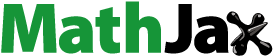
ABSTRACT
Cyanobacterial outbreaks seriously affect drinking water safety. Therefore, developing effective algal removal technologies is urgently needed. The study aims to investigate the effects of pH, electrode distance, current density, and initial algal concentration on electrochemical algae collection. Consequently, we loaded nanoscale zero-valent iron (nZVI) on activated carbon fiber/nickel foam (ACF/Ni) to form an ACF/nZVI/Ni composite cathode using the liquid phase reduction method and used titanium-based platinum plating (Pt/Ti) as the anode to construct a novel Pt/Ti- ACF/nZVI/Ni electrochemical system. The results showed that on pH of 6.0, the electrode distance was 10 mm, the current density was 75 mA/cm2, and the initial algal concentration was OD680 = 0.100; the algal cell removal rate was 94.20% after electrolysis for 30 min. This paper provides an efficient and environmentally friendly electrochemical algal removal technology for protecting the safety of drinking water and dealing with cyanobacterial outbreaks.
1. Introduction
Global warming and year-on-year increases in greenhouse gas emissions such as carbon dioxide (CO2) have expanded the cyanobacterial bloom outbreaks [Citation1]. This threatens marine life worldwide and human being survival and development [Citation2]. This situation has raised public awareness of the occurrence of algal blooms and there is an urgent need to develop effective techniques to control their growth [Citation3]. As an effective and environmentally friendly method, electrochemical technology has attracted much attention in the treatment of polluted water bodies [Citation4].
Electro-Fenton (EF), a highly utilized electrochemical technology, can robustly degrade various organic compounds, including toxic and non-biodegradable compounds [Citation5,Citation6]. Particularly, the non-homogeneous-EF technique provides hydrogen peroxide (H2O2) through cathodic reduction of two-electron molecular oxygen (EquationEquation 1(1)
(1) ) [Citation7], which reacts with a nanoscale zero-valent iron (nZVI) catalyst to produce •OH to remove the pollutants and cycle Fe3+ and Fe2+ on the catalyst surface (EquationEquations 2
(2)
(2) –Equation4
(4)
(4) ) [Citation8,Citation9]. Nanoscale zero-valent iron has a large specific surface area, strong reducibility, simple synthesis and other characteristics, has a wide range of applications in groundwater pollution treatment [Citation10–12].
According to the study, electro-Fenton is an efficient process for cyanobacteria removal using carbon cathode under acidic conditions [Citation13]. However, the narrow working pH range and the large consumption of exogenous chemicals limit the widespread use of the electro-Fenton process [Citation14]. Few studies have been conducted on algal removal by EF technology, and most of the studied EF systems were based on acidic conditions and involved adding Fe2+ [Citation15]. In certain cases, a sheet-iron anode was used, leading to anode corrosion and air passage to support the cathode for producing H2O2 [Citation15,Citation16]. Carbon-based materials such as the activated carbon fiber (ACF) electrode have high hydrogen precipitation overpotentials and can autonomously produce H2O2 [Citation17]. Accordingly, they are widely used in non-homogeneous-EF systems [Citation18,Citation19]. ACF, an activated carbon fiber (CF), is a cathode material used to treat recalcitrant organic pollutants and has enhanced adsorption capacity and extensive applications in domestic and industrial wastewater treatments [Citation20].
The carbon-based material ACF – non-toxic, chemically stable, and corrosion-resistant – was adhered to nickel foam (Ni) – inexpensive, conductive, strong, and enhances current efficiency – as a metallic skeleton with a conductive substrate as a binder to form an ACF/Ni cathode capable of generating large quantities of H2O2 without air intake [Citation21]. This ACF/Ni cathode addresses ACF mat limitations that cannot be used as electrodes independently and meet the non-homogeneous-EF requirements. The conventional electro-Fenton system suffers from high ferrous ion loss and iron containing sludge [Citation8]. In this study, although there was also iron ion leaching, the amount of iron ions in the solution was less compared to the electro-Fenton system with direct Fe2+ injection [Citation21].
In this paper, the composite cathode of ACF/nZVI/Ni was prepared by loading nZVI on ACF/Ni, and titanium-based platinum plating (Pt/Ti) was used as the anode to investigate the effects of initial pH, electrode distance, current density, and initial algal concentration on the electrochemical algae removal, and the efficacy of the electrochemical system of Pt/Ti-ACF/nZVI/Ni for the removal of algae in the absence of oxygen supply condition was explored. Based on the indirect detection of ⋅OH and ⋅O2−, infrared spectral analysis of ACF/nZVI and algal cells, scanning electron microscopy analysis and three-dimensional fluorescence analysis of algal cells, the algal removal mechanism of the Pt/Ti-ACF/nZVI/Ni electrochemical system was investigated. In this study, an efficient algal removal system was established, which breaks through the limitations of the narrow working pH range and high consumption of exogenous chemicals of the electro-Fenton method. This study provides an innovative approach for the treatment of highly concentrated algal water.
2. Materials and methods
2.1. Materials and reagents
Microcystis aeruginosa (FACHB-913) was acquired from the Institute of Aquatic Biology, Chinese Academy of Sciences. Under aseptic conditions, the algal solution was mixed with BG-11 medium and placed in a constant temperature light incubator: 3000 Lux light intensity, 26 ± 1°C, 12:12 light–dark ratio. The algal species was subjected to several shakes throughout the day at regular intervals to ensure a homogeneous mixture of the cells. ACFs were purchased from Sutong Carbon Fiber Ltd (Jiangsu, China); Ni from Kunshan Ansu Electronic Materials (China); Pt/Ti electrodes from Jiangsu Yiwanlin Co. (China); Anhydrous ethanol from Chengdu Cologne Chemical Reagent Co. (China); Sodium borohydride (NaBH4) from Cormio Chemical Reagent Co., Ltd. (Tianjin, China); N-Methylpyrrolidone from Aladdin Biochemical Technology (Shanghai, China); Conductive Carbon Black from Fuzhou Yihuan Carbon (China); and the other chemicals from Sinopharm Chemical Reagent Company Limited (China).
2.2. Experimental method and setup
The ACFs were cut into 3 × 4 cm pieces, boiled and soaked in deionized water for 3 and 12 h, respectively, to eliminate water-soluble and volatile, and vacuum-dried at 105°C for 12 h. The Ni was cut into 4 × 4 cm squares, placed in anhydrous ethanol, soaked in ultrasound, and 3 mol/L hydrochloric acid for 30 min each. Subsequently, Ni was washed with hydrochloric acid by ultrasound with pure water and anhydrous ethanol for 10–15 min and vacuum-dried at 60°C for 12 h.
The nZVI was synthesized by the liquid-phase reduction method. ACF/nZVI composites were prepared: 100 mL of aqueous ethanol (ethanol: deionized water = 3:7) was added to a three-necked flask and 0.18 mol/L of FeSO4-7 H2O was dissolved. Then, 0.3 g of ACF was added and stirred for 30 min to form a complex of ACF and iron. Subsequently, the freshly prepared NaBH4 solution (2.04 g NaBH4 in 100 mL) was added drop by drop to the stirred mixture at a rate of 1–2 drops/s until the black substance formed. After the dropwise addition of the NaBH4 solution, nitrogen was continued to pass and stirred for 30 min until H2 production ceased. After the reaction, the composites in the flask were washed sequentially with ultrapure water and anhydrous ethanol, dried in a vacuum drying oven, allowed to cool, rinsed with nitrogen, and set aside labeled ACF/nZVI. Finally, the ACF/nZVI composite was bonded to Ni with a formulated adhesive and vacuum-dried at 60°C for 1 h. In the adhesive formulation, conductive carbon black and polyvinylidene fluoride (PVDF) were used in a 1:1 ratio, followed by adding an appropriate amount of N-methylpyrrolidone and stirring for 2 h.
shows the schematic diagram of the ACF/nZVI synthesis device; it mainly consists of a nitrogen bottle, cantilever stirrer, constant pressure dropping funnel, and three-mouth flask. displays the schematic diagram of the electrochemical experimental setup. The system consists of a DC power supply (DP3020), electrolyzer (250 mL beaker, effective volume 200 mL), electrodes, magnetic stirrer, and so on; the anode was Pt/Ti, and the cathode was ACF/nZVI/Ni. The effective working area of the electrodes was 12 cm2 for both electrodes, and the electrolysis time was 30 min. A 20 mM of Na2SO4 was used as an electrolyte in a 250 mL glass beaker. The experimental algal solution was stirred using a magnetic stirrer at a constant speed to mix the algal solution during electrolysis.
2.3. Analysis
A scanning electron microscope (SEM) was utilized to analyze the algal cell morphology and electrode materials (SU8020, Hitachi, Japan). The material crystalline phase was analyzed by X-ray diffraction (XRD; D8 ADVANCE, Bruker, Germany). Fourier transform infrared spectroscopy (FTIR) data were measured on an FTIR spectrometer (Nicolet IS10, Thermo, Fisher, U.S.A.) using the potassium bromide press method. Fluorescence tests of algal cells were analyzed by steady-state transient fluorescence spectroscopy (FLS1000/FSS, Edinburgh, UK). The chlorophyll content in algal cells was determined by acetone extraction [Citation22]. Superoxide radicals were determined by the nitro tetrazolium blue chloride (NBT) reduction method [Citation23]. Optical density was determined through the UV spectrophotometric method using a UV spectrophotometer (DR6000, Hach, U.S.A.).
3. Results and discussion
3.1. Microcystis aeruginosa removal
3.1.1. Effect of initial pH on the algae removal effectiveness
The algal removal rate was negatively correlated to the solution pH; the pH range was wide . At a pH of 5–7, the algal removal rate was similar and could reach more than 90%; at a pH of 5, the reaction proceeded fastest in the first 10 min, which may be related to Fe2+ solubilization under acidic conditions and, thus, the homogeneous-EF reaction [Citation24]; at a pH of 8–9, the algal removal rate decreased to 84.75% and 82.68%, respectively, which may be related to the decreased Fe2+ in the solution, which aligins with Li et al. [Citation25]. In electrochemical reactions, when a carbon material is used for the cathode, it helps O2 to react at the cathode to form H2O2. Under acidic conditions, H2O2 undergoes an electro-Fenton reaction with Fe2+ to produce •OH, which degrades organic pollutants. The highest algal removal efficiency was 95.94% at pH of 5. Thus, an acidic initial pH helps to increase the algal removal rate of this system, which mainly undergoes an electro-Fenton reaction. When the pH increases, the •OH produced by the reaction may be consumed by the OH− in the solution, thus reducing the removal of organic matter, the reaction equation is shown in EquationEquation (5)(5)
(5) [Citation21]. The system has a wide pH range, and the high algal removal rate can be obtained under neutral conditions, allowing its usage to remove algal cells from natural source water since the natural source water pH is closer to neutral.
Figure 2. (a) Effect of pH, (b) Electrode distance, (c) Current density, and (d) Initial algal concentration on the algal removal rate of Microcystis aeruginosa in Pt/Ti- ACF/nZVI/Ni electrochemical system. Electrolyte: 20 mM Na2SO4; volume of reaction solution: 200 mL; Current density: 80 mA cm−2 (a.B), 70 mA cm−2 (d); initial pH 6.0 (b.C.d); electrode distance 10 mm (a.C.d); initial algal concentration: OD680 = 0.070 (a.B.c); Electrolysis time: 30 min (a. b.C.d).
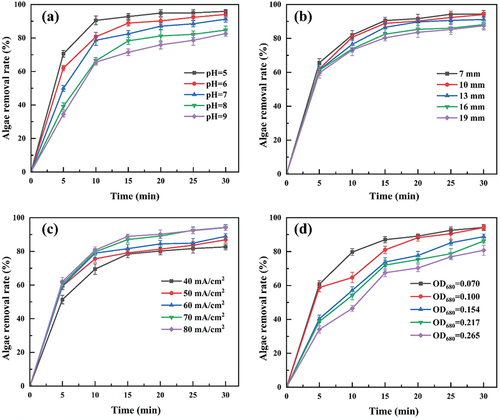
Therefore, an initial pH of 6 is recommended, which requires no adjustment to the natural water pH in the EF systems. Further studies are required to investigate the algal removal mechanism of the Pt/Ti-ACF/nZVI/Ni electrochemical system under neutral conditions.
3.1.2. Effect of electrode distance on the algae removal effectiveness
In the range of 7 mm ~19 mm, the algal removal rate decreased with the increase of electrode spacing and showed a trend that the smaller the electrode distance, the higher the algal removal rate . This is because a smaller distance between the anode and cathode promotes the active intermediate accumulation, which facilitates the algae removal. Conversely, increasing the distance between electrodes results in a higher resistance, which increases energy consumption and decreases electrolysis efficiency. Furthermore, excessively narrow electrode distances can cause inadequate solution mixing. The electrode distance was comprehensively determined to be 10 mm.
3.1.3. Effect of current density on the algae removal effectiveness
The algae removal rate gradually increased with the current density increase, with little difference in the algae removal rate after the current density exceded 70 mA/cm2 , consistent with Hou B et al. [Citation26]. The current density affects H2O2 generation in the EF system; therefore, when the current density increases, the H2O2 generation increases that could generate more •OH [Citation6,Citation27]. H2O2 generation is accompanied by oxidation reactions through the anode and its decomposition (EquationEquation 6(6)
(6) , Equation7
(7)
(7) ). When the current density is too large, the H2O2 decomposition rate with the increase in concentration and accelerates at the same time by the anodic oxidation of the reaction rate is also accelerated, the EF system of the active material instead of reducing, in addition to when the current generates energy higher than the energy required to generate H2O2, O2 can directly get the electrons to generate H2O (EquationEquation 8
(8)
(8) ). This elucidates the tendency of the algal removal rate to stabilize when our current density was higher than 70 mA/cm2 that can reach 94.26% in 30 min.
In addition, excessive current density reduces current efficiency, increases heat production at the electrodes, raises the solution temperature, and increases energy consumption. Therefore, a current density of 70 mA/cm2 was selected for the subsequent experiments on initial algal concentration.
3.1.4. Effect of initial algae concentration on the algae removal effectiveness
When the concentration of algal cells was OD680 = 0.070 and 0.100, electrolysis was performed for 30 min, and the algal removal rate was 94.26% and 94.18%, respectively, . With the increase of algal cell concentration, the alga removal rate gradually decreased, and when the algal cell concentration was OD680 = 0.265, the alga removal rate could only reach 80.75% in 30 min. However, the alga removal rate still showed an increasing trend, which aligns with Wang [Citation28]. This is because the treatment load of the electrochemical system increases as the algal cell concentration increases, and •OH generated by the electrochemical system at lower algal cell concentrations was sufficient to remove the algal cells. Consequently, the difference in the algal removal effect is insignificant. When the algal cell concentration increases significantly, electrolysis performed to produce active substances becomes insufficient to eliminate the cells instantly; it will reduce the de-algae rate. The increase in electrolysis time continues to produce •OH and gradually decreases algal cells; the de-algae rate will still be an upward trend. Therefore, an initial algal concentration of OD680 = 0.100 was used in subsequent experiments.
3.2. Analysis of the response surface analysis method results
3.2.1. The centralized combined experimental design (Box-Behnken Design; BDD) tests
A three-factor, three-level test of pH (5–7), electrode distance (7–13 mm), and current density (60–80 mA/cm2) with BDD design () yielded 17 sets of results, with a maximum algal removal rate of 95.94% and a minimum value of 88.17% ().
Table 1. Response surface factor level table.
Table 2. Response surface experimental design results.
3.2.2. Quadratic regression fitting and analysis of variance (ANOVA)
The algae removal rate was used as the response value, and regression analysis was performed using Design-Expert 10 software to obtain the quadratic regression model equation as:
Algae removal rate (%) = 94.86–1.01A–0.86B + 2.32C + 0.93AB–1.08AC–0.79BC–2.15A2 − 2.89B2 − 1.67C2
The regression model analyzed the parameters of the response surface, and the model was highly significant (p < 0.0001), and the p = 0.3415 for the misfit term indicated that the misfit was insignificant. The primary terms A (p = 0.0009), B (p = 0.0022), and C (p < 0.0001) had a highly significant effect on the results (p < 0.01); the interaction terms AB (p = 0.0086) and AC (p = 0.0042) were highly significant (p < 0.01), and BC (p = 0.0184) were significant (p < 0.05) on the results. The secondary terms A2 (p < 0.0001), B2 (p < 0.0001), and C2 (p = 0.0003) were highly significant (p < 0.01) (). The R2 = 0.9870 revealed that this model explains 98.70% of the variation in response values, the correction coefficient was 0.9702, the prediction coefficient was 0.8798, and the difference between the predicted values and the corrected values was less than 0.2, indicating a good model fit ().
Table 3. Analysis of variance for the regression model.
Table 4. Analysis of model R2..
3.2.3. Interactions between factors
Different response surfaces and contour plots indicate different interactions between the two factors, and the downward opening of the response surface indicates a stable maximum value of the response value (); the elliptical shape of the contour line indicates that the interaction between the factors significantly affects the response value [Citation29]. reflects the effect of pH and electrode distance on the algal removal rate. The three-dimensional response surface of algal removal rate gradually increased as the initial pH decreased from 7 to 6. When the initial pH is less than 6, the 3D response surface changes are smooth. The electrochemical system was shown to have better algal removal in an acidic environment than in an alkaline environment. The three-dimensional response surface of algal removal rate gradually increased as the electrode distance was decreased from 13 mm to 10 mm. When the electrode distance is less than 10 mm, the 3D response surface changes are smooth. In addition, the degree of change in the three-dimensional response surface of initial pH was greater than that of electrode distance, indicating that the effect of initial pH on algal removal was greater than that of electrode distance [Citation30].
Figure 3. Response curves and contour plots of the effect of factor interactions on algal removal rate: (a–b) Response surface and contour plots of the interaction effect of pH and electrode distance on algal removal rate, respectively; (c–d) Response surface plots versus contour plots of the interaction effect of pH and current density on algal removal rate, respectively; (e–f) Response surface and contour plots of the interaction effects of electrode distance and current density on algal removal rate, respectively.
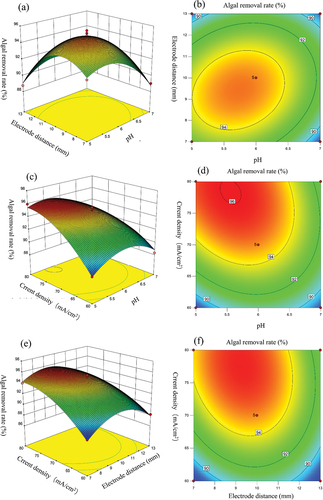
reflects the effect of pH and current density on the algal removal rate. The three-dimensional response surface of algal removal rate gradually decreased when the current density was reduced from 80 mA/cm2 to 60 mA/cm2. The increase in current density helps in algae removal. The three-dimensional response surface of algal removal rate gradually increased as the initial pH decreased from 7 to 6, and there was a slight decrease in the three-dimensional response surface of algal removal rate when the initial pH was less than 6. The change degree of current density on the three-dimensional response surface is more obvious than that of initial pH, indicating that the change degree of current density on the three-dimensional response surface is greater than that of initial pH [Citation30].
reflects the effect of electrode distance and current density on algal removal rate. The three-dimensional response surface of algal removal rate gradually decreased when the current density was reduced from 80 mA/cm2 to 60 mA/cm2. The results showed that the three-dimensional response surface of algal removal rate gradually increased as the electrode distance decreased from 13 mm to 10 mm, and there was a slight decrease in the three-dimensional response surface of algal removal rate when the electrode distance was less than 10 mm. In addition, the degree of change in the three-dimensional response surface of current density was greater than that of electrode distance, indicating that the effect of current density on algal removal was greater than that of electrode distance [Citation30].
3.2.4. Response surface methodology optimization validation
Under the prediction results of this BDD software, the theoretical optimal conditions obtained were pH of 5.863, electrode distance of 9.048 mm, current density of 76.850 mA/cm2, and the maximum algal removal rate predicted by this model was 96.057%. To examine the accuracy of the model prediction, the feasibility and economy of the treatment of water body eutrophication were comprehensively considered to determine the best model: pH of 6, electrode distance of 10 mm, current density of 75 mA/cm2, three parallel experiments under this condition, the algal cell algal removal rate of 94.20%, and the prediction of the error of ±1.857%, there is no significant difference, which can be seen in the model better to predict the experimental results. The subsequent experiments were conducted based on a pH of 6, electrode distance of 10 mm, and current density of 75 mA/cm2.
Electrical energy consumption is an important factor in evaluating the cost-effectiveness of electric restoration [Citation31]. The energy consumption can be estimated from the equation p = UIt/V, where P stands for the energy consumption (kWh/m3), I stands for the current (A), U stands for the voltage (V), t stands for the time (h), and V stands for the volume of the reactor (L) [Citation32]. In this study, under optimal conditions, the current density was 75 mA/cm2 and the removal of algal cells reached 94.20% after 30 min of electrolysis with a required energy consumption of 15.98 kWh/m3. In the future we need further research and development to reduce the cost of electrochemical treatment for wider application in water and wastewater treatment.
3.3. Characterization of ACF/nZVI/Ni cathodes and algal cells
3.3.1. Characterization of ACF/nZVI/Ni cathodes
The XRD patterns of ACF/nZVI before and after electrolysis () showed a distinct diffraction peak at 44.262° before electrolysis , corresponding to the body-centered cubic α-Fe, indicating that nZVI was successfully loaded onto ACF, which is in agreement with other related studies [Citation12]. The diffraction peaks show some broadening, which may be the result of grain refinement. The ACF can effectively improve the dispersion of nZVI particles and reduce agglomeration. In the XRD pattern one insignificant diffraction peak was also detected at 31.374°, corresponding to iron tetraoxide, indicating the presence of oxidation products produced under atmospheric conditions [Citation33]. After electrolysis a diffraction peak appears at 26.878°Corresponding to iron trioxide. The two diffraction peaks appeared at 36.139° and 60.670°, corresponding to iron tetraoxide [Citation34]. The results showed that the peak of nZVI disappeared after electrolysis, indicating that nZVI was oxidized to iron oxides.
SEM was used to observe the morphology of the electrode materials, demonstrating that the surface of the pristine ACFs was smooth and fibrous, with grooves of varying depths and shades of longitudinal texture . After loading the pristine ACFs with nZVI, the nZVI was uniformly dispersed on the surface of the ACFs . The Ni showed a clear three-dimensional reticular skeleton structure and a smooth surface with good internal connectivity . By attaching the Energy Dispersive X-Ray Fluoresence Spectrometer (EDX) to the SEM (), the selected regions showed that C and Fe were the main elements constituting the ACF/nZVI composites, and partial oxidation occurred during nZVI preparation. Therefore, the ACF/nZVI composites contain a certain amount of O. The peaks of Fe in the EDX images were low, and the mass fraction of Fe in the ACF/nZVI composites was 7.20%. The surface atomic ratio of ACF/nZVI composites in the selected region was C: O: Fe = 61.38: 36.78: 1.84. In summary, it was further confirmed that nZVI was successfully loaded onto the surface of ACF in a dispersed form.
Figure 5. (A–b) Scanning electron micrograph of ACF; (c–d) Scanning electron micrograph of ACF/nZVI; (e–f) Scanning electron micrograph of nickel foam.
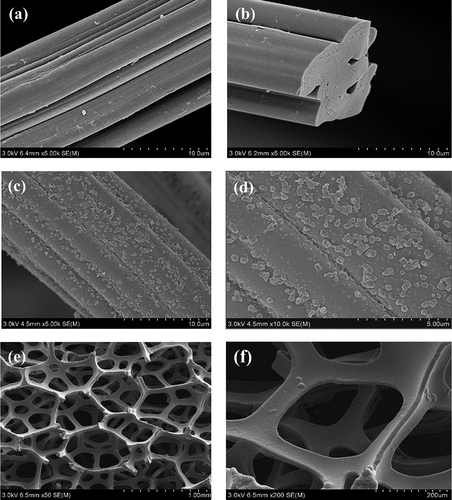
After 30 min of electrolysis, algal cells remained attached to the surface of the cathode, which may be due to the algal cell contents (e.g. polysaccharides, proteins, peptides, monosaccharides, and another organic acid) [Citation35] dissolved and adhered to the surface of the algal cells. These substances may be protonated in an acidic environment, which makes the surface of the algal cells positively charged. Meanwhile, the surface of the ACF contains many oxygen-containing functional groups, such as carboxyl, carbonyl, and hydroxyl groups, which have good adsorption effects on the organic substances. The algal cells were then adsorbed on the cathode surface by electrostatic interaction, van der Waals forces, and hydrogen bonding between the cellular contents on the surface and the ACF surface [Citation36] ().
3.3.2 ACF/nZVI FTIR spectral analysis
FTIR was used to analyze the differences in ACF/nZVI composites before and after electrolysis (). In addition to a small change in the intensity of the peaks before and after electrolysis, the change in peak position and the appearance of new characteristic peaks were less, inferring that fewer new functional groups were produced in the ACF molecular bonds. Among them, the width of the telescopic vibrational absorption peak of –OH corresponding to the wave number 3438.02 cm−1 of the infrared spectrogram of the ACF/nZVI composite after electrolysis was enhanced, and the intensity was changed. We hypothesized that this group was involved in the adsorption process in the reaction [Citation37]. The intensity of the stretching vibration peak of the oxygen-containing functional group –C=O- at 1637.17 cm−1 was weakened, indicating that the adsorption capacity of ACF was reduced, which coincided with the adsorption phenomenon of the algal cells observed during the experimental process, and the results of the electron microscopy analysis [Citation38]. 2920.94 and 2850.89 cm−1 correspond to C-H stretching vibrations [Citation39]. In the figure, 1069.01 cm−1 is attributed to the stretching vibration of -C-O- [Citation40]. The appearance of the N=O vibrational peak at 1384.25 cm−NaN Invalid Date NaNbe due to the oxidation of the nitrogen-containing functional groups of ACF during the generation of H2O2 from O2 on the surface of ACF [Citation41]. 603.04 cm−1 corresponds to the absorption peak of the Fe-oxidized Fe-O bond [Citation42], and the width of this peak is significantly enhanced in ACF after electrolysis. Combined with the results of XRD analysis, this suggests that a non-homogeneous electrical Fenton reaction occurs at the cathode surface allowing solid phase iron to attach to the ACF surface.
3.3.3 SEM analysis of algal cells
The integrity of algal cells is an important factor in ensuring their growth and reproduction. To investigate the effect of the Pt/Ti-ACF/nZVI/Ni system on the morphology of algal cells, scanning electron microscopy was used to observe the morphology of algal cells before and after electrolysis (). The algal cells before electrolysis were close to ellipsoidal with full morphology and complete structure . In contrast, the morphology of the algal cells after electrolysis was incomplete, with depressions on the cell surface and a large amount of material dissolved around the cells. This indicates that the algal cells had ruptured. Therefore, the Pt/Ti-ACF/nZVI/Ni system damaged the algal cell structure, which further caused their death.
3.3.4 FTIR spectroscopic analysis of algal cells
Microcystis aeruginosa cellular composition before and after electrolysis was analyzed by FTIR (). The positions of the main absorption peaks in the infrared spectra of Microcystis aeruginosa before and after electrolysis were roughly the same, but the intensities of the peaks were relatively weakened. The stretching vibrational region of saturated C-H was 2922 cm−1, and the intensity of its absorption peak decreased, which may indicate the disruption of carbon-hydrogen bonds in the protein structure [Citation43,Citation44]. The location of the O-H bond in the cell wall chitosan and protein of the algal cell was 2851.23 cm−1, and during algal removal, the O-H bond at this location stretched and vibrated, the cell wall and protein structure was destroyed, and the algal cell ruptured [Citation45]. The -C-O bending vibrational region was 1078.40 cm−1, and the intensity of the absorption peak decreased, which may indicate that the carbon-oxygen bond was broken in the protein structure [Citation46]. The O-H and N-H stretching vibrations in cellular polysaccharides and proteins were 3418.63 cm−1 [Citation47]. The absorption peaks at 1654.04 and 1648.44 cm−1, 1545.95 and 1539.95 cm−1, 1406.24 and 1400.92 cm −1 of the absorption peaks were shifted, and occurred due to -C-O bending vibration [Citation44,Citation48], C=O (amide II band) stretching vibration, and –COOH induced vibration [Citation49], respectively. Wave numbers below 1000 cm−1 correspond to C–H vibrations on aliphatic or aromatic groups [Citation50].
3.3.5 Three-dimensional fluorescence analysis of algal cells
This study determined the three-dimensional fluorescence of Microcystis aeruginosa before and after electrolysis; the fluorescence characteristic peak labeled A has the strongest signal and is located at 274–283/325–339 nm excitation/emission (Ex/Em), which belongs to a protein-like substance containing tryptophan [Citation51] . The characteristic peak B was observed at 229–234/324–343 nm (Ex/Em), which is located in the region of complex amino acid aromatic protein [Citation52]. After 30 min of electrolysis, all regions showed weaker fluorescence signals than the initial cycle . This implies that the Pt/Ti-ACF/nZVI/Ni system achieves protein-like substances of tryptophan and complex aromatic proteins degradation during algal removal. Interestingly, the characteristic peak C appeared at 251–282/432–471 nm (Ex/Em) after 30 min of electrolysis, and this peak was located in the humic acid region [Citation52]. The increase in humic acid content is due to the release of humic acid-like substances into solution by the algae as a result of cell rupture during the reaction [Citation53].
3.4 Mechanisms based on •OH
Due to the limitations of laboratory conditions, hydroxyl radical generation could not be detected directly, and the presence and role of •OH could only be verified indirectly by using tertiary butyl alcohol (TBA), a radical trapping agent, to capture •OH [Citation54,Citation55]. Keeping the conditions of electrolysis experiments unchanged, 0, 100, 200, and 250 mmol/L tert-butanol were added to the algal solution of ACF/nZVI/Ni system to quench •OH, respectively. Meanwhile, to exclude the effect of ACF/Ni cathode, •OH was quenched by adding 0 and 250 mmol/L tert-butanol to the Pt/Ti-ACF/Ni electrochemical system, respectively. The presence of •OH and its role was indirectly verified by detecting and comparing the changes in algal removal rates of the two systems.
presents that with the increase of tert-butanol concentration, the Inhibition effect of •OH in the Pt/Ti-ACF/nZVI/Ni system was more significant, when adding 100, 200 and 250 mmol/L of tert-butanol and electrolyzing for 30 min, the rate of algal removal decreased from 94.20% to 66.67%, 43.50%, and 40.06%, while the rate of algal removal only decreased from 64.87% to 59.71% for the Pt/Ti-ACF/nZVI/Ni system. This indicates that the Pt/Ti-ACF/nZVI/Ni system can produce a large amount of •OH, and the presence of nZVI significantly promotes •OH production in this system, which leads to the rupture and death of more algal cells. Therefore, the Pt/Ti-ACF/nZVI/Ni system may have undergone an EF reaction. In the Pt/Ti-ACF/Ni system, the effect of tert-butanol on the algal alga removal rate was insignificant, which might be because other substances besides •OH played a major role in algae treatment.
3.5 Mechanisms based on •O2−
According to He J et al. [Citation19], the non-homogeneous Fenton reaction produces •O2− in addition to •OH as the reactive species. Therefore, a neutral electrochemical system was constructed by replacing the algal solution with 5 × 10−4 mol/L NBT solution and using ACF/nZVI/Ni as the cathode. The other reaction conditions remained unchanged, and the NBT concentration was detected spectrophotometrically after 10-fold dilution of the samples to indirectly verify whether the Pt/Ti- ACF/nZVI/Ni electrochemical system could produce •O2− under the neutral conditions by the change of NBT concentration in the electrolysis experiments. The Pt/Ti-ACF/Ni system was examined for •O2− production under energized and neutral conditions.
indicates that the removal rates of Pt/Ti-ACF/nZVI/Ni and Pt/Ti-ACF/Ni systems for NBT were 79.28% and 58.05%, respectively. The production of the black-purple material in the solution indicates that when the cathode was ACF/nZVI/Ni and ACF/Ni, the systems produced a large amount of •O2−. Similarly, the Pt/Ti-ACF/Ni system produced a large amount of •O2−, which may also explain the ability of the Pt/Ti-ACF/Ni system to remove algal cells.
3.6 Synergistic mechanisms
In this electrochemical system, the Pt/Ti anode has good electro-catalytic properties to induce the oxidation reaction of water. The cathode consists of nZVI, ACF and Ni. The ACF provides a large surface area that facilitates the reaction. The nZVI has good electrical conductivity and electro-catalytic effect, which can accelerate the reaction and significantly promote the degradation of Microcystis aeruginosa. Foam Ni is an electrically conductive material that provides the conductivity of the electric current. In this electrolytic cell, the potential difference created by the applied direct current power source causes an electric field to form between the anode and the cathode. Under the action of the electric field, the water molecules on the anode undergo an oxidation reaction, producing oxygen and hydrogen ions. At the same time, an electro-Fenton reaction occurs at the cathode to generate hydroxyl radicals (•OH) to degrade Microcystis aeruginosa. In this process, the ions in the electrolyte solution between the anode and cathode participate in the movement of the also current, thus forming a complete closed loop.
The reaction mechanism of the Pt/Ti-ACF/nZVI/Ni electrochemical algae removal system was as follows (). First, the anodic electrolysis of water in the solution produced O2 and H+, which generated electro-floatation to remove algae; O2 got electrons at the ACF/nZVI/Ni cathode and reacted with H+ to generate H2O2, and the nZVI on the cathode reacted to become Fe2+, which reacted with H2O2 to generate •OH to degrade the algal cells. Specifically, the algal cells are oxidized and ruptured through the EF reaction and release the cell contents. The cellular contents adhere to the surface of the algal cells, thereby causing the algal cells to become positively charged and adsorbed to the cathode surface through electrostatic interactions, hydrogen bonding, and van der Waals forces, consistent with the SEM results. Meanwhile, Fe2+ becomes Fe3+ in the cathode for the in situ cycling of Fe0→Fen+, in which Fe3+ hydrolysis may lead to electro-flocculation and algal cell removal. The Pt/Ti-ACF/nZVI/Ni can generate numerous •O2− and •OH to inactivate the algal cells.
4. Conclusion
This study prepared ACF/nZVI/Ni composite cathodes by liquid-phase reduction method to remove Microcystis aeruginosa, the main algal species in cyanobacterial blooms. The results showed that the algal removal rate could reach 94.20% at a pH of 6.0, electrode distance of 10 mm, current density of 75 mA/cm2, and initial algal concentration of OD680 = 0.100 with 30 min electrolysis. In the Pt/Ti-ACF/nZVI/Ni electrochemical system, the reaction was dominated by the non-homogeneous-EF reaction that produced massive •OH and •O−2, accompanied by the synergistic effects of electro-absorption, electro-flotation, and electro-flocculation.
CRediT authorship contribution statement
Guoming Zeng: Conceptualization, Methodology, Software, Validation, Formal analysis, Visualization, Writing – original draft. Da Sun: Project administration, Funding acquisition, Writing – review& editing. Rui Zhang: Writing – review & editing. Cenhui Liao: Investigation, Writing – review &editing. Dong Liang: Investigation, Writing – review & editing. Fei Wang: Investigation. Qihui Wang: Conceptualization, Methodology, Writing – review & editing, Supervision, Resources, Funding acquisition. Xiaoling Lei: Investigation.
Disclosure statement
No potential conflict of interest was reported by the author(s).
Data availability statement
Data will be made available on request.
Additional information
Funding
References
- Wu Y, Ding H, Wan L, et al. Single and combined toxic effects of clarithromycin and levofloxacin on Microcystis aeruginosa. Environ Pollut Bioavailabil. 2022;34(1):482–101. doi: 10.1080/26395940.2022.2130825
- Hadiyanto H, Khoironi A, Dianratri I, et al. Biodegradation of oxidized high-density polyethylene and oxo-degradable plastic using microalgae dunaliella salina. Environ Pollut Bioavailabil. 2022;34(1):469–481. doi: 10.1080/26395940.2022.2128884
- Zhang Y, Zhang J, Song K, et al. Potential of biochar derived from three biomass wastes as an electrode catalyzing oxygen reduction reaction. Environ Pollut Bioavailabil. 2022;34(1):42–50. doi: 10.1080/26395940.2021.2022538
- Che N, Liu L, Liu Y, et al. Application and influence factors of capacitive deionization method for removing inorganic contaminated ions. Environ Pollut Bioavailabil. 2021;33(1):365–376. doi: 10.1080/26395940.2021.1990798
- Brillas E, Sires I, Oturan MA. Electro-fenton process and related electrochemical technologies based on Fenton’s reaction chemistry. Chem Rev. 2009;109(12):6570–6631. doi: 10.1021/cr900136g
- Nidheesh PV, Gandhimathi R. Trends in electro-fenton process for water and wastewater treatment: an overview. Desalination. 2012;299:1–15. doi: 10.1016/j.desal.2012.05.011
- Li N, He X, Ye J, et al. H2O2 activation and contaminants removal in heterogeneous Fenton-like systems. J Hazard Mater. 2023;458:131926. doi: 10.1016/j.jhazmat.2023.131926
- Zhao H, Wang Y, Wang Y, et al. Electro-Fenton oxidation of pesticides with a novel Fe3O4@Fe2O3/activated carbon aerogel cathode: high activity, wide pH range and catalytic mechanism. Appl Catal B Environ. 2012;125:120–127. doi: 10.1016/j.apcatb.2012.05.044
- Li H, Lei H, Chen K, et al. A nano-Fe0/ACF cathode applied to neutral electro-Fenton degradation of orange II. J Chem Tech Biotech. 2011;86(3):398–405. doi: 10.1002/jctb.2530
- Li T, Zhu F, Gao Y, et al. Efficient elimination of Cr(vi) in groundwater using nano zero-valent iron synthesized with ginkgo biloba extracts: enhanced mechanism and reduced toxicity. Environ Sci Water Res Technol. 2024. doi: 10.1039/d3ew00479a
- Zhu F, Wu Y, Liang Y, et al. Degradation mechanism of norfloxacin in water using persulfate activated by BC@nZVI/Ni. Chem Eng J. 2020;389:124276. doi: 10.1016/j.cej.2020.124276
- Zhu F, Ma S, Liu T, et al. Green synthesis of nano zero-valent iron/Cu by green tea to remove hexavalent chromium from groundwater. J Clean Prod. 2018;174:184–190. doi: 10.1016/j.jclepro.2017.10.302
- An J, Li N, Wang S, et al. A novel electro-coagulation-fenton for energy efficient cyanobacteria and cyanotoxins removal without chemical addition. J Hazard Mater. 2019;365:650–658. doi: 10.1016/j.jhazmat.2018.11.058
- Das S, Raj R, Ghangrekar MM. Efficient algal lipid extraction via a green bio-electro-fenton process and its conversion into biofuel and bioelectricity with concurrent wastewater treatment in a photosynthetic microbial fuel cell. Green Chem. 2023;25(18):7166–7182. doi: 10.1039/D3GC01548C
- Long Y, Li H, Xing X, et al. Enhanced removal of Microcystis aeruginosa in BDD-CF electrochemical system by simple addition of Fe2+. Chem Eng J. 2017;325:360–368. doi: 10.1016/j.cej.2017.05.067
- Zhang J, Wang H. Study on mechanism of algal inactivation and pollution removal by Fe-ACF electro fenton-like process. Water Sci Technol. 2015;72(10):1700–1712. doi: 10.2166/wst.2015.388
- Liu Y, Zhao Y, Wang J. Fenton/fenton-like processes with in-situ production of hydrogen peroxide/hydroxyl radical for degradation of emerging contaminants: advances and prospects. J Hazard Mater. 2021;404:124191. doi: 10.1016/j.jhazmat.2020.124191
- Sun M, Ru X-R, Zhai L-F. In-situ fabrication of supported iron oxides from synthetic acid mine drainage: high catalytic activities and good stabilities towards electro-Fenton reaction. Appl Catal B Environ. 2015;165:103–110. doi: 10.1016/j.apcatb.2014.09.077
- He J, Yang X, Men B, et al. Interfacial mechanisms of heterogeneous fenton reactions catalyzed by iron-based materials: a review. J Environ Sci. 2016;39:97–109. doi: 10.1016/j.jes.2015.12.003
- Yu F, Wang Y, Ma H, et al. Enhancing the yield of hydrogen peroxide and phenol degradation via a synergistic effect of photoelectrocatalysis using a g-C3N4/ACF electrode. Int J Hydrogen Energy. 2018;43(42):19500–19509. doi: 10.1016/j.ijhydene.2018.08.217
- Lian H, Xiang P, Xue Y, et al. Efficiency and mechanisms of simultaneous removal of Microcystis aeruginosa and microcystins by electrochemical technology using activated carbon fiber/nickel foam as cathode material. Chemosphere. 2020;252:126431. doi: 10.1016/j.chemosphere.2020.126431
- Ma X, Chen Y, Liu F, et al. Enhanced tolerance and resistance characteristics of scenedesmus obliquus FACHB-12 with K3 carrier in cadmium polluted water. Algal Res. 2021;55:102267. doi: 10.1016/j.algal.2021.102267
- Palanivel B, Hu C, Shkir M, et al. Fluorine doped g-C3N4 coupled NiFe2O4 heterojunction: consumption of H2O2 for production of hydroxyl radicals towards paracetamol degradation. Coll Inter Sci Commun. 2021;42:100410. doi: 10.1016/j.colcom.2021.100410
- Zhang G, Wang S, Yang F. Efficient adsorption and combined heterogeneous/homogeneous Fenton oxidation of Amaranth using supported nano-FeOOH as cathodic catalysts. J Phys Chem C. 2012;116(5):3623–3634. doi: 10.1021/jp210167b
- Li J, Ai Z, Zhang L. Design of a neutral electro-Fenton system with Fe@Fe2O3/ACF composite cathode for wastewater treatment. J Hazard Mater. 2009;164(1):18–25. doi: 10.1016/j.jhazmat.2008.07.109
- Hou B, Han H, Jia S, et al. Heterogeneous electro-Fenton oxidation of catechol catalyzed by nano-Fe3O4: kinetics with the Fermi’s equation. J Taiwan Inst Chem Eng. 2015;56:138–147. doi: 10.1016/j.jtice.2015.04.017
- Khataee AR, Safarpour M, Zarei M, et al. Electrochemical generation of H2O2 using immobilized carbon nanotubes on graphite electrode fed with air: investigation of operational parameters. J Electroanal Chem. 2011;659(1):63–68. doi: 10.1016/j.jelechem.2011.05.002
- Wang X, Xiang P, Zhang Y, et al. The inhibition of microcystis aeruginos by electrochemical oxidation using boron-doped diamond electrode. Environ Sci Pollut Res Int. 2018;25(21):20631–20639. doi: 10.1007/s11356-018-1977-3
- Rastegar SO, Mousavi SM, Shojaosadati SA, et al. Optimization of petroleum refinery effluent treatment in a UASB reactor using response surface methodology. J Hazard Mater. 2011;197:26–32. doi: 10.1016/j.jhazmat.2011.09.052
- He S, Zhu F, Li L, et al. Box–Behnken design for the optimization of the removal of Cr(VI) in soil leachate using nZVI/Ni bimetallic particles. Soil Sediment Contam An Int J. 2018;27(8):658–673. doi: 10.1080/15320383.2018.1502744
- Zhang Z, Ren W, Zhang J, et al. Electrokinetic remediation of Pb near the e-waste dismantle site with Fe(NO3)3 as cathode electrolyte. Environ Technol. 2021;42(6):884–893. doi: 10.1080/09593330.2019.1648559
- Bakheet B, Islam MA, Beardall J, et al. Electrochemical inactivation of Cylindrospermopsis raciborskii and removal of the cyanotoxin cylindrospermopsin. J Hazard Mater. 2018;344:241–248. doi: 10.1016/j.jhazmat.2017.10.024
- Zhu F, Li L, Ma S, et al. Effect factors, kinetics and thermodynamics of remediation in the chromium contaminated soils by nanoscale zero valent Fe/Cu bimetallic particles. Chem Eng J. 2016;302:663–669. doi: 10.1016/j.cej.2016.05.072
- Zhu F, Liu T, Zhang Z, et al. Remediation of hexavalent chromium in column by green synthesized nanoscale zero-valent iron/nickel: factors, migration model and numerical simulation. Ecotoxicol Environ Saf. 2021;207:111572. doi: 10.1016/j.ecoenv.2020.111572
- Huang J, Graham N, Templeton MR, et al. A comparison of the role of two blue–green algae in THM and HAA formation. Water Res. 2009;43(12):3009–3018. doi: 10.1016/j.watres.2009.04.029
- Le Cloirec P, Brasquet C, Subrenat E. Adsorption onto fibrous activated carbon: applications to water treatment. Energy Fuels. 1997;11(2):331–336. doi: 10.1021/ef9601430
- Liu Z, Zhao C, Wang P, et al. Removal of carbamazepine in water by electro-activated carbon fiber-peroxydisulfate: comparison, optimization, recycle, and mechanism study. Chem Eng J. 2018;343:28–36. doi: 10.1016/j.cej.2018.02.114
- Mishra S, Dwivedi J, Kumar A, et al. Studies on salophen anchored micro/meso porous activated carbon fibres for the removal and recovery of uranium. RSC Adv. 2015;5(42):33023–33036. doi: 10.1039/C5RA03168K
- Liu LF, Zhang PH, Yang FL. Adsorptive removal of 2,4-DCP from water by fresh or regenerated chitosan/ACF/TiO2 membrane. Sep Purif Technol. 2010;70(3):354–361. doi: 10.1016/j.seppur.2009.10.022
- Li T, Zhu F, Liang W, et al. Simultaneous removal of p-nitrophenol and Cr(VI) using biochar supported green synthetic nano zero valent iron-copper: mechanistic insights and toxicity evaluation. Process Saf Environ Prot. 2022;167:629–640. doi: 10.1016/j.psep.2022.09.049
- Dung NT, Duong LT, Hoa NT, et al. A comprehensive study on the heterogeneous electro-Fenton degradation of tartrazine in water using CoFe2O4/carbon felt cathode. Chemosphere. 2022;287:132141. doi: 10.1016/j.chemosphere.2021.132141
- Lan H, Wang A, Liu R, et al. Heterogeneous photo-Fenton degradation of acid red B over Fe2O3 supported on activated carbon fiber. J Hazard Mater. 2015;285:167–172. doi: 10.1016/j.jhazmat.2014.10.057
- Kim DJ, Lee HI, Yie JE, et al. Ordered mesoporous carbons: implication of surface chemistry, pore structure and adsorption of methyl mercaptan. Carbon. 2005;43(9):1868–1873. doi: 10.1016/j.carbon.2005.02.035
- Yi ZJ, Yao J, Chen HL, et al. Uranium biosorption from aqueous solution onto Eichhornia crassipes. J Environ Radioact. 2016;154:43–51. doi: 10.1016/j.jenvrad.2016.01.012
- Wang H, Wang H, Zhao H, et al. Adsorption and Fenton-like removal of chelated nickel from Zn-ni alloy electroplating wastewater using activated biochar composite derived from taihu blue algae. Chem Eng J. 2020;379:122372. doi: 10.1016/j.cej.2019.122372
- Mecozzi M, Onorati F, Oteri F, et al. Characterisation of a bioassay using the marine alga Dunaliella tertiolecta associated with spectroscopic (visible and infrared) detection. Int J Environ Pollut. 2008;32(1):104–120. doi: 10.1504/IJEP.2008.016902
- Fernando IPS, Sanjeewa KKA, Samarakoon KW, et al. FTIR characterization and antioxidant activity of water soluble crude polysaccharides of Sri Lankan marine algae. Algae. 2017;32(1):75–86. doi: 10.4490/algae.2017.32.12.1
- Zou W, Zhao L. Removal of uranium(VI) from aqueous solution using citric acid modified pine sawdust: batch and column studies. J Radioanal Nucl Chem. 2011;292(2):585–595. doi: 10.1007/s10967-011-1452-9
- Xiong Q, Hu LX, Liu YS, et al. New insight into the toxic effects of chloramphenicol and roxithromycin to algae using FTIR spectroscopy. Aquat Toxicol. 2019;207:197–207. doi: 10.1016/j.aquatox.2018.12.017
- Xu C, Li W, Tan B, et al. Adsorption of Gardenia jasminoides fruits extract on the interface of Cu/H2SO4 to inhibit Cu corrosion: experimental and theoretical studies. J Mol Liq. 2022;345:116996. doi: 10.1016/j.molliq.2021.116996
- Xiaoling Z, Gaofang Y, Nanjing Z, et al. Chromophoric dissolved organic matter influence correction of algal concentration measurements using three-dimensional fluorescence spectra. Spectrochim Acta A Mol Biomol Spectrosc. 2019;210:405–411. doi: 10.1016/j.saa.2018.10.050
- Kang J, Ma T, Zhou Q, et al. New insight into DOC and DON in a drinking water Biological Aerated Filter (BAF) by multimethod and correlation analysis of 3D-EEM. Anal Methods. 2015;7(23):9885–9893. doi: 10.1039/C5AY02156A
- Fan G, Chen Z, Yan Z, et al. Efficient integration of plasmonic Ag/AgCl with perovskite-type LaFeO3: enhanced visible-light photocatalytic activity for removal of harmful algae. J Hazard Mater. 2021;409:125018. doi: 10.1016/j.jhazmat.2020.125018
- Kozmér Z, Takács E, Wojnárovits L, et al. The influence of radical transfer and scavenger materials in various concentrations on the gamma radiolysis of phenol. Radiat Phys Chem. 2016;124:52–57. doi: 10.1016/j.radphyschem.2015.12.011
- Lee W, Lee Y, Allard S, et al. Mechanistic and kinetic understanding of the UV254 Photolysis of chlorine and bromine species in water and formation of oxyhalides. Environ Sci Technol. 2020;54(18):11546–11555. doi: 10.1021/acs.est.0c02698