Abstract
Muscle-derived stem/progenitor cells (MDSPCs) have been demonstrated as a promising source of cellular therapy for the regeneration of peripheral nerves and how to improve the therapeutic potential of these cells is the focus of future research. This study aims to investigate the role of hypoxia on functional effects in MDSPCs. In this study, MDSPCs were isolated from skeletal muscles of limbs; meanwhile, the flow cytometry analysis, immunocytochemistry and multilineage differentiation were performed to characterize MDSPCs. We set cultured MDSPCs in a hypoxic condition with 3% oxygen. The quantitative RT–PCR results showed that hypoxia inhibits myogenic differentiation genes expression, while it is beneficial for the stemness and pro-angiogenic genes. The CCK-8, flow cytometry, Hoechst 33342 and trypan blue dye assays revealed that hypoxia promotes survival and reduces apoptotic cells from oxidative stress damage. The western blot analysis indicated increased expression of HIF-1α and its downstream proteins by hypoxia. Moreover, the angiogenesis assay showed that condition media extracted from hypoxia-treated MDSPCs support endothelial cells’ survival, migration and tube formation in vitro. The cultured cells under hypoxia appear as a salutary strategy to improve the survival and stemness of MDSPCs and enhance angiogenesis capacity in tissue injury repair and reconstruction applications.
Abbreviations: MDSPCs, Muscle-derived stem/progenitor cells; ESCs, embryonic stem cells; PPSCs, induced pluripotent stem cells; ACSs, adult stem cells; PNI, Peripheral nerve injuries; BMSCs, bone marrow mesenchymal stem cells; NSCs, neural stem cells; ADSCs, adipose-derived stem cells; HIF-1α; Hypoxia-inducible factor-1α ; VEGF, vascular endothelial growth factor; SDF-1, stromal-derived factor 1; PM, proliferation media; DMEM, Dulbecco’s modified eagle medium; FBS, fetal bovine serum; MYHC, Myosin heavy chain; CCK-8, cell counting kit-8; H2O2, hydrogen peroxide; CM, conditioned media; CON, control group; qPCR, Quantitative real-time polymerase chain reaction; MRL/MpJ, Murphy Roths Large
Introduction
With the development of stem/progenitor cell-based tissue engineering, cell regenerative therapy provides a promising prospect in tissue repair after various diseases and traumas in tissue injury. Stem/progenitor cells consist of many precursor populations, including embryonic stem cells (ESCs), induced pluripotent stem cells (PPSCs), adult stem cells (ACSs), which show strong self-renewal and pluripotent differentiation ability to become ideal regenerative medicine in tissue regeneration (Faroni et al. Citation2015; Ilic and Ogilvie Citation2017). Muscle-derived stem/progenitor cells (MDSPCs) are a population of adult stem/progenitor cells isolated from post-natal skeletal muscles with a greater self-renewal capacity and differentiation potential (Qu-Petersen et al. Citation2002). MDSPCs were shown to be capable of differentiating into polyhedral lineages in vitro and in vivo, such as muscular, adipogenic, osteogenic, chondrogenic, endothelial, hepatic, pancreatic and neural cells (Bellayr et al. Citation2010; Park et al. Citation2014; Kazuno et al. Citation2018; Wang et al. Citation2018b). MDSPCs can also cure multiple tissue lesions after transplant in vivo with adaptive immune responses through several recruitment mechanisms that revealed advantageous outcomes (Kwon et al. Citation2006). Although promising results are conducive to promote the potential application of MDSPCs in cell-based therapy, more efforts are still indispensable to improve the efficiency of MDSPCs therapy.
Peripheral nerve injuries (PNI) with severe regenerative microenvironments, such as ischemic, oxidative stress damage and inflammatory, often result in rock-ribbed sequelae (Santos Citation2000; Scheib and Höke Citation2013). In recent years, numerous studies applied MDSPCs to neuroregenerative engineering which could differentiate into neuronal, glial, and vascular phenotype and show some satisfactory repair effects (Alessandri et al. Citation2004; Lavasani et al. Citation2014). However, the fate of transplanted stem cells seems to be pessimistic. It is reported that only a small portion of MDSPCs could be detected in the interior of the regenerated tissues (Oshima et al. Citation2005), and the donor MDSPCs could be excluded within 2 weeks after transplantation into the severe injured peripheral nerves (Tamaki Citation2017). Further study indicates that the cross-talk between donor stem cells and host cells maybe the primary determinant during repairing the damaged tissues (Gharaibeh et al. Citation2011). Accordingly, the stem cells’ therapeutic effect was largely determined by cells’ paracrine function and engraftment efficiency after transplantation in vivo. Interestingly, hypoxia is one of the most hopeful stratagems to promote donor cells’ survival and secretion function and strengthen the regeneration capacity in rigorous ischemic environments (Zhang et al. Citation2019).
Changing the original culture environment of stem cells and even set them to a sub-lethal inducement arises the previous activation of survival mechanisms of these cells. As opposed to genetic modification and pharmacological preconditioning, hypoxia is another simplified, safe and reliable way in which stem cells can be accepted (Zhilai et al. Citation2016). This strategy has been designed to stimulate stem cells express salutary factors to enhance the stemness, proliferation, survival, migration and angiogenesis, which are essential for the success of cell therapies (Choi et al. Citation2014; Wan Safwani et al. Citation2017). Although long-term austere hypoxia may initiate cell death, exposure to short-lived hypoxia has been proven to confer cytoprotective profits, which have been reported in various kinds of ACSs, such as bone marrow mesenchymal stem cells (BMSCs) (Ho et al. Citation2018), neural stem cells (NSCs) (Zhang et al. Citation2017), adipose-derived stem cells (ADSCs) (Han et al. Citation2019). In addition, hypoxia could mainly utilize stabilization and accumulation of hypoxia-inducible factor-1α (HIF-1α) to realize the defensive effects; meanwhile, this special factor activates its downstream signaling mediators participated in protecting donor cells from further damage, such as vascular endothelial growth factor (VEGF) and stromal-derived factor 1 (SDF-1) (Bernhardt et al. Citation2007; Calinescu et al. Citation2017). When under hypoxia, stem cells raise expression and secretion of these factors, which have acknowledged angiogenesis effects and are quite crucial for tissue regeneration and therapeutic effect (Haider and Ashraf Citation2010). What’s more, MDSPCs would increase VEGF secretion by responding to hypoxia and the mechanical stretch in an ischemic environment (Payne et al. Citation2007). This evidence indicates the probability of using hypoxic culture condition to develop the normoxic culture method for MDSPCs before transplantation.
As an easily reachable source of pluripotent cells, MDSPCs showed impressive regenerative ability with less restriction by age. During durable expansion in vitro, MDSPCs can maintain a high proliferation rate with little indication of replicative senescence but the loss of partial stemness with asymmetric division and commit to myogenesis (Deasy et al. Citation2005). Hypoxia is a critical factor for maintaining the stemness and undifferentiated quiescent state of stem cells from different sources (Werle et al. Citation2019). The effects on their pluripotency and quiescent state seem to be associated with a cellular adaption response to hypoxia, which includes the stabilization of HIF-1α and the transcription of several genes that regulate these cellular characteristics (Haque et al. Citation2013). Maintenance of the stemness and less differentiated state of MDSPCs before transplanting is quite important for their engraftment efficiency (Li et al. Citation2013). According to the modified preplate technique we have successfully isolated MDSPCs from murine skeletal muscle and treated complex tissue defects using MDSPCs with autologous fat (Ma et al. Citation2012). To further increase the therapeutic effects after transplantation, methods should enhance the survival, function and engraftment efficiency of the transplanted MDSPCs. Several strategies have been devised to enhance the therapeutic efficiency of MDSPCs, such as genetic modify, laser exposure and tissue engineering material (Wu et al. Citation2010). However, whether hypoxia could bring positive effects of cultured MDSPCs has not yet been well described. In this preliminary study, we investigate the effects of hypoxia on stemness, proliferation, survival and angiogenic capacity of cultured MDSPCs in vitro, and aim to develop a proper strategy to further increase the therapeutic potential of MDSPCs.
Materials and methods
Cell isolation and culture
Muscle-derived stem/progenitor cells were isolated from the hind-limb skeletal muscles of newborn C57BL/6 mice, using a modified preplate technique, as previously described (Gharaibeh et al. Citation2008; Ma et al. Citation2012). In short, repurified and fragmented tissue samples were enzymatically digested using collagenase, dispase and trypsin. After percolation through 70 µm sieve filtration and pelleting, the cells were suspended in proliferation media (PM), containing high glucose Dulbecco’s modified eagle medium (DMEM) (Invitrogen, NY, Grand Island, USA), 10% fetal bovine serum (FBS) (Invitrogen, USA), 10% heat inactivated horse serum (Hyclone, Logan, UT), 0.5% chick embryo extract (Gemini, Australia) and 1% penicillin/streptomycin (Invitrogen, USA). Cells were cultured onto collagen-coated flasks at 37°C in a humidified 5% CO2 incubator and preplating for 6 days. Afterward, the slow adhering cells contained MDSPCs cultured in PM for expansion. When MDSPCs reached 70–80% confluence, the cells dissociated with Trypsin-EDTA (Invitrogen, USA) for subculture. The cells used in further experiments were expanded less than 25 passages. The hypoxia treatment involved three gases containing 3% O2, 5% CO2, and balanced N2 incubator at 37°C. The normoxia treatment was performed by a regular incubator at 37°C. MDSPCs were cultured with those two conditions for 12–24 h depending on the different experimental process. The umbilical vein endothelial cell line EA. hy926 (Cell Bank, Chinese Academy of Sciences) was cultured in DMEM, containing 10% FBS and 1% penicillin/streptomycin at humidified 37°C with 5% CO2 incubator. Cells were passaged by Trypsin-EDTA when 90% confluence was reached.
Characterization of MDSPCs by flow cytometry analyses
Cell surface markers of cultured MDSPCs were detected by flow cytometry methods. Cells harvest was performed by trypsin without EDTA and washed by PBS. Then, the 106 cells per suspension were incubated with specific antibody: FITC-conjugated anti-CD45, PE-conjugated anti-CD90 (Elabscience, China) and PE-conjugated anti-CD34, FITC-conjugated anti-Sca-1 (Biolegend, USA) at 4°C for 30 min in the dark, after that the cells were subjected to BD FACScanto cytometer (Becton-Dickinson, San Jose, CA, USA) for analysis.
Myogenic, osteogenic and adipogenic differentiation assay
For myogenic differentiation, MDSPCs were cultured in 24-well plates (2.5 × 104 cells/cm2) with PM for 2 days. Then the PM was replaced by a myogenic differentiation medium containing DMEM, 2% FBS, 1% penicillin/streptomycin and culture for 4 more days (Kawakami et al. Citation2019). After morphological symbols of myotube were watched, a Myosin heavy chain (MYHC) immunofluorescence was taken. For osteogenic differentiation, MDSPCs were seeded in 6-well plates at a density of 2.5 × 105 cells per well. When cells reached 70% confluence, PM was removed and changed into osteogenic medium containing DMEM with 100 nM dexamethasone, 0.05 mM ascorbic acid-2-phosphate, 10 mM β-glycerolphosphate, 10% FBS and 1% penicillin/streptomycin (Wei et al. Citation2011). The medium was refreshed every 3 days. After 10 days of differentiation, the histological stain of differentiated cells was performed by Alizarin Red (Beyotime Biotechnology, Shanghai, China). For adipogenic differentiation, cells were seeded on 6-well plates at a density of 2.5 × 105 cells per well. After confluency was reached, the adipogenic medium, containing DMEM supplemented with 1 µM dexamethasone, 0.5 mM isobutylmethylxanthine, 0.2 mM indomethacin, 10 µg/ml insulin, 10% FBS and 1% penicillin/streptomycin, was replaced. After 3 days the differentiation medium was changed by the maintenance medium, containing 10 µg/ml insulin and 10% FBS in DMEM, for 2 days (Choi et al. Citation2012). Alternating culture conditions with those two mediums were repeated two times when the lipid droplets appeared among the cells; histological stain was performed by Oil Red O (Solarbio Life Sciences, Beijing, China). All photographs were taken by an inverted fluorescent microscope (Nikon, Tokyo, Japan).
Immunofluorescence
Cells were fixed in 4% paraformaldehyde and underwent permeabilization with 0.5% Triton X-100 for 10 min at room temperature, blocked with 5% goat serum at 37°C for 30 min and incubated overnight at 4°C with primary antibodies. After that, samples were incubated with the associated fluorescence secondary antibodies at room temperature for 1 h. At last, the nuclei were visualized by staining with DAPI (4’, 6’ diamidino-2-phenylindole, Beyotime, China) for 10 min at room temperature. The images were obtained by an inverted fluorescent microscope (Nikon, Tokyo, Japan). The primary antibodies used in this assay include mouse anti-Desmin (Abcam, USA), mouse anti-MYHC (ABclonal, China) and Cy3-conjugated IgG (ABclonal, China) used as fluorescence secondary antibody.
Cell apoptosis assays
We performed MDSPC apoptosis with hydrogen peroxide (H2O2). The effect of hypoxia on MDSPC survival was measured by a preconditioning method (Meng et al. Citation2018). In short, after being cultured under normoxia or hypoxia for 12 h, the cells were washed with PBS and replaced by DMEM supplemented with or without damaging dose H2O2 (350 µM) before continuously incubated for another 12 h. Cell apoptosis was measured by AnnexinV-FITC-propidium iodide (PI) double-stained flow cytometry (BD Biosciences, USA) and Hoechst 33342 stain (Solarbio, China). The flow cytometry was done according to the manufacturer’s instructions; cells were harvested with trypsin and resuspended in a binding buffer that achieved 1 × 105 cells per milliliter, and then the dye of Annexin V-FITC and PI were added, respectively. After incubated at 4°C for 30 min in the dark, the cells were analyzed by the BD FACScanto cytometer, the apoptotic cells, including early apoptotic cells (annexin V-FITC +/PI−) and late apoptotic cells (annexin V-FITC +/PI +), were counted. In Hoechst 33342 stain assay, MDSPCs were washed in PBS twice, stained with Hoechst 33342 for 15 min at 37°C, and then the images of cell nuclei morphological changes were obtained by an inverted fluorescence microscope (Nikon, Tokyo, Japan). The cell nuclei were blue and apoptotic cells’ chromatin showed a strong blue fluorescence.
Cell viability assays
The cell viability was measured by a cell counting kit-8 (CCK-8) assay kit (Beyotime, China) and trypan blue dye (Beyotime, China) according to the manufacturer’s instructions. MDSPCs were seeded into 96-well plates (20,000 cells/cm2) and cultured with different conditions. In the CCK-8 assay, the CCK-8 reagent was added into each well before incubated for 1 h at 37°C and used a micro-plate reader to exam absorbance under 450 nm wavelengths at different time points. In the trypan blue dye assay, cells were digested by trypsin and stained with 0.1% trypan blue dye for 5 min at room temperature. A hemacytometer was used to count cell suspension and analysis for the ratio of living cells.
Quantitative real-time polymerase chain reaction (qPCR)
The total RNA was isolated from cells by using Trizol (Invitrogen, USA) according to the manufacturer’s instructions. RNA concentration was determined spectrophotometrically from 1.8 to 2.1 with a purity of an A260/A280 ratio. cDNA was synthesized with reverse transcription by using RevertAid First Strand cDNA Synthesis Kit (Life Technologies, Grand Island, NY, USA), Then, qPCR was done on the ABI 7300 Fast Real-Time PCR System with Maxima SYBR Green/ROX qPCR Master Mix Kit (Life Technologies, USA). The primer sequences used for mRNA expression analysis by qPCR are shown in Table . GAPDH served as an internal reference. Target genes’ expression changes were calculated by the 2−ΔΔCT method.
Table 1. Sequences of primers used for qPCR.
Western blot
Protein of MDSPCs was extracted lysing with ice-cold RIPA lysis buffer (Beyotime), the isolated proteins were quantified with a BCA Protein Assay Reagent Kit (Beyotime) after centrifuged at 12,000g at 4°C for 10 min. The protein assay was carried out separately by SDS-PAGE electrophoresis and transferred to PVDF membranes. After blocked in 5% skim milk, the membranes were incubated with primary antibodies against HIF-1α, SDF-1, VEGF and GAPDH (ABclonal, China) overnight at 4°C and then probed with connected secondary antibodies conjugated with horseradish peroxidase for 1 h. GAPDH served as an endogenous control. The ECL chemiluminescence detection system was used to visualize the protein bands.
Angiogenic ability of MDSPCs
The conditioned media (CM) was extracted from MDSPCs cultured under hypoxia (H-CM) or normoxia (N-CM) with serum-free media for 24 h and centrifuged at 300 g for 5 min to remove floating cells and debris (Stubbs et al. Citation2012; Proto et al. Citation2015). The endothelial cells co-cultured with uncultivated serum-free media served as a control group (CON) in the following tests. For survival tests, endothelial cells were seeded into 96-well plates (25,000 cells/cm2) and cultured with N-CM, H-CM or serum-free media for 48 h. The viability of cells was measured by a micro-plate reader at 450 nm with the CCK-8 assay. For migration tests, scratch migration assay was performed when endothelial cell grew to 80–90% confluence in 6-well plates. The line-shaped wound across scratches of cell monolayers was made by a standard sterile pipette tip (Axygen, Inc., USA). The cells were then cultured with N-CM, H-CM or serum-free media. Photographs were taken by a inverted microscope at 0 and 18 h. The migration was quantified by the ratio of closure area to initial wound area by the ImageJ software (NIH, USA). For test in vitro capillary-like tube formation ability, the endothelial cells (2 × 105 cells/ml) were seeded onto a 96-well plate precoated with Matrigel (Corning, USA) and cultured in N-CM, H-CM or serum-free media at 100 µL/well. After 8 h co-culture, tube formation was captured by the inverted fluorescent microscope. The number of segment tube lengths was counted by using the ImageJ software (NIH, USA).
Statistical analysis
The experimental data were analyzed by the Graphpad Prism 8.0 software (GraphPad Software, Inc., USA). Data are presented as mean ± standard deviation from at least three independent experiments. Comparisons between two groups were measured using unpaired Student’s two-tailed t-test, and differences among multiple groups were detected by one-way analysis followed with Tukey’s test. A P value of less than 0.05 was considered statistically significant.
Results
Identification of MDSPCs
An identifying characteristic of MDSPCs is the long-term proliferation ability. In the current cultivation condition as report previously, MDSPCs could be expanded for more than 20 passages without losing their self-renewal and differentiation capabilities (Deasy et al. Citation2005; Yang et al. Citation2012). The flow cytometry results showed that the expanded cells expressed Sca-1, CD34, CD90 (90.6%, 98.6%, 89.2%, respectively), but not CD45 (0.4%) (Figure (A)). In addition, the immunocytochemistry of Desmin, a muscle precursor antigen, showed that most of the isolated cells were positive for Desmin (Figure (B)). MYHC is a terminal myogenic differentiation marker which was used to detect differentiated myotube (Song et al. Citation2013). After cultured in myogenic differentiation medium for 4 days, isolated MDSPCs could differentiate into myotubular structures expressing MYHC (Figure (C,D)). Furthermore, after treatment with osteogenic and adipogenic differentiation media for 10 days, these cells could be differentiated into osteoblasts and adipocytes, as evidenced by Alizarin Red staining of mineralized nodules and Oil Red O staining of fatty droplets, respectively (Figure (E,F)). These results indicate these passaged MDSPCs have multilineage potential in vitro. On the other hand, we found that some of the extracted cells expanded beyond 30 passages spontaneously to form islet-like cell clusters with over 10-day cultivation (Figure (G)). These clusters may have a particular ability of MDSPCs to grow into islet-like formed colony during long-term cultivation (Mitutsova et al. Citation2017).
Figure 1. Characterization of MDSPCs. (A) Flow cytometry analysis for the detection of MDSPCs’ surface markers. (B) Cellular immunofluorescence image of Desmin in MDSPCs, cells nuclei were counterstained with DAPI. (C, D) Myogenic differentiation potential of MDSPCs. Brightfield image of myotube structures (C). Cellular immunofluorescence image of MYHC and nuclei counterstained with DAPI (D). (E, F) Osteogenic and adipogenic differentiation potential of MDSPCs, typical mineralization nodules and lipid droplets stained with Alizarin Red and Oil Red O, respectively. (G) Phase-contrast images of small and large islet-like living clusters spontaneously formed in long-term cultured MDSPCs. Bars, 100 µm .
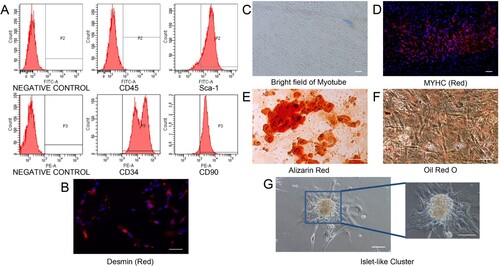
Effects of hypoxia on stemness and myogenic gene expression of MDSPCs
We performed a qPCR assay to measure the gene expression levels of stemness and myogenic transcription factors in normoxic or hypoxic cultured MDSPCs at 12 and 24 h. Our data showed that the stemness-related gene expression levels of Sox2 and Nanog increased after hypoxia for 24 h, but not Oct4, compared with cells cultured in normoxic condition (Figure (A)). On the other hand, we observed a statistically significant downregulation of two genes associated with myogenic differentiation in hypoxia for 24 h, including MYOG and MYOD (Figure (B)). The slightly decreased gene expression levels of MYF5 were observed but showed no statistical significance (Figure (B)). These data indicate that hypoxia negatively regulates genes that are related to MDSPC myogenesis, while positively regulating genes that are related to MDSPCs’ stemness.
Figure 2. The effect of hypoxia on MDSPCs’ stemness markers and myogenic transcription factors’ genes expression. The qPCR analysis of genes expression of stemness markers (A) and myogenic transcription factors (B) under normoxia or hypoxia for 12 and 24 h (n = 3 per group). Data are represented as mean ± standard deviation. *p < 0.05 versus the normoxia group.
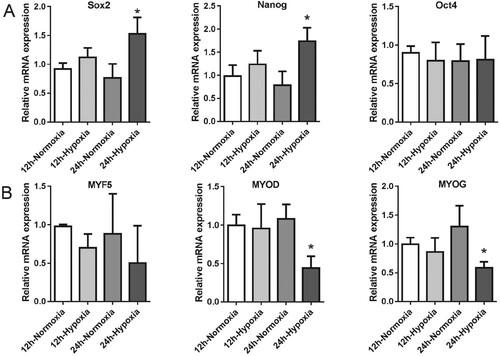
Effects of hypoxia on the vitality and survival of MDSPCs
We first tested the vitality changes in cultured MDSPCs under hypoxia for 6–24 h by the CCK-8 assay. As shown in Figure (A,B), the cell vitality cultured in 3% O2 with or without serum for 6, 12 and 24 h was not statistically significant from normoxic culture. The survival of cells is comparatively assessed with CCK-8, Annexin V-FITC/PI flow cytometry, Hoechst 33342 stain and trypan blue dyeing to observe the apoptosis of the cells in 350 µM H2O2 with or without hypoxic preconditioning. The results show that the exposed H2O2 in normoxic cultured cells increased the ratio of apoptosis cells (Figure (C–F)), while the cell survival rate decreased markedly (Figure (G,H)), and hypoxic preconditioning upgraded MDSPCs’ viability and mitigated MDSPCs’ apoptosis against H2O2 compared with normoxic culture (Figure (C–H)). Our results indicate that hypoxia preconditioning improves MDSPCs’ survival under an extremely harsh environment by reducing the apoptosis of cells.
Figure 3. The effect of hypoxia on MDSPCs’ vitality and survival. (A,B) The effect of hypoxia on MDSPCs’ vitality was tested by the CCK-8 assay compared to normoxia with (A) or without (B) serum at 6, 12 and 24 h (n = 6 per group). (C–F) The effect of hypoxic preconditioning on H2O2-induced MDSPCs’ apoptosis by the Hoechst 33342 stained assay (C, D) (n = 3 per group) and the Annexin V-FITC/PI stained flow cytometry assay (E F) (n = 4 per group). (G) The viability of hypoxic preconditioned MDSPCs with H2O2 was tested by the CCK-8 assay (n = 4 per group). (H) The sketch image of trypan blue dye, small arrow demonstrates dead cell, while the big arrow indicates live cell on the hemacytometer. (I) The viability of hypoxic preconditioned MDSPCs with H2O2 was tested by trypan blue dye (n = 4 per group). Bars, 100 µm. Data are represented as mean ± standard deviation. *p < 0.05 versus absence H2O2 group. #p < 0.05 versus normoxia + H2O2 group.
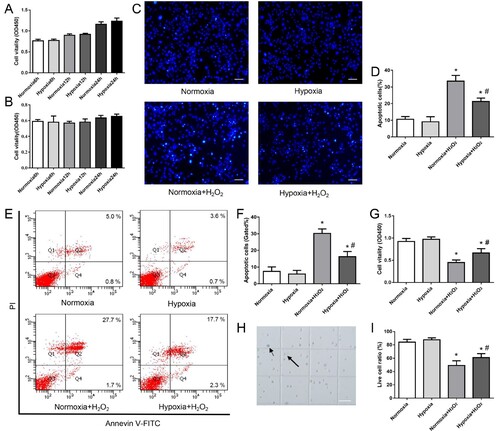
Effects of hypoxia on HIF-1α and angiogenic factors’ synthesis of MDSPCs
To further investigate the potential mechanisms may be accountable for the observed survival promotion of hypoxia in MDSPCs, we performed qPCR and Western blot to assess the level of several genes and protein expressions associated with cytoprotection and hypoxia. As a master factor for cellular response closely related to hypoxia, the protein expression level of HIF-1α with the hypoxia group was more elevated than that of the normoxic culture group (Figure (A)). The SDF-1 protein expression was highly up-regulated in MDSPCs following hypoxia for 12 h compared with normoxia, while the mRNA expression level had only a slight increase but not statistically significant in the hypoxia group (Figure (B,C)). It should be noted that mRNA and protein expression levels of VEGF enhanced at 12 and 24 h after being exposed to hypoxic culture condition compared with normoxia (Figure (D,E)). These results imply that HIF-1α signaling may be involved in the beneficial effects of hypoxia on MDSPCs.
Figure 4. The effect of hypoxia on HIF-1α protein and its downstream target expression of MDSPCs. (A) HIF-1α protein level was measured by western blot under normoxia or hypoxia for 12 and 24 h. (B–E) Gene and protein expression levels of SDF-1 (B, C) and VEGF (D–E) under normoxia or hypoxia for 12 and 24 h were assessed by qPCR and western blot. Data are represented as mean ± standard deviation (n = 3 per group). *p < 0.05 versus normoxia group.
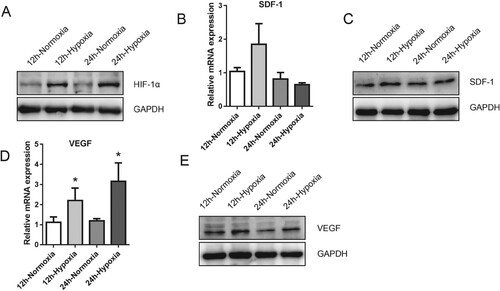
Effects of pro-angiogenic properties of hypoxia MDSPCs
To explore the paracrine effects of angiogenesis from different cultured MDSPCs, we further tested the effects of CM extracted from MDSPCs treated with or without hypoxia on the functions of endothelial cells in vitro. The migration and vitality of endothelial cells was assessed by the scratch migration assay and the CCK-8 assay respectively, and the results showed that the migration and vitality of endothelial cells cultured in CM from hypoxia-cultured MDSPCs had more effect than those cultured in CM from normoxic condition (Figure (A–C)). The tube formation assay results showed that the CM, extracted from MDSPCs treated with hypoxia, had a more powerful effect on promoting the tube formation capacity of endothelial cells on Matrigel in vitro (Figure (D–F)). The results indicate that the paracrine mechanism may be related to the pro-angiogenic property of hypoxia MDSPCs in vitro.
Figure 5. The effect of hypoxia on MDSPCs’ angiogenic ability. (A) The viability of endothelial cells treated with different media was tested by the CCK-8 assay (n = 4 per group). (B, C) The migration capacity of endothelial cells treated with different media was measured by the scratch migration assay (n = 4 per group). (D–F) The angiogenic capacity of endothelial cells treated with different media was measured by the tube formation assay on Matrigel and the images were analyzed by the ImageJ software (n = 3 per group). Bars, 200 µm. Data are represented as mean ± standard deviation. *p < 0.05 or *p < 0.01 versus CON. CON, control; N-CM, condition media from normoxic cultured MDSPCs; H-CM, condition media from hypoxic cultured MDSPCs.
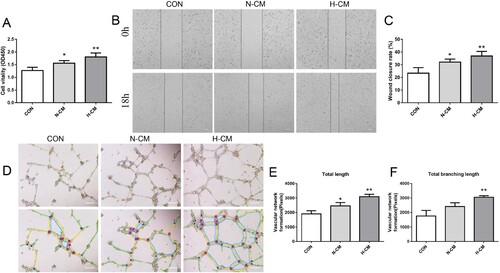
Discussion
Complex pathologic microenvironments are involved in PNI, which usually causes devastating and worrisome burden for the involvement of cytothesis. MDSPCs-based therapy is a possible new strategy to enhance neurodegenerative treatment. In the present study, MDSPCs were isolated from the skeletal muscles of limbs. Consistent with previous reports, our study confirmed that the most isolated cells express the MDSPCs’ associated markers (Sca-1, CD34, CD90 and desmin) and display multilineage differentiation ability in vitro (Wu et al. Citation2010). To further promote the clinical application of MDSPCs’ therapy, applied culture approaches in vitro should be established to overcome a range of issues accompanied with the following competitive transplantation in vivo, such as limited differentiation potency and ischemic injury or oxidative stress, caused low cell viability and grafting efficiency (Oshima et al. Citation2005). Techniques to enhance cell survival reply to injury by following transplantation, and techniques to improve cell functions, such as paracrine effects and pluripotency ability, have enlarged increasing attention in MDSPC therapy engineering (Gharaibeh et al. Citation2011). Among the various strategies that promote cultured stem cell survival and function, cell pretreatment by hypoxic cultivation has received special attention (Zhang et al. Citation2017).
The procedure of tissue repair relies on the functionality of adult stem/progenitor cells, which are maintained in hypoxic conditions and prepared for activation to participate in tissue regeneration. MDSPCs are considered a precursor cell of satellite and other myogenic cells in the skeletal muscle tissues (Wei et al. Citation2011). These myogenic stem cells are reportedly exposed to a low O2 (1–10%) environment in vivo with a mean level of 3% O2 (Li et al. Citation2012; Redshaw and Loughna Citation2012). A previous study demonstrated that the partial oxygen tension in skeletal muscle tissues was low (a 12% oxygen environment) during exhaustive exercise and promotes muscle growth (Hoppeler et al. Citation2003). For satellite cells, culturing at 6% oxygen concentration generates increased proliferation and survival (Csete et al. Citation2001); on the contrary, the fate of differentiation toward myotube decreased under extremely low oxygen (1–5%) (Sakushima et al. Citation2020). These findings suggest that the low oxygen tension may be a key factor in the regulation of stemness, differentiation, and survival in MDSPCs. Furthermore, a recent study maintained myoblast at different O2 tension for different time points exhibited diverse expression level of HIF-1α (Zimna et al. Citation2018). On the basis of hypoxia optimization results, in this study, we performed 3% O2 for MDSPCs’ treatment to acquire a higher expression of HIF-1α, while simulating a similar hypoxic condition in PNI and physiological O2 levels in skeletal muscles (McManis and Low Citation1988).
The sufficient quantity of quiescent and effective stem/progenitor cells in vitro culture is a major determinant for tissue reconstruction. The myogenic transcription factors (MYF5, MYOG and MYOD) and pluripotency-related genes (Sox2, Nanog, and Oct4) have been used to describe undifferentiated state and stemness of MDSPCs (Li et al. Citation2013; Rugowska et al. Citation2019). Hypoxia could maintain self-renewal and pluripotency capacity of stem cells most likely through regulation expression of these markers (Liu et al. Citation2012; Werle et al. Citation2019). Our data showed that this hypoxic condition is beneficial for increasing the gene expressions of Sox2 and Nanog, while decreasing the gene expressions of MYOG and MYOD in MDSPCs. These results are similar to other studies and indicate that hypoxia can maintain stemness and less differentiated state of cultured MDSPCs. A previous study indicated that after transplantation in an ischemic environment, the original undifferentiated state may contribute to myogenic stem cell survival and repair effects (Laumonier et al. Citation2017). However, whether hypoxia influences the multilineage differentiation ability of MDSPCs remained unclear, this is another study that we are working on.
Using the knowledge of mechanisms giving rise to cell viability during organ ischemic preconditioning, hypoxic culture on ex vivo stem cells has been described to promote these grafted cell proliferation, survival and become more impervious to death stimulation (Muscari et al. Citation2013). Our results of MDSPCs’ vitality with hypoxia suggest that short-term hypoxia culture in vitro may not promote MDSPCs’ proliferation; meanwhile, this culture condition probably does not cause the additional damage to MDSPCs. Thereby, we further investigated the effect of hypoxia on MDSPCs’ survival; the data showed that hypoxia promotes MDSPCs’ survival from the damage of H2O2 by attenuating apoptosis. In addition, we preliminarily evaluated the involved mechanisms, our results showed that HIF-1α protein in our hypoxic cultured model is up-regulated in MDSPCs, which are hardly detected under normoxic culture. HIF-1α is a key moderator by mediating the hypoxic response in cells. Under normoxia, HIF-1α can be rapidly degraded by ubiquitination and proteasome, which were the results of hydroxylate by HIF prolyl hydroxylases and conformational changes of the binding with von Hippel-Lindau protein were followed (Boulahbel et al. Citation2009). However, hypoxic conditions inhibit hydroxylation and degradation of HIF-1α and lead to its stable high expression in cells (Zagórska and Dulak Citation2004). One study showed that HIF-1α signaling plays a key role in maintaining stemness and preventing the differentiation of Pax7 positive muscle satellite cells (Jash and Adhya Citation2015). In addition, a research presented that HIF-1α-mediated autophagy in genioglossus muscle-derived stem cells played a cytoprotective role in the face of hypoxic stress (Wang et al. Citation2018a). A very recent study indicated that the stable expression of HIF-1α by hypoxia in MDSPCs from Murphy Roths Large (MRL/MpJ) mouse results in encouraging stemness of these cells through inhibition of the WNT pathway and differentiation factors (Sinha et al. Citation2019). Our present study provides initiatory evidence of hypoxia that promotes the survival and HIF-1α stabilization of MDSPCs, while suppressing myogenic gene expression and maintaining the stemness of MDSPCs, which is consistent with other studies and may suggest that HIF-1α plays a critical role in the survival and balance of myogenesis and stemness of MDSPCs.
Many research studies have demonstrated that hypoxia could regulate a series of endogenous regenerative factors involved in stem cell survival, apoptosis, and angiogenesis, which are the downstream molecules of HIF-1α signaling (Stubbs et al. Citation2012; Hao et al. Citation2019; Xu et al. Citation2019). Previous study showed that increased stabilization of HIF-1α simulates expression of genes involved in higher MRL/MpJ MDSPCs function and survival, includes inhibitor of differentiation 2, Insulin-Like Growth Factor 2 and NO synthase 2 (Sinha et al. Citation2019). A recent study reported that transduced to over express HIF-1α in rabbit muscle-derived stem cells could rise the expression levels of VEGF and SDF-1 and promote the functional regeneration of corpus cavernosa injury (An et al. Citation2020). Previous studies showed that exogenous VEGF promotes MDSPCs’ proliferation, migration and angiogenesis, as well as genetically engineered MDSPCs, to overexpression VEGF, have more powerful function in cardiac repair (Payne et al. Citation2007; Wang et al. Citation2017). What’s more, it has been demonstrated that SDF-1 and VEGF are key factors in peripheral nerve regeneration by recruiting repair cells to facilitate axonal growth and vascularization (Sheu et al. Citation2012). In our study, we examined VEGF and SDF-1 expressions in MDSPCs to test whether hypoxia could increase these HIF-1α downstream targets. The significantly increased expression of SDF-1 protein with a slight increase in mRNA level was observed in the first 12 h, while VEGF mRNA and protein level gradually up-regulated under hypoxia. Similar regulation of SDF-1 response to hypoxia accords with the conclusion that it seems that cells increase this factor to accommodate acute rather than chronic hypoxia and the degradation rates of mammalian mRNA transcript are faster than those of protein (Schneider et al. Citation2012; Vogel and Marcotte Citation2012).
Ideal peripheral nerve regeneration needs a vigorous vascular bed to support all cells with adequate nutrients and recruitment of repaired cells, the newly formed vascular connection network needs to be highly structured (Rouwkema and Khademhosseini Citation2016). Previous studies reported that hypoxia could enhance the paracrine function of mesenchymal stem cells and conditioned media improved endothelial cell survival, tube formation and promote their tissue repair effects (Hao et al. Citation2019; Jiang et al. Citation2019). Many new research studies have reported that hypoxia stimulates the levels of paracrine factors in the exosomes secreted by stem cells in the media which can commendably enhance the angiogenesis function of transplanted cells (Gonzalez-King et al. Citation2017; Han et al. Citation2019). Based on these studies, we performed CM extracted from MDSPCs to assess hypoxia on MDSPCs’ paracrine effects in endothelial cells’ function. Our results showed that hypoxia promotes the pro-angiogenic capacity of MDSPCs by keeping viability, increasing migration and angiogenesis of endothelial cells, suggesting that the paracrine effects of MDSPCs were improved by hypoxia. Therefore, transplanting hypoxia-cultured MDSPCs may be an ideal approach for enhancing stem cell-based regenerative effects.
Conclusion
Hypoxic culture condition improves survival and pro-angiogenic property and maintains the stemness of MDSPCs. The HIF-1α signaling seems to be involved in the alternate capacity and function of MDSPCs. Further in vitro or vivo experiments are necessary to verify the neural regeneration functions of hypoxic pretreated MDSPCs. However, this study tried to develop a practical approach to improve the efficiency of applying MDSPCs for tissue regeneration engineering.
Acknowledgments
All procedures involving the use of animals were approved by the Ethics Committee of the Second Affiliated Hospital of Harbin Medical University (NO. SYDW2019-108).
Data availability statement
The data that support the findings of the present study are available at the fig share repository (http://figshare.com/) at https://figshare.com/s/aa3b2eb462a6f9c8aa7d.
Disclosure statement
No potential conflict of interest was reported by the author(s).
Additional information
Funding
References
- Alessandri G, Pagano S, Bez A, Benetti A, Pozzi S, Iannolo G, Baronio M, Invernici G, Caruso A, Muneretto C, et al. 2004. Isolation and culture of human muscle-derived stem cells able to differentiate into myogenic and neurogenic cell lineages. Lancet. 364:1872–1883. Epub 2004/11/24.
- An G, Guo F, Liu X, Wang Z, Zhu Y, Fan Y, Xuan C, Li Y, Wu H, Shi X, et al. 2020. Functional reconstruction of injured corpus cavernosa using 3D-printed hydrogel scaffolds seeded with HIF-1α-expressing stem cells. Nat Commun. 11:2687. Epub 2020/06/03.
- Bellayr IH, Gharaibeh B, Huard J, Li Y. 2010. Skeletal muscle-derived stem cells differentiate into hepatocyte-like cells and aid in liver regeneration. Int J Clin Exp Pathol. 3:681–690. Epub 2010/09/11.
- Bernhardt WM, Warnecke C, Willam C, Tanaka T, Wiesener MS, Eckardt KU. 2007. Organ protection by hypoxia and hypoxia-inducible factors. Methods Enzymol. 435:221–245. Epub 2007/11/14.
- Boulahbel H, Durán RV, Gottlieb E. 2009. Prolyl hydroxylases as regulators of cell metabolism. Biochem Soc Trans. 37(Part 1):291–294. Epub 2009/01/16.
- Calinescu AA, Yadav VN, Carballo E, Kadiyala P, Tran D, Zamler DB, Doherty R, Srikanth M, Lowenstein PR, Castro MG. 2017. Survival and proliferation of neural progenitor-derived glioblastomas under hypoxic stress is controlled by a CXCL12/CXCR4 autocrine-positive feedback mechanism. Clin Cancer Res. 23:1250–1262. Epub 2016/08/21.
- Choi JR, Pingguan-Murphy B, Wan Abas WAB, Omar SZ, Chua KH, Mat Adenan NA, Wan Safwani WKZ. 2014. Hypoxia promotes growth and viability of human adipose-derived stem cells with increased growth factors secretion. J Asian Sci Res. 4:328–338.
- Choi YJ, Lee JY, Lee SJ, Chung CP, Park YJ. 2012. Determination of osteogenic or adipogenic lineages in muscle-derived stem cells (MDSCs) by a collagen-binding peptide (CBP) derived from bone sialoprotein (BSP). Biochem Biophys Res Commun. 419:326–332. Epub 2012/02/22.
- Csete M, Walikonis J, Slawny N, Wei Y, Korsnes S, Doyle JC, Wold B. 2001. Oxygen-mediated regulation of skeletal muscle satellite cell proliferation and adipogenesis in culture. J Cell Physiol. 189:189–196. Epub 2001/10/13.
- Deasy BM, Gharaibeh BM, Pollett JB, Jones MM, Lucas MA, Kanda Y, Huard J. 2005. Long-term self-renewal of postnatal muscle-derived stem cells. Mol Biol Cell. 16:3323–3333. Epub 2005/05/06.
- Faroni A, Mobasseri SA, Kingham PJ, Reid AJ. 2015. Peripheral nerve regeneration: experimental strategies and future perspectives. Adv Drug Deliv Rev. 82-83:160–167. Epub 2014/12/03.
- Gharaibeh B, Lavasani M, Cummins JH, Huard J. 2011. Terminal differentiation is not a major determinant for the success of stem cell therapy – cross-talk between muscle-derived stem cells and host cells. Stem Cell Res Ther. 2:31. Epub 2011/07/13.
- Gharaibeh B, Lu A, Tebbets J, Zheng B, Feduska J, Crisan M, Péault B, Cummins J, Huard J. 2008. Isolation of a slowly adhering cell fraction containing stem cells from murine skeletal muscle by the preplate technique. Nat Protoc. 3:1501–1509. Epub 2008/09/06.
- Gonzalez-King H, García NA, Ontoria-Oviedo I, Ciria M, Montero JA, Sepúlveda P. 2017. Hypoxia inducible factor-1α potentiates jagged 1-mediated angiogenesis by mesenchymal stem cell-derived exosomes. Stem Cells. 35:1747–1759. Epub 2017/04/05.
- Haider H, Ashraf M. 2010. Preconditioning and stem cell survival. J Cardiovasc Transl Res. 3:89–102. Epub 2010/06/19.
- Han Y, Ren J, Bai Y, Pei X, Han Y. 2019. Exosomes from hypoxia-treated human adipose-derived mesenchymal stem cells enhance angiogenesis through VEGF/VEGF-R. Int J Biochem Cell Biol. 109:59–68. Epub 2019/02/03.
- Hao D, He C, Ma B, Lankford L, Reynaga L, Farmer DL, Guo F, Wang A. 2019. Hypoxic preconditioning enhances survival and proangiogenic capacity of human first trimester chorionic villus-derived mesenchymal stem cells for fetal tissue engineering. Stem Cells Int. 2019:9695239. Epub 2019/11/30.
- Haque N, Rahman MT, Abu Kasim NH, Alabsi AM. 2013. Hypoxic culture conditions as a solution for mesenchymal stem cell based regenerative therapy. Sci World J. 2013:632972. Epub 2013/09/27.
- Ho SS, Hung BP, Heyrani N, Lee MA, Leach JK. 2018. Hypoxic preconditioning of mesenchymal stem cells with subsequent spheroid formation accelerates repair of segmental bone defects. Stem Cells. 36:1393–1403. Epub 2018/07/04.
- Hoppeler H, Vogt M, Weibel ER, Flück M. 2003. Response of skeletal muscle mitochondria to hypoxia. Exp Physiol. 88:109–119. Epub 2003/01/15.
- Ilic D, Ogilvie C. 2017. Concise review: human embryonic stem cells – what have we done? What are we doing? Where are we going? Stem Cells. 35:17–25. Epub 2016/06/29.
- Jash S, Adhya S. 2015. Effects of transient hypoxia versus prolonged hypoxia on satellite cell proliferation and differentiation in vivo. Stem Cells Int. 2015:961307. Epub 2015/03/20.
- Jiang RH, Wu CJ, Xu XQ, Lu SS, Zu QQ, Zhao LB, Wang J, Liu S, Shi HB. 2019. Hypoxic conditioned medium derived from bone marrow mesenchymal stromal cells protects against ischemic stroke in rats. J Cell Physiol. 234:1354–1368. Epub 2018/08/05.
- Kawakami Y, Hambright WS, Takayama K, Mu X, Lu A, Cummins JH, Matsumoto T, Yurube T, Kuroda R, Kurosaka M, et al. 2019. Rapamycin rescues age-related changes in muscle-derived stem/progenitor cells from progeroid mice. Mol Therapy Methods Clin Develop. 14:64–76. Epub 2019/07/18.
- Kazuno A, Maki D, Yamato I, Nakajima N, Seta H, Soeda S, Ozawa S, Uchiyama Y, Tamaki T. 2018. Regeneration of transected recurrent laryngeal nerve using hybrid-transplantation of skeletal muscle-derived stem cells and bioabsorbable scaffold. J Clin Med. 7:276–293. Epub 2018/09/15.
- Kwon D, Kim Y, Pruchnic R, Jankowski R, Usiene I, de Miguel F, Huard J, Chancellor MB. 2006. Periurethral cellular injection: comparison of muscle-derived progenitor cells and fibroblasts with regard to efficacy and tissue contractility in an animal model of stress urinary incontinence. Urology. 68:449–454. Epub 2006/08/15.
- Laumonier T, Bermont F, Hoffmeyer P, Kindler V, Menetrey J. 2017. Human myogenic reserve cells are quiescent stem cells that contribute to muscle regeneration after intramuscular transplantation in immunodeficient mice. Sci Rep. 7:3462. Epub 2017/06/16.
- Lavasani M, Thompson SD, Pollett JB, Usas A, Lu A, Stolz DB, Clark KA, Sun B, Péault B, Huard J. 2014. Human muscle-derived stem/progenitor cells promote functional murine peripheral nerve regeneration. J Clin Invest. 124:1745–1756. Epub 2014/03/20.
- Li H, Usas A, Poddar M, Chen CW, Thompson S, Ahani B, Cummins J, Lavasani M, Huard J. 2013. Platelet-rich plasma promotes the proliferation of human muscle derived progenitor cells and maintains their stemness. PLoS One. 8:e64923. Epub 2013/06/14.
- Li X, Wang X, Zhang P, Zhu L, Zhao T, Liu S, Wu Y, Chen X, Fan M. 2012. Extracellular signal-regulated kinase 1/2 mitogen-activated protein kinase pathway is involved in inhibition of myogenic differentiation of myoblasts by hypoxia. Exp Physiol. 97:257–264. Epub 2011/10/18.
- Liu W, Wen Y, Bi P, Lai X, Liu XS, Liu X, Kuang S. 2012. Hypoxia promotes satellite cell self-renewal and enhances the efficiency of myoblast transplantation. Development. 139:2857–2865. Epub 2012/07/06.
- Ma Z, Han D, Zhang P, Yang JF, Wang Y, Zhang Y, Yang D, Liu J. 2012. Utilizing muscle-derived stem cells to enhance long-term retention and aesthetic outcome of autologous fat grafting: pilot study in mice. Aesthetic Plast Surg. 36:186–192. Epub 2011/05/25.
- McManis PG, Low PA. 1988. Factors affecting the relative viability of centrifascicular and subperineurial axons in acute peripheral nerve ischemia. Exp Neurol. 99:84–95. Epub 1988/01/01.
- Meng SS, Xu XP, Chang W, Lu ZH, Huang LL, Xu JY, Liu L, Qiu HB, Yang Y, Guo FM. 2018. LincRNA-p21 promotes mesenchymal stem cell migration capacity and survival through hypoxic preconditioning. Stem Cell Res Ther. 9:280. Epub 2018/10/26.
- Mitutsova V, Yeo WWY, Davaze R, Franckhauser C, Hani EH, Abdullah S, Mollard P, Schaeffer M, Fernandez A, Lamb NJC. 2017. Adult muscle-derived stem cells engraft and differentiate into insulin-expressing cells in pancreatic islets of diabetic mice. Stem Cell Res Ther. 8:86. Epub 2017/04/20.
- Muscari C, Giordano E, Bonafè F, Govoni M, Pasini A, Guarnieri C. 2013. Priming adult stem cells by hypoxic pretreatments for applications in regenerative medicine. J Biomed Sci. 20:63. Epub 2013/08/30.
- Oshima H, Payne TR, Urish KL, Sakai T, Ling Y, Gharaibeh B, Tobita K, Keller BB, Cummins JH, Huard J. 2005. Differential myocardial infarct repair with muscle stem cells compared to myoblasts. Mol Therapy. 12:1130–1141. Epub 2005/08/30.
- Park HS, Choi GH, Hahn S, Yoo YS, Jung IM, Lee T. 2014. Tracking the fate of muscle-derived stem cells: an insight into the distribution and mode of action. Vasc Specialist Int. 30:11–18. Epub 2014/03/01.
- Payne TR, Oshima H, Okada M, Momoi N, Tobita K, Keller BB, Peng H, Huard J. 2007. A relationship between vascular endothelial growth factor, angiogenesis, and cardiac repair after muscle stem cell transplantation into ischemic hearts. J Am Coll Cardiol. 50:1677–1684. Epub 2007/10/24.
- Proto JD, Tang Y, Lu A, Chen WC, Stahl E, Poddar M, Beckman SA, Robbins PD, Nidernhofer LJ, Imbrogno K, et al. 2015. NF-κB inhibition reveals a novel role for HGF during skeletal muscle repair. Cell Death Dis. 6:e1730. Epub 2015/04/24.
- Qu-Petersen Z, Deasy B, Jankowski R, Ikezawa M, Cummins J, Pruchnic R, Mytinger J, Cao B, Gates C, Wernig A, et al. 2002. Identification of a novel population of muscle stem cells in mice: potential for muscle regeneration. J Cell Biol. 157:851–864. Epub 2002/05/22.
- Redshaw Z, Loughna PT. 2012. Oxygen concentration modulates the differentiation of muscle stem cells toward myogenic and adipogenic fates. Differentiation. 84:193–202. Epub 2012/07/14.
- Rouwkema J, Khademhosseini A. 2016. Vascularization and angiogenesis in tissue engineering: beyond creating static networks. Trends Biotechnol. 34:733–745. Epub 2016/04/02.
- Rugowska A, Wiernicki B, Maczewski M, Mackiewicz U, Chojnacka K, Bednarek-Rajewska K, Kluk A, Majewski P, Kolanowski T, Malcher A, et al. 2019. Human skeletal muscle-derived stem/progenitor cells modified with connexin-43 prevent arrhythmia in rat post-infarction hearts and influence gene expression in the myocardium. J Physiol Pharmacol. 70:917–933. Epub 2020/03/24.
- Sakushima K, Yoshikawa M, Osaki T, Miyamoto N, Hashimoto T. 2020. Moderate hypoxia promotes skeletal muscle cell growth and hypertrophy in C2C12 cells. Biochem Biophys Res Commun. 525:921–927. Epub 2020/03/17.
- Santos PM. 2000. A functional model system of an hypoxic nerve injury and its evaluation. Laryngoscope. 110:845–853. Epub 2000/05/12.
- Scheib J, Höke A. 2013. Advances in peripheral nerve regeneration. Nat Rev Neurol. 9:668–676. Epub 2013/11/13.
- Schneider C, Krischke G, Rascher W, Gassmann M, Trollmann R. 2012. Systemic hypoxia differentially affects neurogenesis during early mouse brain maturation. Brain Dev. 4:261–273. Epub 2011/08/10.
- Sheu ML, Cheng FC, Su HL, Chen YJ, Chen CJ, Chiang CM, Chiu WT, Sheehan J, Pan HC. 2012. Recruitment by SDF-1α of CD34-positive cells involved in sciatic nerve regeneration. J Neurosurg. 116:432–444. Epub 2011/08/23.
- Sinha KM, Tseng C, Guo P, Lu A, Pan H, Gao X, Andrews R, Eltzschig H, Huard J. 2019. Hypoxia-inducible factor 1α (HIF-1α) is a major determinant in the enhanced function of muscle-derived progenitors from MRL/MpJ mice. FASEB J. 33:8321–8334. Epub 2019/04/11.
- Song M, Lavasani M, Thompson SD, Lu A, Ahani B, Huard J. 2013. Muscle-derived stem/progenitor cell dysfunction in Zmpste24-deficient progeroid mice limits muscle regeneration. Stem Cell Res Ther. 4:33. Epub 2013/03/28.
- Stubbs SL, Hsiao ST, Peshavariya HM, Lim SY, Dusting GJ, Dilley RJ. 2012. Hypoxic preconditioning enhances survival of human adipose-derived stem cells and conditions endothelial cells in vitro. Stem Cells Dev. 21:1887–1896. Epub 2011/12/15.
- Tamaki T. 2017. Therapeutic capacities of human and mouse skeletal muscle-derived stem cells for a long gap peripheral nerve injury. Neural Regen Res. 12:1811–1813. Epub 2017/12/15.
- Vogel C, Marcotte EM. 2012. Insights into the regulation of protein abundance from proteomic and transcriptomic analyses. Nat Rev Genet. 13:227–232. Epub 2012/03/14.
- Wang H, Zhang D, Jia S, Huang S, Xiao L, Ma L, Liu G, Gong K, Xu L. 2018a. Effect of sustained hypoxia on autophagy of genioglossus muscle-derived stem cells. Med Sci Monit. 24:2218–2224. Epub 2018/04/14.
- Wang HD, Guo Q, Quan A, Lopez J, Alonso-Escalante JC, Lough DM, Lee WPA, Brandacher G, Kumar AR. 2017. Vascular endothelial growth factor induction of muscle-derived stem cells enhances vascular phenotype while preserving myogenic potential. Ann Plast Surg. 79:404–409. Epub 2017/06/02.
- Wang J, Zhang F, Yang H, Wu H, Cui R, Zhao Y, Jiao C, Wang X, Liu X, Wu L, et al. 2018b. Effect of TEAD4 on multilineage differentiation of muscle-derived stem cells. Am J Transl Res. 10:998–1011. Epub 2018/04/11.
- Wan Safwani WKZ, Choi JR, Yong KW, Ting I, Mat Adenan NA, Pingguan-Murphy B. 2017. Hypoxia enhances the viability, growth and chondrogenic potential of cryopreserved human adipose-derived stem cells. Cryobiology. 75:91–99. Epub 2017/01/22.
- Wei Y, Li Y, Chen C, Stoelzel K, Kaufmann AM, Albers AE. 2011. Human skeletal muscle-derived stem cells retain stem cell properties after expansion in myosphere culture. Exp Cell Res. 317:1016–1027. Epub 2011/02/01.
- Werle SB, Chagastelles P, Pranke P, Casagrande L. 2019. Hypoxia upregulates the expression of the pluripotency markers in the stem cells from human deciduous teeth. Clin Oral Investig. 23:199–207. Epub 2018/04/08.
- Wu X, Wang S, Chen B, An X. 2010. Muscle-derived stem cells: isolation, characterization, differentiation, and application in cell and gene therapy. Cell Tissue Res. 340:549–567. Epub 2010/05/25.
- Xu W, Xu R, Li Z, Wang Y, Hu R. 2019. Hypoxia changes chemotaxis behaviour of mesenchymal stem cells via HIF-1α signalling. J Cell Mol Med. 23:1899–1907. Epub 2019/01/11.
- Yang J, Wang X, Wang Y, Guo ZX, Luo DZ, Jia J, Wang XM. 2012. Dopaminergic neuronal conversion from adult rat skeletal muscle-derived stem cells in vitro. Neurochem Res. 37:1982–1992. Epub 2012/06/23.
- Zagórska A, Dulak J. 2004. HIF-1: the knowns and unknowns of hypoxia sensing. Acta Biochim Pol. 51:563–585. Epub 2004/09/28.
- Zhang T, Yang X, Liu T, Shao J, Fu N, Yan A, Geng K, Xia W. 2017. Adjudin-preconditioned neural stem cells enhance neuroprotection after ischemia reperfusion in mice. Stem Cell Res Ther. 8:248. Epub 2017/11/09.
- Zhang Y, Ma L, Su Y, Su L, Lan X, Wu D, Han S, Li J, Kvederis L, Corey S, et al. 2019. Hypoxia conditioning enhances neuroprotective effects of aged human bone marrow mesenchymal stem cell-derived conditioned medium against cerebral ischemia in vitro. Brain Res. 1725:146432. Epub 2019/09/07.
- Zhilai Z, Biling M, Sujun Q, Chao D, Benchao S, Shuai H, Shun Y, Hui Z. 2016. Preconditioning in lowered oxygen enhances the therapeutic potential of human umbilical mesenchymal stem cells in a rat model of spinal cord injury. Brain Res. 1642:426–435. Epub 2016/04/18.
- Zimna A, Wiernicki B, Kolanowski T, Malcher A, Rozwadowska N, Labedz W, Kubaszewski L, Kurpisz M. 2018. Influence of hypoxia prevailing in post-infarction heart on proangiogenic gene expression and biological features of human myoblast cells applied as a pro-regenerative therapeutic tool. J Physiol Pharmacol. 69:859–874. Epub 2019/03/23.