country-scale assessment of heavy metal pollution: a case study for Germany. Technical Report 1/2020; 2020.
- Mousavi SR. Zinc in crop production and interaction with phosphorus. Aust J Basic Appl Sci. 2011;5(9):1503–1509.
- European Parliament. Directive 2002/32/EC of the European Parliament and of the Council on undesirable substances in animal feed (Annex I); Brussels, Belgium, 2013.
- Chien SH, Menon RG. Dilution effect of plant biomass on plant cadmium concentration as induced by application of phosphate fertilizers. Rodriguez-Barrueco C editor. Fertilizers and environment. Springer Netherlands: Dordrecht. 1996. 437–442. DOI:https://doi.org/10.1007/978-94-009-1586-2_74
- Lux A, Martinka M, Vaculík M, et al. Root responses to cadmium in the rhizosphere: a review. J Exp Bot. 2011;62(1):21–37.
- Bogdanovic D, Ubavic M, Cuvardic M. Effect of phosphorus fertilization on Zn and Cd contents in soil and corn plants. Nutr Cycling Agroecosyst. 1999;54(1):49–56.
- Płaza A, Gąsiorowska B, Rzążewska E. Heavy metal content in the green fodder of field pea/oat mixtures destined for cattle feed. Environ Monit Assess. 2019;191(11):680.
- Dai Y, Nasir M, Zhang Y, et al. Comparison of DGT with traditional methods for assessing cadmium bioavailability to Brassica chinensis in different soils. Sci Rep. 2017;7(1):14206.
- Meers E, Samson R, Tack F, et al. Phytoavailability assessment of heavy metals in soils by single extractions and accumulation by Phaseolus vulgaris. Environ Exp Bot. 2007;60(3):385–396.
- Sönmez O, Pierzynski G, Kaya C, et al. The effects of phosphorus addition on phytoavailability of zinc by diffusive gradients in thin films (DGT). Turk J Agric For. 2016;40:379–385.
- Mirecki N, Agič R, Ljubomir Š, et al. Transfer factor as indicator of heavy metals content in plants. Fresenius Environ Bull. 2015;24:4212–4219.