ABSTRACT
In this report, Acinetobacter calcoaceticus Aca13 was isolated from crude oil-polluted soil. The optimization of growth conditions showed that Acinetobacter calcoaceticus Aca13 can tolerate a wide range of temperatures (14–42°C). The whole-genome sequence showed that the circular chromosome of Acinetobacter calcoaceticus Aca13 was 3,966,524 bp in size, with an average GC content of 38.78%. Whole-genome analysis revealed that 39 cytochrome proteins, 49 monooxygenases and 46 dioxygenases might be related to hexadecane and naphthalene degradation. Whole-transcriptome analysis revealed that six dioxygenases could be induced by naphthalene and that one monooxygenase could be significantly induced by hexadecane. Both genomic and transcriptional results showed that alkane 1-monooxygenase is the key enzyme for hexadecane degradation and that aromatic ring-hydroxylating dioxygenase is the key enzyme for naphthalene degradation. The present study contributes to the genomic and transcriptomic databases of crude oil-degrading microorganisms.
Introduction
Crude oil pollution has been and remains an important problem because of its refractory resistance to environmental degradation. Crude oil is a complex mixture of alkanes, polycyclic aromatic hydrocarbons and other compounds. Among these, alkanes constitute the major fraction, accounting for up to 20–50% of crude oil [Citation1,Citation2]. Alkanes are saturated hydrocarbons of different sizes and structures formed exclusively by carbon and hydrogen atoms. These alkanes are classified as short-, medium- or long-chain alkanes according to size [Citation3]. Polycyclic aromatic hydrocarbons (PAHs) are a large group of diverse organic compounds that contain two or more fused aromatic rings ranging from two-ring naphthalene and naphthalene derivatives to complex ring structures containing up to 10 rings. Most alkanes and PAHs are apolar molecules that are chemically inert [Citation4]. Crude oil, which includes alkanes and polycyclic aromatic hydrocarbons, is difficult to degrade.
Microorganisms have enormous metabolic capacity and adaptive abilities. Scientists are committed to finding appropriate microbial remediation methods for crude oil pollution. Some reported microorganisms, such as bacteria, archaea, filamentous fungi and yeasts, can use crude oil as a source of carbon and energy. Among these, oil-degrading bacteria have been extensively studied Fernando [Citation5–7]. The genera Acinetobacter, Pseudomonas, Bacillus, and Rhodococcus are the main crude oil-degrading bacteria isolated from polluted environments Fernando [Citation6,Citation8]. Most crude oil-degrading bacteria can only use a narrow range of substrates. For example, Bacillus stearothermophilus can only utilize C15-C17 alkanes, whereas A. borkumensis strains can only utilize C6-C16 alkanes [Citation9,Citation10]. Recently, some bacteria successfully isolated by scientists have been shown to degrade a wide range of alkanes. For example, Gordonia iterans Co17 can use C8-C32 alkanes [Citation8], and Acinetobacter pittii H9–3 can use C10-C36 alkanes [Citation8]. Overall, there has been some progress in understanding the genetic and metabolic characteristics of microorganisms that can degrade oil, but more research is needed in the future.
The Shengli Oil Field is the second largest oilfield in China. The surrounding soil and water environments are polluted by crude oil. Our group hopes to use microorganisms combined with physicochemical methods for the elimination of oil contaminants in the future. The first and most important step is to obtain crude oil-degrading microorganisms adapted to the local environment. Oil-polluted soil and water samples were collected from the Shengli Oil Field and then used for bacterial isolation. Several bacteria were obtained successfully. Acinetobacter calcoaceticus Aca13 was the isolate that showed the strongest degradation ability. Strain Aca13 grew well in mineral salt medium supplemented with naphthalene. Therefore, in this study, hexadecane and naphthalene were chosen as representative substrates for transcriptome induction. The breakdown efficiency of hexadecane and naphthalene by Acinetobacter calcoaceticus Aca13 was first investigated. Then, genomic and transcriptional analyses were used to identify oil-degrading genes in strain Aca13. Finally, aerobic pathways for the degradation of hexadecane and naphthalene in Acinetobacter calcoaceticus Aca13 were discussed.
Materials and methods
Chemicals, enzymes, and kits
Crude oil was obtained from the Shengli Oil Field. N-alkanes (>99% purity), naphthalene (>98% purity) and phenanthrene (>98% purity) were purchased from Macklin Biochemical Co. (Shanghai, China). Ethanol, acetonitrile, ethyl acetate, and chloroform were purchased from Thermo Fisher Scientific (U.S.A., HPLC grade). LB broth, SOC broth, agar powder, Petri dishes, 96-well plates, and other chemicals were obtained from Sangon Biotech (Shanghai, China). The RNAprotect Bacteria Reagent was obtained from QIAGEN (Germany). The PrimeScript Reverse Transcriptase Kit, PrimeScriptTM RT Reagent Kit and TB GreenTM Premix Ex TaqTM Kit were obtained from TaKaRa, China. The NEBNext Ultra Directional RNA Library Prep Kit was obtained from NEB, China. The DNA extraction kit, RNase and RNase-free DNase I were obtained from Tiangen, China.
Enrichment, isolation, and identification of crude oil-degrading bacteria
To enrich crude oil-degrading bacteria, oil-polluted soil was added to a 250 mL flask containing 100 mL of sterile mineral salt medium (without carbon) supplemented with crude oil as a carbon and energy source. The ingredients of the mineral salt medium in 1 L of Milli-Q water were as follows: NaNO3, 1.5 g; (NH4)2SO4, 1.5 g; K2HPO4, 1 g; MgSO4·7 H2O, 0.5 g; KCl, 0.5 g; FeSO4·7 H2O, 0.01 g; and CaCl2, 0.002 g. The enrichment culture was shaken at 150 rpm in an orbital shaker for 3 days at 28°C, transferred to fresh mineral salt medium and incubated for two more days. The resulting culture was spread onto mineral salt media plates containing agar and incubated at 28°C for 2 days. Colonies were selected and inoculated into new fresh mineral salt liquid medium, which was further spread onto agar plates and then into mineral salt liquid medium. This process was repeated twice to obtain pure isolates. Several colonies were tested for their utilization of alkane and polycyclic aromatic hydrocarbons as the sole carbon source, and the one with the fastest growth rate was selected for further research.
The amplification and identification of 16S rDNA in Acinetobacter calcoaceticus Aca13 was performed with reference to the strain Gordonia sp. R9 [Citation11]. All 19 reported whole genomes of alkane- or PAH-degrading bacteria were chosen for phylogenetic tree construction based on core-pan genes. Whole-genome sequences were downloaded from NCBI. The phylogenetic tree included four Acinetobacter calcoaceticus strains, one Alloalcanivorax dieselolei B5 strain, two Gordonia alkanivorans strains, one Desulfatibacillum alkenivorans DSM 16,219 strain, one Desulfosudis oleivorans H×d3strain, two Mycolicibacterium vanbaalenii strains, one Polaromonas naphthalenivorans CJ2 strain, four Rhodococcus erythropolis strains, one Alcanivorax borkumensis SK2 strain, one Petrogoga mobilis SJ95 strain and one Alteromonas naphthalenivorans SN2 strain.
Growth rate of Acinetobacter calcoaceticus Aca13 in the presence of pure n-alkanes, naphthalene and phenanthrene
The cell growth rate of strain Aca13 in the presence of n-alkanes, naphthalene and phenanthrene was determined by OD600 measurement. A single colony of strain Aca13 was inoculated into a 100 mL baffled flask containing 20 mL of LB broth and cultured on a rotary shaker at 28°C overnight. Then, 50 μL of bacteria incubated overnight was inoculated into a 50 mL sterile centrifuge tube containing 5 mL of mineral salt medium supplemented with 0.5% pure n-alkanes (C9-C17). C18-C24, C28, C32, naphthalene and phenanthrene, which are solids, were supplied as solids at 0.5 g/L. Samples without substrate were used as controls. Temperatures of 14°C, 20°C, 28°C, 35°C and 42°C were also used. Samples were collected at different incubation time points (12 h, 24 h, 48 h, 72 h, 96 h, and 120 h). Three replicates of bacterial samples (200 μL) were pipetted into 96-well plates for each OD600 measurement. The results shown are the average values.
Sequencing and analysis of the Acinetobacter calcoaceticus Aca13 genome
The genomic DNA of strain Aca13 was extracted from an overnight culture using a bacterial genomic DNA extraction kit and then assessed for quantity and quality by agarose gel electrophoresis and an Agilent 2100 Bioanalyzer. The genome sequence of strain Aca13 was determined using both PacBio RSII and Illumina HiSeq 500. The clean data were generated from filtered raw sequencing data. The genome sequence of strain Aca13 was assembled with SMRT Analysis v2.3.0, Celera Assembler, SOAPanp, SOAPindel and GATK software. Clusters of Orthologous Groups (COG), Kyoto Encyclopedia of Genes and Genomes (KEGG), Swiss-Prot, Gene Ontology (GO), and Nonredundant Protein Database (NR) were used to predict gene functions in strain Aca13 [Citation12].
Sequencing and analysis of the Acinetobacter calcoaceticus Aca13 transcriptome
Acinetobacter calcoaceticus Aca13 was cultured in LB broth at 30°C. After 12 h of incubation, hexadecane and naphthalene were then added to the fresh culture for induction. After induction, the RNAprotect Bacteria Reagent was used on the samples for RNA isolation. Altogether, there were three groups containing nine samples, and each group had three replicates. Group 1 included three samples without induction after 3 h of incubation (designated ‘Aca1’, ‘Aca2’ and ‘Aca3’), Group 2 included three samples with hexadecane induction (designated ‘AcaH1’, ‘AcaH2’ and ‘AcaH3’), and Group 3 included three samples with naphthalene induction (designated ‘AcaN1’, ‘AcaN2’ and ‘AcaN3’). Among all nine samples, three samples from Group 1 (including ‘Aca1’, ‘Aca2’ and ‘Aca3’) were negative controls without substrate induction. All treated samples were centrifuged at 4°C for 10 min at 5000 × g. RNA extraction reagent was used for extraction of total RNA according to the manufacturer’s guidelines. For removal of genomic DNA, the RNA samples were treated with RNase-free DNase I. RNA degradation and contamination were monitored on 1% agarose gels. RNA integrity was assessed using the RNA Nano 6000 Assay Kit of the Bioanalyzer 2100 system (Agilent Technologies, CA, U.S.A.).
cDNA libraries for RNA sequencing were constructed according to the strand-specific library with the NEBNext Ultra Directional RNA Library Prep Kit (Illumina) according to the manufacturer’s instructions. Paired-end sequencing was performed on a NovaSeq 6000 sequencing platform. Clean reads were obtained by removing reads containing adapters, reads containing N bases and low-quality reads from the raw data. All downstream analyses were based on high-quality clean data. The reference genome and gene model annotation files of the Acinetobacter calcoaceticus Aca13 strain were downloaded from the NCBI database (genome accession number CP088954.1).
Rockhopper was used to identify novel genes, operons, transcription start sites (TSSs), transcription terminal sites (TTSs) and cis-natural antisense transcripts. The 700 bp upstream sequence of the transcription start site was extracted for promoter prediction using a time delay neural network (TDNN). According to the transcription and translation start/terminal site information, 5’ UTR (3’ UTR) sequences were extracted. Then, RBSfinder and TransTermH were used to predict the Shine‒Dalgarno (SD) sequence and terminator sequence, respectively. Rockhopper was used to identify new intergenic region transcripts, Blastx was compared with the NR library to annotate the newly predicted transgenic regions, and the unmarked transcripts were used as candidate noncoding sRNAs. HTSeq v0.6.1 was used to count the number of reads mapped to each gene. The fragments per kilobase of transcript sequence per million base pairs (FPKM) of each gene were calculated based on the length of the gene and the number of reads mapped to the gene.
Differential expression analysis of hexadecane and naphthalene induction was performed using the DESeq R package (1.18.0). DESeq provides statistical routines for determining differential expression in digital gene expression data using a model based on the negative binomial distribution. The resulting p values were adjusted using Benjamini and Hochberg’s approach for controlling the false discovery rate. Genes with an adjusted p value <0.05 according to DESeq were considered to be differentially expressed.
Quantitative real-time PCR (qRT‒PCR) analysis of hexadecane- and naphthalene-induced genes
To confirm the RNA-seq results, two inducible genes were selected for validation by qRT‒PCR. Alkane 1-monooxygenase (gene ID: LRO55_RS12655) was chosen for hexadecane induction, and aromatic ring-hydroxylating dioxygenase (gene ID: LRO55_RS12945) was chosen for naphthalene induction. 16S rRNA was chosen as the reference gene. Total RNA was extracted by using an RNAprotect® Bacteria kit according to the manufacturer’s instructions. The RNA yield was estimated by a nucleic acid quantitative instrument. Reverse transcription was completed by using the PrimeScript Reverse Transcriptase Kit. The qRT‒PCRs were performed using a Stratagene Mx3000P instrument. A TB GreenTM Premix Ex TaqTM kit and gene-specific primers were used for qRT‒PCR following the manufacturer’s guidelines. The primers for the 16S rRNA control were 5’ TACGGGAGAAAGCAGGGGAT 3’ (forward primer) and 5’ CGTGTCTCAGTCCCAGTGTG 3’ (reverse primer). The primers for the RS12655 gene were 5’ AAGACACAGAAAATCCGCCA 3’ (forward primer) and 5’ TTCCCACCAAAGTTCCCAGT 3’ (reverse primer). The primers for the RS12945 control gene were 5’ TTCAATGCCAGCTCAACGTG 3’ (forward primer) and 5’ CCTCCGCCATAGCTCCATTC 3’ (reverse primer). Each reaction was carried out in triplicate with a total reaction mixture of 20 µL final volume consisting of 1 µL of 50 ng/µL cDNA, 10 µL of TB GreenTM Premix Ex TaqTM II (Tli RNaseH Plus) (2X), 0.5 µL of PCR forward primer (10 µM), 0.5 µL of PCR reverse primer (10 µM), 0.4 µL of ROX Reference Dye II (50X) and 7.6 µL of sterile purified water. The qRT‒PCR procedure was as follows: 5 min of predenaturation, 40 cycles of 95°C for 10 s, 60°C for 30 s, and 72°C for 30 s, and a melting curve stage from 60°C to 95°C. The cDNA amplification efficiency of the samples, 16S rRNA internal standards, and controls without substrate induction were equivalently modulated, and the relative fold change in mRNA quantity was calculated according to the 2−△△Ct method [Citation13].
Results and discussion
Isolation and identification of the crude oil-degrading strain Acinetobacter calcoaceticus Aca13
Strain Aca13, which was isolated from the enrichment culture of crude oil-contaminated soil, is a gram-negative bacterium that is rod-shaped (cells in the stationary phase of growth are spherical). After growing on LB agar at 30°C for 36 h, the colonies were observed to be circular, convex, smooth, and slightly opaque with entire margins. Analysis of the 16S rRNA gene sequences revealed that strain Aca13 was identical to Acinetobacter calcoaceticus strains, with 99.6% similarity (accession number CP088954). A phylogenetic tree constructed by using whole genomes from crude oil-degrading strains based on core-pan genes showed that strain Aca13 was grouped with the other three Acinetobacter calcoaceticus strains (). Strain Aca13 was therefore designated Acinetobacter calcoaceticus Aca13. Acinetobacter is a genus of Gammaproteobacteria in the family Moraxellaceae. It has been estimated that at least 0.001% of the total culturable, heterotrophic, and aerobic population in soil and water are acinetobacters [Citation14]. Acinetobacters can degrade a wide variety of organic compounds, especially hydrocarbons and aromatic compounds. Since hydrocarbons and aromatic compounds constitute the major fraction of crude oil, acinetobacter species play a very important role in the degradation of crude oil. Therefore, they could be used for crude oil bioremediation [Citation14,Citation15]. In this study, our group investigated the global degradation mechanism of alkanes and aromatic compounds via genome and transcriptome analysis.
Figure 1. A phylogenetic tree was constructed by using whole genomes from 19 crude oil-degrading strains based on core-pan genes. All the reference whole-genome sequences were obtained from the NCBI. Single-copy core genes were identified by core-pan analysis. The number on the branch indicates the credibility of the branch, the branch length indicates the size of the evolutionary distance, and the evolutionary distance is based on the average number of replacements of each nucleotide.
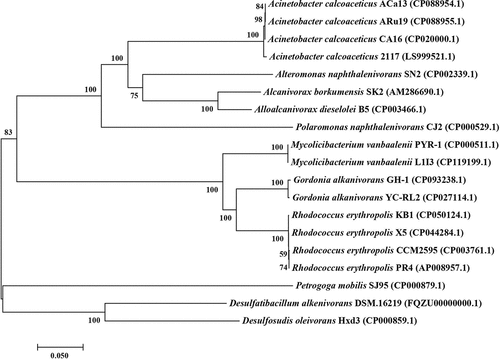
Growth curve of strain Aca13 in the presence of n-alkanes, naphthalene and phenanthrene
Previous studies have reported that Acinetobacter calcoaceticus can degrade hydrocarbons and aromatic compounds. It was therefore necessary to determine the bioavailability of Acinetobacter calcoaceticus Aca13 at different temperatures on different substrates. This result illustrated that 0.5% substrate could provide enough carbon and energy sources for the growth of strain Aca13. As shown in , strain Aca13 grew well in a mineral salt medium supplemented with n-alkanes (C14-C24) at 35°C, with an OD600 ranging from approximately 0.4–0.5. However, the OD600 value was lower than 0.4–0.5 in medium supplemented with other substrates (C9–12, C28, C32 and naphthalene). Strain Aca13 did not grow well in the medium supplemented with phenanthrene and did not grow at all in the controls. The results showed that strain Aca13 must utilize exogenous chemicals for growth and can use n-alkanes and naphthalene as sole carbon and energy sources. Strain Aca 13 could grow on C9-C11 alkanes but not very well. Strain Aca 13 grew better in naphthalene than in phenanthrene. The cells started multiplying immediately after 24 h of incubation, reached the exponential growth phase at 35°C, and had the highest OD600 value of 0.5. This suggested that 35°C is the optimal temperature for the growth of strain Aca13. Low temperatures caused a longer lag phase; for example, approximately 5 days were needed for the plants to start growing at 14°C, and approximately 3 days were needed for the bacteria to start growing at 20°C. However, it only took 1 day to reach the stationary phase at 35°C. shows that strain Aca 13 grew well in the medium supplemented with alkanes (C18-C32) at 42°C but could not utilize C9-C17 alkanes, naphthalene or phenanthrene. Although strain Aca could grow at a wide range of temperatures from 14°C to 42°C, the temperature had an impact on substrate utilization. The best temperature range for strain Aca13 growth was approximately 28–35°C.
Figure 2. Growth curves of Acinetobacter calcoaceticus Aca13 in the presence of n-alkanes, naphthalene and phenanthrene. The strains were inoculated in mineral salt medium supplemented with 0.5% n-alkanes or 0.5 g/L n-alkanes for solid substrates and cultured for seven days at different temperatures.
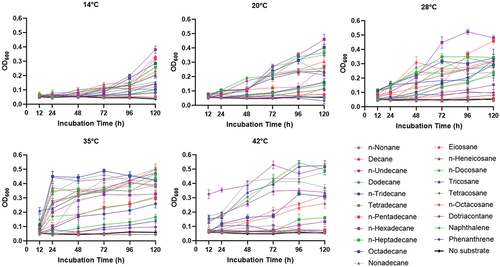
Whole-genome sequence analysis of alkane- and aromatic compound-degrading genes in strain Aca13
To investigate the mechanism underlying the degradation of crude oil by Acinetobacter calcoaceticus Aca13, chromosomal DNA was extracted and sequenced. The whole-genome DNA sequence of strain Aca13 was obtained and deposited in GenBank under the accession number CP088954.1. The complete genome of Acinetobacter calcoaceticus Aca13 is composed of one circular chromosome of 3,966,524 bp with an average GC content of 38.78%. The genome is predicted to contain 3725 putative protein-coding sequences, 18 rRNA genes, 73 tRNA genes, and 1 sRNA gene.
Monooxygenase and dioxygenase have been reported to be key enzymes for alkane and aromatic compound degradation, respectively [Citation16–18]. Forty-nine monooxygenase genes and 46 dioxygenase genes were found in the genome of Acinetobacter calcoaceticus Aca13. All acinetobacters lack cytochrome c but contain cytochromes b, o, and occasionally d and p-450, as well as flavoproteins, for a normal electron transport system [Citation14]. Indeed, no cytochrome c was detected in the Acinetobacter calcoaceticus Aca13 genome, but 39 other cytochrome genes were detected, including 11 cytochrome P450 genes. Among all the cytochrome genes, cytochrome ubiquinol oxidase is important for aromatic compound degradation. There were four cytochrome ubiquinol oxidases found in the Aca13 genome. Interestingly, two novel cytochrome bd terminal oxidases (Novel00194 and Novel00296) were identified. These genes found in the Acinetobacter calcoaceticus Aca13 genome might be involved in the degradation of alkanes and aromatic compounds.
Whole-transcriptome analysis of hexadecane and naphthalene degradation genes in strain Aca13
To investigate the highly expressed genes involved in crude oil degradation, we performed whole-transcriptome analysis by using RNA-Seq [Citation19,Citation20]. Hexadecane was chosen as the substrate for alkanes, and naphthalene was chosen as the substrate for aromatic compounds in crude oil. All the data were submitted to NCBI GEO (accession number GSE235642). The detailed sequencing information and quality control data are shown in . All sequenced transcripts were used to map annotated genes in the genome sequence of Acinetobacter calcoaceticus Aca13. According to the assembly results, 4175 genes were found in Acinetobacter calcoaceticus Aca13 transcripts, including 3731 annotated genes, 428 novel genes and 16 small RNA genes. The gene structures predicted in Acinetobacter calcoaceticus Aca13 include 121 SD sequences, 400 3’UTRs, 225 5’UTRs, 106 terminators, and 602 operons. According to whole-transcriptome sequence analysis, six dioxygenases, four cytochrome oxidases and one alkane-1-monooxygenase could be significantly upregulated by naphthalene. One key enzyme of alkane-1-monooxygenase was induced by hexadecane. Detailed information on the key upregulated enzymes is listed in .
Table 1. Total reads of strain Acinetobacter calcoaceticus Aca13 (control and treated) generated by using NovaSeq 6000 platform.
Table 2. Up-regulated key genes found in Acinetobacter calcoaceticus Aca13 by whole transcriptome analysis.
There were 166 genes whose expression could be induced by hexadecane. Alkane-1-monooxygenase (gene ID: LRO55_RS12655) had the greatest log2-fold change among all the inducible genes. The log2-fold change in alkane-1-monooxygenase was greater than 5. Alkane-1-monooxygenase is the key enzyme for alkane degradation. The length of the LRO55_RS12655 gene is 1194 bp. According to the whole-transcriptome analysis results, most of the hexadecane-inducible genes involved in fatty acid degradation included genes encoding enoyl-CoA hydratase, short chain dehydrogenases, aldehyde dehydrogenase, thioesterase, ABC transporters, MFS transporters, acyl-CoA dehydrogenase, and other β-oxidation enzymes. The transcriptional regulator AraC was detected near the alkane-1-monooxygenase gene on the chromosome. These fatty acid degradation enzymes were expressed even without hexadecane, which indicated that the expression background of these genes is high. This is probably the reason that the inducible value of most genes induced by hexadecane was relatively low. Normally, rubredoxin and rubredoxin reductase are needed for alkane degradation, together with alkane-1-monooxygenase ([Citation20] Park and Choi et al., 2020). Both the rubredoxin and rubredoxin reductase genes indeed exist in the Acinetobacter calcoaceticus Aca13 genome, but they were not detected by whole-transcriptome analysis in this study. Flavodoxin family proteins and iron-containing redox enzymes are also important for hexadecane degradation and were induced by hexadecane in this study.
There were 448 genes whose expression could be induced by naphthalene, and the log2-fold change with hexadecane was approximately 1–6. Fatty acid desaturase (gene ID: LRO55_RS06205) had the greatest log2-fold change among all the inducible genes. The log2-fold change in fatty acid desaturase activity was 6. According to the whole-transcriptome analysis results, most naphthalene-inducible genes were involved in microbial metabolism pathways in diverse environments, benzoate degradation, fatty acid degradation and aromatic compound degradation. The chemical structure of naphthalene has two aromatic rings. Aromatic ring-hydroxylating dioxygenase is the key enzyme for naphthalene degradation. Six dioxygenase genes could be induced by naphthalene, and their gene IDs are LRO55_RS12945 (aromatic ring-hydroxylating dioxygenase subunit alpha), LRO55_RS09405 (ring hydroxylating alpha subunit: catalytic domain), LRO55_RS09415 (dioxygenase electron transfer component), LRO55_RS13455 (aromatic ring-opening dioxygenase), LRO55_RS08095 (protocatechuate C4-dioxygenase subunit beta), and LRO55_RS08100 (protocatechuate C4-dioxygenase subunit alpha); detailed information is shown in .
For confirmation and validation of the RNA-seq data by qRT‒PCR, alkane-1-monooxygenase (LRO55_RS12655) was selected for hexadecane induction, and aromatic ring-hydroxylating dioxygenase subunit alpha (LRO55_RS12945) was selected for naphthalene induction. As shown in , alkane-1-monooxygenase could be significantly induced by hexadecane, and aromatic ring-hydroxylating dioxygenase could be significantly induced by naphthalene. The qRT‒PCR results were consistent with the transcriptome sequencing results.
Figure 3. Transcriptional levels of two upregulated genes in strain Aca13 determined by qRT‒PCR. The 16S rRNA gene was used as the reference gene. Hexadecane and naphthalene were added at the late logarithmic phase for induction. Samples without hexadecane and naphthalene were used as noninduced controls.
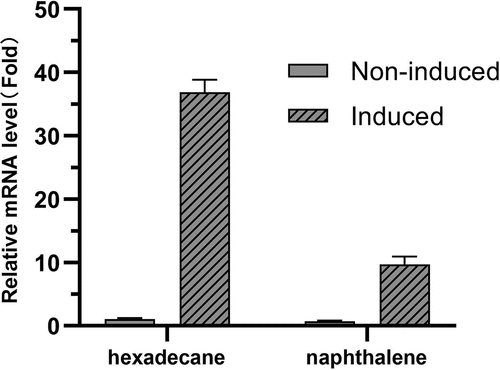
Proposed degradation pathways of hexadecane and naphthalene in strain Aca13 based on whole-genome and transcriptome analysis
There are four main pathways for n-alkane biodegradation: the terminal oxidation pathway, subterminal oxidation pathway, diterminal oxidation pathway, and Finnerty pathway [Citation21]. The ω-hydroxylase gene is lacking in the Acinetobacter calcoaceticus Aca13 genome. This indicates that Acinetobacter calcoaceticus Aca13 could not degrade alkanes via the diterminal oxidation pathway, as the critical point of the diterminal oxidation pathway is the existence of ω-hydroxylase. In the subterminal oxidation pathway, Baeyer-Villiger monooxygenases (BVMOs) are needed to convert the metabolic intermediate 2-hexadecanal into tetradecyl acetate for further oxidization by esterase to the corresponding alcohol. Two Baeyer-Villiger monooxygenase genes and many dioxygenase genes were found in the Acinetobacter calcoaceticus Aca13 genome, but they could not be induced by hexadecane in this study. Dioxygenases are needed for the subterminal oxidation pathway. Although many dioxygenases exist in the Aca13 genome, no dioxygenases can be induced by hexadecane. These genes may be responsible for the degradation of other alkanes or aromatic compounds but not for hexadecane degradation in strain Aca13. Overall, the terminal oxidation pathway was the main pathway for hexadecane degradation in strain Aca13 based on whole-genome and transcriptome analysis (). As shown in , hexadecane was first transformed into strain Aca13 by the ABC and MFS transporters. Three ABC transporters (RS09395, RS09010, and RS04855) and one MFS transporter (RS14640) were induced by hexadecane. Alkane 1-monooxygenase is the key enzyme for hexadecane degradation. Two alkane 1-monooxygenase genes (LRO55_RS12655 and LRO55_RS09975) are present in the Aca13 genome, and only LRO55_RS12655 could be significantly induced by hexadecane. Alcohol dehydrogenase, aldehyde dehydrogenase and acyl CoA synthase were reported to be subsequently involved in hexadecane degradation. The whole-transcriptome results showed that alcohol dehydrogenase and acyl CoA synthase could not be induced. Two short-chain dehydrogenases (RS18455 and RS11220) were upregulated by hexadecane. Since alcohol dehydrogenase belongs to the short chain dehydrogenase family, these two short chain dehydrogenases likely play a role in this process. Many enzymes involved in β-oxidation were also induced by hexadecane in this study (data not shown).
Figure 4. Proposed alkane degradation pathway in Acinetobacter calcoaceticus Aca13 based on whole-genome and transcriptome data. Pathways of alkane degradation from the literature were also considered. Numbers indicate the hexadecane-inducible gene IDs in the Aca13 genome. Red indicates key enzymes involved in alkane degradation [Citation21].
![Figure 4. Proposed alkane degradation pathway in Acinetobacter calcoaceticus Aca13 based on whole-genome and transcriptome data. Pathways of alkane degradation from the literature were also considered. Numbers indicate the hexadecane-inducible gene IDs in the Aca13 genome. Red indicates key enzymes involved in alkane degradation [Citation21].](/cms/asset/97b41cee-9907-47c9-90c7-2be2a40ac8a0/tcsb_a_2376008_f0004_oc.jpg)
According to whole-transcriptome analysis, naphthalene degradation in strain Aca13 starts with an initial deoxygenation reaction by an aromatic ring-hydroxylating dioxygenase (). The catalytic sites of aromatic ring-hydroxylating dioxygenases share a Rieske [2Fe-2S] nonheme iron catalytic center. Aromatic ring-hydroxylating dioxygenases have low substrate specificity for the oxidation of many aromatic compounds with different conversion rates. In strain Aca13, aromatic ring dioxygenase and aromatic ring-opening dioxygenase could be induced by naphthalene with a high log2-fold change (). Trans-o-hydroxybenzylidenepyruvate was generated after ring cleavage. The side chain of trans-o-hydroxybenzylidenepyruvate was then degraded by hydratase and aldehyde dehydrogenase. The expression of two protocatechuate dioxygenases was significantly induced by naphthalene. This result showed that naphthalene is further transformed into intermediates that enter catechol central metabolism after ring cleavage. Many TCA cycle enzymes can be induced by naphthalene, including short chain dehydrogenase, alcohol dehydrogenase, aldehyde dehydrogenase, thioesterase, and hydratase. This study contains the first report that anthranilate dioxygenase and anthranilate dioxygenase electron transfer components could be induced by naphthalene. The chemical structure of anthranilate is similar to that of trans-o-hydroxybenzylidenepyruvate, which is a very important metabolic intermediate of naphthalene. Anthranilate dioxygenase likely plays an important role in naphthalene degradation in strain Aca13. The function of anthranilate dioxygenase in naphthalene degradation needs further research.
Figure 5. Proposed naphthalene degradation pathway in Acinetobacter calcoaceticus Aca13 based on whole-genome and transcriptome data. Pathways of naphthalene degradation from the literature were also considered. Numbers indicate the naphthalene-inducible gene IDs in the Aca13 genome. Red indicates key enzymes involved in naphthalene degradation [Citation21].
![Figure 5. Proposed naphthalene degradation pathway in Acinetobacter calcoaceticus Aca13 based on whole-genome and transcriptome data. Pathways of naphthalene degradation from the literature were also considered. Numbers indicate the naphthalene-inducible gene IDs in the Aca13 genome. Red indicates key enzymes involved in naphthalene degradation [Citation21].](/cms/asset/1ca0e146-1ac0-40cd-9392-ec0cbcfa1dad/tcsb_a_2376008_f0005_oc.jpg)
In conclusion, Acinetobacter calcoaceticus Aca13, a newly isolated and characterized crude oil degradation strain from the Shengli Oil Field in China, exhibited a high capability for degrading n-alkanes and aromatic compounds. Whole-genome and transcriptome analysis revealed that the terminal oxidation pathway was the main pathway for hexadecane degradation and that central catechol metabolism was the main pathway for naphthalene degradation in strain Aca13. Among the upregulated genes, alkane 1-monooxygenase (gene ID: LRO55_RS12655) was the key enzyme for hexadecane degradation, and aromatic ring-hydroxylating dioxygenase (gene ID: LRO55_RS12945) and other aromatic ring-opening dioxygenases were key enzymes for naphthalene degradation. The present study contributes to the genome and transcriptome database of crude oil-degrading microorganisms and provides a new bacterium for the bioremediation of crude oil in Shengli Oil Field, China.
Ethics approval and consent to participate
This article does not contain any studies with human participants or animals performed by any of the authors.
Disclosure statement
No potential conflict of interest was reported by the author(s).
Additional information
Funding
References
- Vargas GC, Au WW, Izzotti A. Public health issues from crude-oil production in the Ecuadorian Amazon territories. Sci Total Environ. 2020;719:134647. doi: 10.1016/j.scitotenv.2019.134647
- Fuentes S, Patricia AVM, Seeger M. Bioremediation of petroleum hydrocarbons: catabolic genes, microbial communities, and applications. Appl Microbiol Biotechnol. 2014;98(11):4781–10. doi: 10.1007/s00253-014-5684-9
- Liu H, Xu J, Liang R, et al. Characterization of the medium- and long-chain n-Alkanes degrading Pseudomonas aeruginosa strain SJTD-1 and its alkane hydroxylase genes. PLOS ONE. 2014;9(8):e105506. doi: 10.1371/journal.pone.0105506
- Bourguignon N, Irazusta V, Isaac P, et al. Identification of proteins induced by polycyclic aromatic hydrocarbon and proposal of the phenanthrene catabolic pathway in Amycolatopsis tucumanensis DSM 45259. Ecotoxicol Environ Saf. 2019;175:19–28. doi: 10.1016/j.ecoenv.2019.02.071
- Ławniczak Ł, Woźniak-Karczewska M, Loibner AP, et al. Microbial degradation of hydrocarbons-basic principles for bioremediation: a review. Molecules. 2020;25(4):856. doi: 10.3390/molecules25040856
- Rojo F. Degradation of alkanes by bacteria. Environ Microbiol. 2009;11(10):2477–2490. doi: 10.1111/j.1462-2920.2009.01948.x
- Wegener G, Laso-Pérez R, Orphan VJ, et al. Anaerobic degradation of alkanes by marine Archaea. Annu Rev Microbiol. 2022;76(1):553–577. doi: 10.1146/annurev-micro-111021-045911
- Kim HS, Dong K, Kim J, et al. Characteristics of crude oil-degrading bacteria Gordonia iterans isolated from marine coastal in Taean sediment. Microbiology. 2019;8(6):e754. doi: 10.1002/mbo3.754
- Hao R, Lu A, Wang G. Crude-oil-degrading thermophilic bacterium isolated from an oil field. Can J Microbiol. 2004;50(3):175–182. doi: 10.1139/w03-116
- Van Beilen JB, Marin MM, Smits TH, et al. Characterization of two alkane hydroxylase genes from the marine hydrocarbonoclastic bacterium Alcanivorax borkumensis. Environ Microbiol. 2004;6(3):264–273. doi: 10.1111/j.1462-2920.2004.00567.x
- Liu N, Shi YE, Li J, et al. Isolation and characterization of a new highly effective 17β-estradiol-degrading Gordonia sp. strain R9. 3 Biotech. 2020;10(4):174. doi: 10.1007/s13205-020-2156-z
- Liu N, Maser E, Zhang T. Genomic analysis of Gordonia polyisoprenivorans strain R9, a highly effective 17 beta-estradiol and steroid-degrading bacterium. Chem Biol Interact. 2021;350:109685. doi: 10.1016/j.cbi.2021.109685
- Livak KJ, Schmittgen TD. Analysis of relative gene expression data using real-time quantitative PCR and the 2−ΔΔCt method. Methods. 2001;25(4):402–408. doi: 10.1006/meth.2001.1262
- Brenner DJ, Krieg NR, Staley JT. Part B (The Gammaproteobacteria), Volume two (the Proteobacteria), Bergey’s manual of systemtic bacteriology. Second (New York, USA: Springer) ed. 2009. p. 425–436.
- Yu T, Liu X, Ai J, et al. Microbial community succession during crude oil-degrading bacterial enrichment cultivation and construction of a degrading consortium. Front Microbiol. 2022;13:1044448. doi: 10.3389/fmicb.2022.1044448
- Williams SC, Austin RN. An overview of the electron-transfer proteins that activate alkane monooxygenase (AlkB). Front Microbiol. 2022;13:845551. doi: 10.3389/fmicb.2022.845551
- Yavas A, Icgen B. Diversity of the aromatic-ring-hydroxylating dioxygenases in the monoaromatic hydrocarbon degraders held by a common ancestor. Bull Environ Contam Toxicol. 2018;101(3):410–416. doi: 10.1007/s00128-018-2350-4
- Yesankar PJ, Patil A, Kapley A, et al. Catalytic resilience of multicomponent aromatic ring-hydroxylating dioxygenases in Pseudomonas for degradation of polycyclic aromatic hydrocarbons. World J Microbiol Biotechnol. 2023;39(7):166. doi: 10.1007/s11274-023-03617-0
- Park H, Choi I. Genomic and transcriptomic perspectives on mycoremediation of polycyclic aromatic hydrocarbons. Appl Microbiol Biotechnol. 2020;104(16):6919–6928. doi: 10.1007/s00253-020-10746-1
- Yuan K, Xie X, Wang X, et al. Transcriptional response of Mycobacterium sp. strain A1-PYR to multiple polycyclic aromatic hydrocarbon contaminations. Environ Pollut. 2018;243(Pt B):824–832. doi: 10.1016/j.envpol.2018.09.001
- Wang M, Ding M, Yuan Y. Bioengineering for the microbial degradation of petroleum hydrocarbon contaminants. Bioengineering. 2023;10(3):347. doi: 10.3390/bioengineering10030347