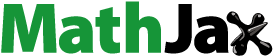
ABSTRACT
Heavy metals were typical contaminants in aquatic ecosystems, while their effects on the biotoxicity and metabolism of algal cells in the presence of organic ligands were still unclear. In this study, the contrasting effects of Cu and humic acids (HA) on the growth inhibition, oxidative stress and extracellular secretion of microalga Chlorella vulgaris were studied at environmentally relevant concentrations of Cu (0~2 mg/L) and HA (0~20 mg/L). Results showed that, with increased Cu addition, the algal growth rate decreased while concentrations of superoxide dismutase and malondialdehyde increased showing evident growth inhibition and oxidative damage in the sole presence of Cu. However, the presence of HA could significantly alleviate the Cu-induced toxicity damage, and higher concentrations of HA exhibited greater alleviation efficiencies. Fluorescence spectroscopy combined with flow field flow fractionation revealed that secretion of 50 kDa~0.45 μm of extracellular protein-like substances was the critical fractions responsible for the biotoxicity alleviation. Analysis of Cu contents within extracellular polymeric substance (EPS) matrix showed that not only the secretion amounts but also the acquisition capacities of EPS matrix decreased due to the addition of HA. This study benefited an in-depth understanding toward the toxicity of heavy metals to aquatic microorganisms in natural waters that contained abundant organic ligands.
1. Introduction
In recent decades, heavy metal pollution has become a global environmental issue and the prime focus of environmental security [Citation1–3]. It was reported that the total amounts of heavy metals released to environment in the second half of the twentieth century reached about 22,000 ton of Cd (II), 939,000 ton of Cu (II), 783,000 ton of Pb (II), and 1,350,000 ton of Zn (II) [Citation1,Citation4]. The accumulation of heavy metals in the aquatic environment poses a potential risk to not only aquatic organisms (e.g. microalgae and zooplankton) but also human health [Citation5–8]. For example, although low levels of Cu and Cd exhibited no apparent harm to the metabolism of Alexandrium catenella, the growth system was highly inhibited as the heavy metal concentration exceeded 10−7 M [Citation9].
The toxicity process of heavy metals to microorganisms consists of adsorption onto the cell surface as well as penetration into the cell interior, which induce variations in oxidative stress and extracellular secretion [Citation10]. Extracellular polymeric substances (EPS), produced via various approaches including excretion, secretion, sorption and cell lysis, consisted mainly of proteins, polysaccharides, humic substances and lipids, etc [Citation11,Citation12]. EPS can physically serve as a layer between organisms and the surrounding environment, which resultantly plays a crucial role in resisting the adverse effects induced by various stressors. For instance, Xie et al. (2020) proved that complexation with EPS could significantly reduce the toxicity of Cd to C. vulgaris. Moreover, the nanotoxicity of ZnO nanoparticles can induce C. vulgaris to secret more dissolved organic carbon, polysaccharides and proteins for cell protection [Citation13].
The toxicity of heavy metals depends on their speciation and the medium in which they were involved. Dissolved organic ligands were ubiquitous in nature ecosystems, which exhibited critical effects on the speciation and toxicities of heavy metals via the complexation/adsorption process due to its functional groups such as carboxyl, hydroxyl and epoxide functional groups [Citation1,Citation14,Citation15]. For instance, organic ligands were found to increase the toxicity of heavy metals to bacteria, ciliate and marine diatom [Citation2,Citation16]. In addition, it was reported that the toxicity and bioaccumulation of heavy metals released from nanoparticles would be promoted in the presence of organic ligands [Citation17–19]. On the contrary, other studies found the relieved toxicity of heavy metals to organism in the presence of ligands [Citation10,Citation20]. It should be noted that the specific effects of heavy metals were highly dependent on the chemical speciation, the organisms conducted and the medium conditions (e.g. pH and organic ligands) [Citation1,Citation10]. Although metal toxicity to microorganism was widely studied, these studies focused mainly on the variations in algal biomass as well as the adsorption/bioaccumulation mechanisms of heavy metals to algal cells. Few attentions were paid to the co-influence of heavy metals and organic ligands on algal growth, emphasizing the changes of antioxidant enzyme activities and the secretion properties of EPS matrix.
In this study, the contrasting effects of heavy metals and organic ligands on the growth inhibition, oxidative stress and extracellular secretion of microalga Chlorella vulgaris were explored. Cu was applied as the typical heavy metal due to its comprehensive detection in aquatic ecosystems and relatively high concentration (1.07~3400 μg/L) [Citation21,Citation22]. Humic acid (HA) was used as the representative organic ligands owing to its obvious influences on the speciation, bioavailability and fate of metals [Citation1,Citation23,Citation24]. The activities of oxidative enzymes, including superoxide dismutase and malondialdehyde, were determined to test the changes of oxidative stress under each treatment. Variations in contents, compositions and molecular weight distribution of EPS matrix under different conditions were analyzed via physicochemical determination combined with fluorescent spectroscopy and flow field-flow fractionation (FlFFF). Results obtained may benefit a deeper understanding of the toxicity of heavy metals to the microorganism in some natural waters that contained high concentrations of dissolved organic ligands.
2. Material and methods
2.1. Microalgae culture conditions and exposure test
Chlorella vulgaris (FACHB-6) was purchased from the Institute of Hydrobiology, Chinese Academy of Sciences. The experimental alga was cultivated in BG-11 medium, which consisted of CaCl2 · 2H2O (15 mg/L), KH2PO4 (1.6 mg/L), MgCl2 · 6H2O (12 mg/L), MgSO4 · 7H2O (15 mg/L), Na2CO3 (15 mg/L), Na2EDTA·2H2O (0.1 mg/L), ZnCl2 (3 μg/L), CuCl2 · 2H2O (0.01 μg/L), FeCl3 · 6H2O (80 μg/L), CoCl2 · 6H2O (1.5 μg/L), NaMoO4 · 2H2O (7 μg/L) and NH4Cl (15 mg/L) [Citation25]. The incubation temperature was 25°C and the light illumination was 130 μmol photons m−2s−1, with light/dark regime of 12 h/12 h and regular shaking (three times a day).
Toxicity tests were conducted following OECD guidelines No. 201 (OECD, 201) [Citation24]. The stock solution of Cu(II) was prepared with CuSO4 (analytic grade) purchased from Sigma-Aldrich Company (USA). Suwannee River humic acids (standard number: 3S101H) was obtained from International Humic Substances Society and dissolved in ultrapure water, followed by microfiltration (0.45 μm) to remove insoluble residues. A total of sixteen experiment groups, each with the initial cell density of 2 × 106 cells/mL, were applied with three biological replicates in this study: group 1 was the control (without addition of Cu and HA); groups 2–6 were exposed to the sole Cu with different concentrations (0.125, 0.5, 1.0, 1.5 and 2.0 mg/L, respectively); groups 7–11 were exposed to the mixture of Cu and HA (10 mg/L), and groups 12–16 to the mixture of Cu and HA (20 mg/L). During the whole experiment, the pHs of solutions varied within the range of 7.0~7.6.
2.2. Growth measurement and Chlorophyll a
The algal cell density (cells/mL) was measured with an optical microscope (Nikon, Shanghai). The density of algal cultures was counted every 24 h for 96 h. The growth rate (μ) of algal cell was calculated by Equation (1) [Citation26]:
where Nt and N0 are the cell densities at day t and day 0, respectively.
The Chlorophyll a (Chl-a) concentration was determined based on the method of acetone extraction [Citation27]. First, 15 mL algal samples were filtered via a cellulose acetate filter (0.45 μm), which were then placed in the refrigerator (−20°C) and suffered frozen-thawed cycles three times. After that, 10 mL of acetone solution (90%) was added to the centrifuge tube and maintained at 4°C for 24 h. Finally, the algal extracts were well shaken and centrifuged at 2500 g for 20 min, with the supernatant collecting for the determination of Chl-a (Ca) contents, which was calculated via the following EquationEquation (2)(2)
(2) :
where A630, A647, A664 and A750 are the absorbance values at 630, 647, 664 and 750 nm, respectively.
2.3. Determination of superoxide dismutase and malondialdehyde
The activity of superoxide dismutase and malondialdehyde was analyzed according to the nitro blue tetrazolium photo-reduction method and barbituric acid method, respectively [Citation27]. Superoxide dismutase and malondialdehyde were identified based on the enzyme solution separated from the algae, which were measured with the absorbance of 450 nm and 532 nm, respectively. First, 10 mL of algal solution was collected and centrifuged at 2500 g for 10 min to obtain algal cells. Second, the algal surface was rinsed using phosphate buffer solution (PBS). Then, 5 mL of PBS solution was added to the algal cells and the cell wall was smashed using an ultrasonic disruptor (Scientz, Ningbo, China). After sonication, the suspension was centrifuged at 5000 g for 20 min to collect the supernatant for analysis.
2.4. The fractionation procedure of EPS matrix
The EPS matrix consists of soluble EPS (SL-EPS) and bound EPS, and the latter can be divided into loosely bound EPS (LB-EPS) and tightly bound EPS (TB-EPS) due to its dynamic double-layered structure [Citation28]. To eliminate the influence of HA in spectral detection, only the bound EPS was collected via the differential centrifugation method [Citation28]. Briefly, an aliquot of 40 mL C. vulgaris sample was centrifuged at 2500 g for 15 min to remove SL-EPS, and an equal volume of 0.05% NaCl was added to the algal cells, followed by centrifugation at 5000 g for 15 min to collect the LB-EPS. Then, the residual sample was dispersed with NaCl solution, heated at 60°C for 30 min and centrifuged at 15,000 g for 20 min to collected the TB-EPS.
2.5. FIFFF analysis of EPS
Variations in the molecular weight distribution of EPS matrix was detected via using the FIFFF system (AF 2000, Postnova, German) equipped with a fluorescence detector (RF-20A, Shimadzu, Japan) with Ex/Em wavelengths of 275/340 nm to detect protein-like substances [Citation28]. The spacer height for the FIFFF system was 0.35 mm, with 0.3 kDa polyether sulfone ultrafiltration membrane. The mobile phase consists of 10 mM NaCl (aq) and 5 mM H3BO3 (aq) at pH of 8.0 [Citation29,Citation30].
2.6. Spectroscopic and physicochemical analysis of EPS matrix
Fluorescence EEM spectra were collected via an FL970-Plus spectrofluorometer by scanning the Ex wavelength at 200–450 nm and Em wavelength at 250–550 nm. The Em and Ex slits were 5 nm, and the scan rate was 2400 nm/min. Water Raman scatter peaks were eliminated by subtracting the EEM spectra of Milli-Q water blank. The PARAFAC analysis was performed using the DOMFluor toolbox (http://www.models. life.ku.dk/) in Matlab R2018a (Math Work Inc, USA).
The concentrations of Cu in EPS matrix were determined via atomic absorption spectrometer (PinAAcle 900 T, America). In addition, the coomassie brilliant blue method was applied to measure the protein concentration, and the phenol sulfuric acid method was used for the polysaccharide analysis [Citation28].
2.7. Statistical analysis
In this study, all of the experiments were performed in three biological replicates and the results were expressed as mean ± standard deviation. Differences between groups were analyzed by one-way ANOVA using IBM SPSS Statistics 22 software, with * and ** defined as significant (p < 0.05) and extremely significant (p < 0.01), respectively.
3. Results and discussion
3.1. Microalgae growth inhibition
The time-dependent growth curves of C. vulgaris under various conditions are presented in ). Overall, the biomass of C. vulgaris under each incubation increased with enhancing exposure time. The biomass of C. vulgaris for each exposure time was highest for the control but lowest with the sole presence of Cu. The results indicated that the 0.5 mg/L of Cu could significantly inhibit the growth of C. vulgaris. In the presence of HA, the algal biomass was higher than those in the sole presence of Cu, and high concentration of HA (e.g. 20 mg/L) exhibited higher improvement effects than low concentration (e.g. 10 mg/L). The results suggested that the addition of HA can alleviate the toxicity of Cu to algal biomass. This was further testified by ) that the final growth rate decreased with enhancing Cu concentrations, and presence of HA can enhance the growth rate irrespective of Cu concentrations. For example, the total growth rate increased from 11.01 ± 0.78% in the absences of HA to 24.05 ± 2.22% and 33.78 ± 4.41% in the presence of 10 mg/L and 20 mg/L of HA, respectively. It was known that Cu could bind with HA to form the HA-Cu complexation, which can change the ion species and decrease the related toxicity. The complexation experiment between Cu and HA testified that, with the increased Cu or HA, the amounts of the complexes increased correspondingly, which resulted in reduced exchangeable Cu [Citation2,Citation31]. In addition, the HA-Cu complexes were usually characterized with large molecular sizes and hard to penetrate the cell membrane, thus mitigating the toxicity of Cu to algal biomass [Citation1].
Figure 1. The time-dependent changes of the algal densities with or without HA as affected by 0.5 mg/L of Cu (a) and variations of final growth rates (b) and Chl-a (c) of C. vulgaris under various Cu and HA concentrations. * and ** mean significantly (p < 0.05) and extremely significantly (p < 0.01), respectively
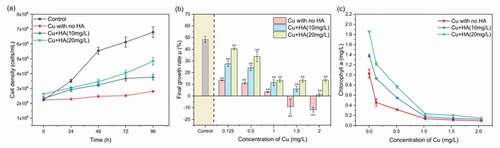
In addition, variations in concentrations of the Chl-a as affected by Cu and HA ()) were similar to those of growth rates. In the absence of HA, the final concentrations of Chl-a decreased from 1.03 ± 0.08 mg/L with no Cu addition to 0.09 ± 0.012 mg/L with 2 mg/L of Cu, showing obvious biotoxicity of Cu to C. vulgaris. The addition of HA increased the abundance of Chl-a, irrespective of Cu levels. For instance, when Cu concentration was 0.125 mg/L, the concentrations of Chl-a with 10 mg/L and 20 mg/L of HA were 1.3 and 1.7 times higher than those without HA, respectively. The results demonstrated that organic ligands in the aquatic solutions could alleviate the biotoxicity of Cu, and the alleviation efficiency was positively correlated with the abundance of organic ligands.
3.2. Changes of enzymatic activities
During normal physiological activities, the antioxidant enzyme system and the level of malondialdehyde in algal cells were retained with a dynamic balance. Superoxide dismutase was a superoxide scavenger that protected the organism from reactive oxygen species damage, while malondialdehyde represented the by-product of peroxide decomposition of unsaturated fatty acids generated from malondialdehyde which can reflect the rate and intensity of lipid peroxidation [Citation26,Citation27]. Thus, superoxide dismutase and malondialdehyde measurements can be used to assess the extent of Cu-induced cellular damage and whether the presence of HA can alleviate the cellular damage.
As shown in , both the two enzymatic activities increased with increasing Cu concentration, indicating obvious antioxidant activity in response to the stress of Cu. For example, the activities of superoxide dismutase increased from 1581.18 ± 138.65 nmol/107 cells in the control to 3244.87 ± 352.72 nmol/107 cells at 2 mg/L of Cu, and those of malondialdehyde increased from 168.20 ± 33.11 nmol/107 cells in the control to 516.51 ± 19.01 nmol/107 cells at 2 mg/L of Cu. The results were in concordance with previous studies that increased concentrations of pollutants (e.g. benzophenone-4, microplastics and nanoparticles) caused an obvious enhancement of the malondialdehyde level in microalgae [Citation26,Citation32]. Similar phenomenon can also be found for other contaminants and algal mediums, such as microplastics on Chlamydomonas reinhardtii [Citation27], nonylphenol on Chlorella pyrenoidosa [Citation33] and cadmium on Scenedesmus obliquus [Citation34], etc.
Figure 2. The concentrations of superoxide dismutase (a) and malondialdehyde (b) in C. vulgaris after 96 h of exposure as affected by different Cu and HA concentrations. * and ** mean significantly (p < 0.05) and extremely significantly (p < 0.01), respectively
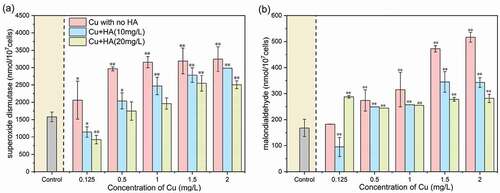
In addition, regardless of Cu levels, the activities of superoxide dismutase and malondialdehyde in the presence of HA were significantly (p < 0.05) lower than those without HA. This phenomenon definitely suggested the efficiencies of dissolved organic ligands in alleviating the oxidative stress to the algal cells [Citation10,Citation30]. Moreover, the activities of superoxide dismutase and malondialdehyde in the presence of 20 mg/L HA were significantly lower than those in the case of 10 mg/L, indicating that the toxicity mitigation of Cu was highly dependent on HA concentration.
3.3. Variations in concentrations and compositions of EPS matrix in response to Cu and HA
3.3.1. Changes of proteins and polysaccharides
EPS were reported as fine threads that framed complicated systems around the algal cell, which played a defensive role in algal metabolism [Citation3,Citation35]. Under the standard medium, the concentrations of polysaccharides were 37.97 ± 3.16 µg/107 cells, which were higher than those of proteins (9.57 ± 1.51 µg/107 cells), demonstrating that polysaccharides were the main components within the EPS matrix, as also found in other microbial cultures such as Dictyosphaerium sp. and M. aeruginosa [Citation28,Citation36]. As for the EPS fractions, the concentrations of proteins were 5.44 ± 2.19 and 13.70 ± 0.83 µg/107 cells in the LB-EPS and TB-EPS, respectively, while those of polysaccharides were 39.33 ± 1.25 and 36.62 ± 5.08 µg/107 cells, respectively. The results demonstrated that proteins were mainly located in the TB-EPS fraction, while polysaccharides were evenly distributed between the LB- and TB-EPS fractions. Previous studies reported the main components of polysaccharides in the TB-EPS fraction for sludge [Citation37], microbial biofilms [Citation38] and Microcystis aeruginosa [Citation28], etc. This difference indicated that the organic components and distribution patterns of EPS matrix were highly dependent on the microbial species applied.
In the absence of HA, contents of proteins in the two EPS fractions increased consistently with enhancing Cu concentrations. For example, the contents of proteins in the LB-EPS and TB-EPS fraction with 2.0 mg/L of Cu were 2.52 and 1.89 times higher than those with 0.125 mg/L of Cu (–b)), which suggested that more proteins were secreted due to the high abundance of Cu addition. The results were consistent with previous findings that enhanced EPS production was observed in algal cultures which were exposed to other heavy metals such as Pb and Cd [Citation3,Citation39]. Contents of polysaccharides in both LB- and TB-EPS fractions consistently increased as Cu increased from 0.125 mg/L to 1.0 mg/L, but immediately decreased with further Cu addition, especially for the LB-EPS fraction. The results indicated that excretion of polysaccharides, especially for those in the LB-EPS fraction, was more sensitive than proteins to the addition of heavy metals.
In the presence of HA, contents of proteins and polysaccharides in both two EPS fractions decreased obviously as compared to those without HA, and the reduction extents at 20 mg/L of HA were higher than those in 10 mg/L of HA. This indicated that the presence of HA could decrease the oxidation damage of algal cells which resultantly induce less excretion of the EPS matrix.
3.3.2. Variations in fluorescent components of EPS matrix revealed by EEM-PARAFAC analysis
To explore the variations in fluorescent components in the EPS matrix, fluorescent spectroscopy was applied herein, with typical EEM contours in the two EPS fractions in Fig. S1. Both the LB-EPS and TB-EPS fractions exhibited two fluorescent peaks with Ex/Em locations of 300/345 nm (peak A) and 245/340 nm (peak B). As previously reported, peak A was assigned as tryptophan-like substances, and peak B to the tyrosine-like ones[40]. The results demonstrated the predominant distribution of protein-like substances within the EPS matrix, which were in discrepancy with other cyanobacterial species such as Microcystis aeruginosa that abundant humic- and/or fulvic-like substances can be found in the EPS matrix [Citation11]. The results indicated that the distribution patterns of fluorescent components in EPS matrix were obviously dependent on the algal species.
The PARAFAC model was applied to reveal the detailed variations in fluorescent components in response to Cu and HA. As shown in , two fluorescent components, namely, component 1 [C1, Ex/Em of (245, 300)/345 nm] and component 2 [C2, Ex/Em of 310/350 nm] that were assigned to tryptophan- and tyrosine-like components, respectively, can be obtained [Citation40, Citation41]. In the absence of HA, the intensities of all fluorescent components increased with enhancing Cu concentration, demonstrating more secretion of the EPS matrix. The result was consistent to previous work that the microalgae can produce more proteins under the stimulation of Cd2+ [Citation3]. However, the fluorescence values of both C1 and C2 decreased significantly in the presence of HA, further testifying the contribution of HA in alleviating the toxicity damage of heavy metals to algae.
Figure 3. The Cu concentration-dependent changes of proteins and polysaccharides in the EPS matrix in the absence and presence of HA after 96 h of exposure. (a) proteins in the LB-EPS fraction; (b) proteins in the TB-EPS fraction; (c) polysaccharides in LB-EPS fraction; and (d) polysaccharides in TB-EPS fraction. * and ** mean significantly (p < 0.05) and extremely significantly (p < 0.01), respectively
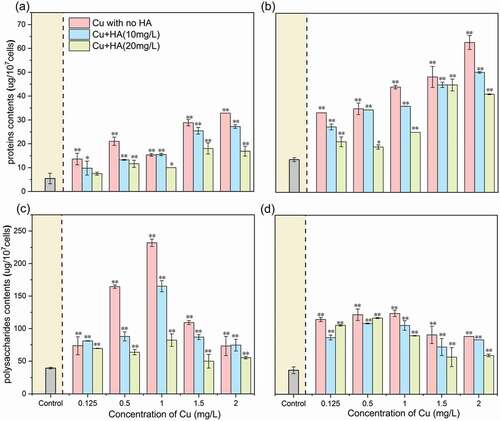
3.4. Changes of molecular weight distribution of EPS matrix as affected by Cu and HA
Although the physicochemical and fluorescent analysis can explore the Cu- and HA-induced variations of organic components within EPS matrix, detailed information on the changes of molecular weight distribution cannot be revealed. Here, the FIFFF was applied to explore the heterogeneities in molecular sizes of EPS matrix under different treatments after 96 h of exposure. As shown in –b), for all treatments, the protein-like fractograms in the LB- and TB-EPS fractions were both characterized with two obvious fluorescent bands locating at 0.3~15 kDa and 50 kDa~0.45 μm, indicating the wide molecular weight distribution range of EPS matrix. In the absence of HA, the fractogram intensities of each band, especially for the large-molecular-sized band (i.e. 100 kDa-0.45um), were significantly higher than the control, demonstrating more EPS secretion in the sole presence of Cu. Addition of HA, however, can significantly decreased the fractogram intensities, and the decline extent at 20 mg/L of HA was greater than those at 10 mg/L of HA. These results were consistent with those of the physicochemical and PARAFAC analysis that presence of HA can alleviate the bio-toxicity of heavy metals to C. vulgaris.
Figure 5. Variations in protein-like FIFFF fractograms and molecular weight distribution in response to Cu and HA. It was noted that (a) and (b) were the FIFFF fractograms for LB-EPS and TB-EPS fractions and (c) and (d) were the molecular weight distribution of protein-like substances for LB-EPS and TB-EPS fractions, respectively
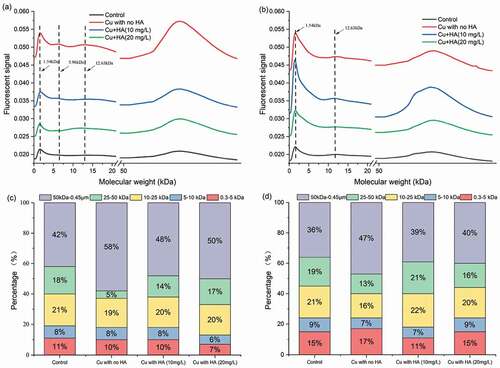
To obtain the quantitative information on the variations in molecular weight of the EPS matrix, the original fractograms were integrated into several fractions and presented in terms of relative percentage. As shown in and d), the main molecular weight range for the two EPS fractions were 50 kDa~0.45 μm (36–58%), followed by 10~25 kDa (16–22%), 25~50 kDa (5–19%), 0.3~5 kDa (7–17%) and 5~10 kDa (6–9%). It was noted that the organic ligands within 0.3~5, 5~10 and 10~25 kDa fractions exhibited somewhat fluctuations and no obvious changing patterns can be observed among different treatments. However, the percentage of organic ligands in the large-molecular-size fraction, i.e. 50 kDa~0.45 μm, exhibited obvious variations in response to Cu and HA. Specifically, the percentage of 50 kDa~0.45 μm in the LB-EPS and TB-EPS fractions increased from 42% and 36% in the control to 58% and 47% in the presence of Cu, respectively. The results indicated that C. vulgaris was more inclined to secrete high molecular weight protein-like substances under the exposure of Cu stress. Similarly, previous studies found that marine diatom Amphora sp. secreted acidic extracellular polysaccharides with molecular weight of 1000 kDa in response to enhancing Th (IV) concentrations [Citation42]. In the presence of HA, the percentage of 50 kDa~0.45 μm fraction decreased from 58% and 47% to 48–50% and to 39–40% for the LB-EPS and TB-EPS fractions, respectively. The physicochemical and fluorescent analysis demonstrated the HA-induced biotoxicity alleviation via more EPS secretion, application of FlFFF further revealed that 50 kDa~0.45 μm of protein-like substances were the critical fractions responsible for the biotoxicity alleviation.
3.5. Distribution of Cu within EPS matrix as affected by Cu and HA
To determine the acquisition potential of Cu by EPS matrix, the time-dependent changes of distribution of Cu in the two EPS fractions are shown in . In the absence of HA, the contents of Cu in both LB- and TB-EPS fractions increased with increasing exposure time, suggesting obvious acquisition capacities of EPS matrix for Cu. After 96 h of exposure, the Cu contents in the LB-EPS and TB-EPS fractions reached 0.012 ± 0.00049 mg/108 cells and 0.029 ± 0.00148 mg/108 cells, respectively. The results indicated that the TB-EPS fraction was characterized with higher Cu acquisition potential than the LB-EPS fraction. Previous studies also found that the TB-EPS fraction extracted from algal aggregates showed higher complexation capacities to metal oxide nanoparticles than the LB-EPS fraction [Citation12,Citation13]. In contrast, Lu et al. (2021) reported that the LB-EPS fraction secreted from bacterial cells exhibited stronger acquisition potential to heavy metals than the TB-EPS fraction. The heterogeneities would be attributed to the different organism species used and the resultant EPS compositions (Xie et al. 2020; Cheng et al. 2020). Presence of HA, however, caused an obvious decline of Cu content in both the LB-EPS and TB-EPS fractions, and the decline extent with 20 mg/L of HA addition was greater than those of 10 mg/L of HA. Therefore, combined with the physicochemical and fluorescent results (), it was found that not only the secretion amounts but also the acquisition capacities of EPS matrix decreased due to the presence of HA.
4. Conclusion
The contrasting effects of Cu and HA on the growth inhibition, oxidative stress, and extracellular secretion were explored. The contents of algal biomass decreased while those of enzymes increased in the sole presence of Cu; however, addition of HA can significantly alleviate the Cu-induced toxicity damage. The physicochemical and fluorescent analysis demonstrated more production of proteins and polysaccharides in the sole presence of Cu but decreased secretion due to the addition of HA. Application of FlFFF revealed that 50 kDa~0.45 μm of protein-like substances were the critical fractions responsible for the biotoxicity alleviation and oxidative stress. Furthermore, it was found that not only the secretion amounts but also the acquisition capacities of EPS matrix decreased due to the presence of HA. These findings can enhance our understandings towards the toxicity of heavy metals to aquatic microorganisms in some natural waters that contained abundant organic ligands.
Supplemental Material
Download MS Word (140.8 KB)Disclosure statement
No potential conflict of interest was reported by the author(s).
Supplementary material
Supplemental data for this article can be accessed here.
Additional information
Funding
References
- Bai HC, Wei SQ, Jiang ZM, et al. Pb (II) bioavailability to algae (Chlorella pyrenoidosa) in relation to its complexation with humic acids of different molecular weight. Ecotox Environ Safe. 2019;167:1–9.
- Sanchez-Marin P, Santos-Echeandia J, Nieto-Cid M, et al. Effect of dissolved organic matter (DOM) of contrasting origins on Cu and Pb speciation and toxicity to Paracentrotus lividus larvae. Aquat Toxicol. 2010;96(2):90–102.
- Xie Q, Liu N, Lin DH, et al. The complexation with proteins in extracellular polymeric substances alleviates the toxicity of Cd (II) to Chlorella vulgaris. Environ Pollut. 2020;263. DOI:https://doi.org/10.1016/j.envpol.2020.114102.
- Yi Y, Yang Z, Zhang S. Ecological risk assessment of heavy metals in sediment and human health risk assessment of heavy metals in fishes in the middle and lower reaches of the Yangtze River basin. Environ Pollut. 2011;159(10):2575–2585.
- Adams MS, Dillon CT, Vogt S, et al. Copper uptake, intracellular localization, and speciation in marine microalgae measured by synchrotron radiation X-ray fluorescence and absorption microspectroscopy. Environ Sci Technol. 2016;50(16):8827–8839.
- Chen K, Huang L, Yan B, et al. Effect of lead pollution control on environmental and childhood blood lead level in Nantong, China: an interventional study. Environ Sci Technol. 2014;48(21):12930–12936.
- Verschoor AJ, Vink JP, De Snoo GR, et al. Spatial and temporal variation of watertype-specific no-effect concentrations and risks of Cu, Ni, and Zn. Environ Sci Technol. 2011;45(14):6049–6056.
- Jedruch A, Beldowska M, Kwasigroch U. Forms of mercury in the baltic mussel (Mytilus trossulus): human and ecosystem health risk assessment. Environ Res. 2019;179:108755.
- Herzi F, Jean N, Zhao H, et al. Copper and cadmium effects on growth and extracellular exudation of the marine toxic dinoflagellate Alexandrium catenella: 3D-fluorescence spectroscopy approach. Chemosphere. 2013;93(6):1230–1239.
- Shi W, Jin ZF, Hu SY, et al. Dissolved organic matter affects the bioaccumulation of copper and lead in Chlorella pyrenoidosa: a case of long-term exposure. Chemosphere. 2017;174:447–455.
- Wang C, Wang X, Wang PF, et al. Effects of iron on growth, antioxidant enzyme activity, bound extracellular polymeric substances and microcystin production of Microcystis aeruginosa FACHB-905. Ecotoxicol Environ Saf. 2016;132:231–239.
- Xu HC, Pan JZ, Zhang H, et al. Interactions of metal oxide nanoparticles with extracellular polymeric substances (EPS) of algal aggregates in an eutrophic ecosystem. Ecol Eng. 2016;94:464–470.
- Zhao JF, Liu SX, Liu N, et al. Accelerated productions and physicochemical characterizations of different extracellular polymeric substances from Chlorella vulgaris with nano-ZnO. Sci Total Environ. 2019;658:582–589.
- Lamelas C, Pinheiro JP, Slaveykova VI. Effect of humic acid on Cd(II), Cu(II), and Pb(II) uptake by freshwater algae: kinetic and cell wall speciation considerations. Environ Sci Technol. 2009;43(3):730–735.
- Sayadi MH, Pavlaki MD, Martins R, et al. Bioaccumulation and toxicokinetics of zinc oxide nanoparticles (ZnO NPs) co-exposed with graphene nanosheets (GNs) in the blackfish (Capoeta fusca). Chemosphere. 2021;269:128689.
- Nogueira PF, Melao MG, Lombardi AT, et al. Natural DOM affects copper speciation and bioavailability to bacteria and ciliate. Arch Environ Contam Toxicol. 2009;57(2):274–281.
- Mansouri B, Maleki A, Davari B, et al. Histopathological effects following short-term coexposure of Cyprinus carpio to nanoparticles of TiO2 and CuO. Environ Monit Assess. 2016;188(10):575.
- Mansouri B, Maleki A, Johari SA, et al. Copper bioaccumulation and depuration in common carp (Cyprinus carpio) following co-exposure to TiO2 and CuO nanoparticles. Arch Environ Contam Toxicol. 2016;71:541–552.
- Wang ZY, Li J, Zhao J, et al. Toxicity and internalization of CuO nanoparticles to prokaryotic alga Microcystis aeruginosa as affected by dissolved organic matter. Environ Sci Technol. 2011;45(14):6032–6040.
- Lamelas C, Slaveykova VI. Pb uptake by the freshwater alga Chlorella kesslerii in the presence of dissolved organic matter of variable composition. Environ Chem. 2008;5(5):366–372.
- Wang J, Liu G, Liu H, et al. Multivariate statistical evaluation of dissolved trace elements and a water quality assessment in the middle reaches of Huaihe River, Anhui, China. Sci Total Environ. 2017;583:421–431.
- Atibu EK, Devarajan N, Thevenon F, et al. Concentration of metals in surface water and sediment of Luilu and Musonoie Rivers, Kolwezi-Katanga, democratic Republic Of Congo. Appl Geochem. 2013;39:26–32.
- Prado AG, Torres JD, Martins PC, et al. Studies on copper(II)- and zinc(II)-mixed ligand complexes of humic acid. J Hazard Mater. 2006;136(3):585–588.
- Kovacik J, Bujdos M, Babula P. Impact of humic acid on the accumulation of metals by microalgae. Environ Sci Pollut Res Int. 2018;25(11):10792–10798.
- Development O. OECD guidelines for testing of chemicals. Section. 2001;4.
- Zhang MC, Steinman AD, Xue QJ, et al. Effects of erythromycin and sulfamethoxazole on Microcystis aeruginosa: cytotoxic endpoints, production and release of microcystin-LR. J Hazard Mater. 2020;399:123021.
- Wang Q, Wangjin X, Zhang Y, et al. The toxicity of virgin and UV-aged PVC microplastics on the growth of freshwater algae Chlamydomonas reinhardtii. Sci Total Environ. 2020;749:141603.
- Xu HC, Cai HY, Yu GH, et al. Insights into extracellular polymeric substances of cyanobacterium Microcystis aeruginosa using fractionation procedure and parallel factor analysis. Water Res. 2013;47(6):2005–2014.
- Fu XW, Du HY, Xu HC. Comparison in UV-induced photodegradation properties of dissolved organic matters with different origins. Chemosphere. 2021;280:130633.
- Pan JZ, Fu XW, Wang CH, et al. Adsorption and molecular weight fractionation of dissolved organic matters with different origins on colloidal surface. Chemosphere. 2020;261:127774.
- Sánchez-Marín P, Slaveykova VI, Cu BR. Pb accumulation by the marine diatom Thalassiosira weissflogii in the presence of humic acids. Environ Chem. 2010;7:3.
- Huang Y, Luo L, Ma XY, et al. Effect of elevated benzophenone-4 (BP4) concentration on Chlorella vulgaris growth and cellular metabolisms. Environ Sci Pollut Res Int. 2018;25(32):32549–32561.
- Yang W, Gao X, Wu Y, et al. The combined toxicity influence of microplastics and nonylphenol on microalgae Chlorella pyrenoidosa. Ecotoxicol Environ Saf. 2020;195:110484.
- Zhang Y, Yang RX, Wang SY, et al. Influence of humic substances on the toxic effects of cadmium and SDBS to the green alga Scenedesmus obliquus. Environ Toxicol Pharmacol. 2019;68:94–100.
- Zhang JY, Zhou F, Liu YX, et al. Effect of extracellular polymeric substances on arsenic accumulation in Chlorella pyrenoidosa. Sci Total Environ. 2020;704:13.
- Cheng Q, Jiang Y, Jin Z, et al. Enhanced excretion of extracellular polymeric substances associated with nonylphenol tolerance in Dictyosphaerium sp. J Hazard Mater. 2020;395:122644.
- Sheng GP, Yu HQ. Characterization of extracellular polymeric substances of aerobic and anaerobic sludge using three-dimensional excitation and emission matrix fluorescence spectroscopy. Water Res. 2006;40(6):1233–1239.
- Miao L, Wang C, Hou J, et al. Response of wastewater biofilm to CuO nanoparticle exposure in terms of extracellular polymeric substances and microbial community structure. Sci Total Environ. 2017;579:588–597.
- Li C, Zheng C, Fu H, et al. Contrasting detoxification mechanisms of Chlamydomonas reinhardtii under Cd and Pb stress. Chemosphere. 2021;274:129771.
- Xu HC, Li FF, Kong M, et al. Adsorption of cyanobacterial extracellular polymeric substance on colloidal particle: influence of molecular weight. Sci Total Environ. 2020;715:136959.
- Xu HC, Yan ZS, Cai HY, et al. Heterogeneity in metal binding by individual fluorescent components in a eutrophic algae-rich lake. Ecotox Environ Safe. 2013;98:266–272.
- Zhang S, Xu C, Santschi PH. Chemical composition and 234Th (IV) binding of extracellular polymeric substances (EPS) produced by the marine diatom Amphora sp. Mar Chem. 2008;112(1–2):81–92.