Abstract
Alpinia zerumbet is a medicinal and ornamental plant of the Zingiberaceae family cultivated in many parts of the world. The plant is medicinally important and often misidentified with closely related species. The objective of this work was to establish its DNA-based identity and a protocol for high-fidelity micropropagation to support the medicinal plant industry. PCR amplification and subsequent sequencing of RuBisCO large subunit (rbcL) and Maturase K (matK) gene successfully report its DNA barcode. In vitro shoots were induced the best from rhizome buds on Murashige and Skoog (MS) medium supplemented with 6.0 mg L−1 6-benzyl amino purine and 30 g L−1 sucrose. Microshoots were rooted well in MS medium amended with 1.5 mg L−1 naphthalene acetic acid. Plantlets were satisfactorily acclimated to soils maintained in a growth chamber and subsequently in the open air for 10–20 days. Analyzing eight random amplified polymorphic DNA and eight inter-simple sequence repeat primers resulted in 53 and 60 distinct bands, respectively. Uniform banding patterns suggest the genetic uniformity of the micropropagated plants. The results offer an accurate identification of A. zerumbet from any plant tissue. The micropropagation protocol would be helpful for economically feasible and environmentally sustainable exploitation of this valuable plant.
Introduction
Alpinia zerumbet (Pers.) B. L. Burtt & R. M. Sm., belongs to the family Zingiberaceae, (Xuan et al. Citation2019) is native to East Asia, including Bangladesh, Northeastern India, Myanmar, China, and Japan. The plant has a leafy stem up to 3 m tall, inflorescences are de-curved or drooping, up to 20 cm long, and bear 25 or more cincinni of two flowers each. A. zerumbet is commercially grown in the United States, Europe, South East Asia, and many other tropical and subtropical nations for ornamental purposes. The plant is propagated by rhizome division and seeds and is a potential invader in favorable environments in some countries.
For centuries, the plant has become part of human food and medicine across the globe. Traditional use includes the preparation of rice dishes in Japan (Victório Citation2011), and infusates in Brazil for antihypertensive and diuretic properties (Chan et al. Citation2010). Scientific studies reported the efficacy of ethanol extract of rhizome against various Gram-positive and Gram-negative bacteria including Helicobacter pylori (Wang and Huang Citation2005). Hexane and ethyl acetate extracts of leaves show antifungal action (Elzaawely et al. Citation2007). Kava pyrones (also known as kavalactones), dihydro-5,6-dehydrokawain, and 5,6-dehydrokawain, are the most notable phytochemical constituents (Xuan and Teschke Citation2015). Antioxidant (Chompoo et al. Citation2012), anti-platelet, anti-obesity (Tu and Tawata Citation2014), anti-glycation (Chompoo et al. Citation2011), osteogenic (Kumagai et al. Citation2016), and neuraminidase inhibitory (Upadhyay et al. Citation2011) activities have been documented for those compounds. Moreover, the extracts have various cardiovascular effects, such as hypotensive, vasorelaxant, and antihypertensive roles (Lahlou et al. Citation2003; de Moura et al. Citation2005).
The ever-increasing human population creates a high demand for medicinal plants and also destroys their natural habitat at the same time. Over-exploitation, well beyond their natural regenerative capacity, from shrinking populations, demands scientific cultivation and conservation effort. Particularly, high-frequency multiplication methods are to be developed for sustainable cultivation and conservation (Sharmin et al. Citation2014). In vitro propagation (tissue culture) can address the issues through rapid multiplication of rate and contributes to sustainable propagation, cultivation, and conservation (Anis and Ahmad Citation2016). Micropropagation offers true-to-type progenies and uniformity in its products, such as the bioactive compounds. Besides, in vitro conservation of germplasm (from healthy meristems) is advantageous through preventing the accumulation of pathogens and mutations and with reduced space and labor requirements (Alam et al. Citation2013).
Appropriate identification of plant species is essential for their medicinal use and conservation activities. Observation of morphological traits (shape, size, structure etc.) and organoleptic tests are commonly practiced for this. Besides, some high-tech qualitative and quantitative techniques, such as thin-layer chromatography, HPLC, GC-MS, and LC-NMR are also applied (Grazina et al. Citation2020). The former methods primarily depend on the expertise of the human sense, while the latter may be affected by several factors such as age, growth, and storage condition. These methods are not entirely accurate and unable to differentiate very closely related species, which share similar morphology or chemical profiles (Abubakar et al. Citation2017). DNA markers overcome these issues as they are not influenced by sample age, environmental factors, physiological and growth conditions, cultivation area, plant part, and storage condition (Abubakar et al. Citation2017). The method is easy, straightforward, works with any living tissue, and is universally applicable. The species identity is based on short, standardized DNA markers. The similarity of the test sample’s DNA sequence is matched against an established database of defined species resulting in the most accurate identity. Its automation compatibility allows testing hundreds of samples simultaneously. DNA barcoding also enables quick characterization of community species composition and richness at unprecedented spatial/temporal scales and taxonomic breadth (Zinger and Philippe Citation2016). Analyzing the vast amount of plant genome data, researchers conclude combining two regions (matK and rbcL) as a satisfactory compromise that best meets the DNA barcoding criteria. Supplementation with geographical origin further increases the accuracy of the species’ identity (Pečnikar and Buzan Citation2014).
A. zerumbet has been studied well for its phytochemicals. In vitro plant regeneration in A. zerumbet has been reported from seed-derived callus (Lin et al. Citation2016). However, this approach is not suitable for true-to-type seedlings. Culturing rhizome buds for in vitro shoot induction (Rakkimuthu et al. Citation2011; Victório et al. Citation2011) still requires improvement in terms of speed, multiplication rate, and especially, testing genetic uniformity among the seedlings. Moreover, explants from different geographical areas are needed to be assessed to present a widely applicable protocol since genotype affects in vitro culture responses. Reports on DNA-based identity and testing uniformity among tissue culture-raised plants are unavailable. Establishing DNA signatures is especially required for the genus Alpinia to delimit its generic boundary better (Kress et al. Citation2002). The objectives of the present work are to ascertain the molecular identification of this species and develop an efficient micropropagation protocol supported by a DNA marker-based genetic fidelity. These would be useful in identifying the medicinal plant accurately for the phytomedicine industry and in cultivation and conservation practices.
Materials and methods
Plant collection, maintenance, and taxonomic identification
Mature living plants with rhizomes were collected from a wild population located in the Rajshahi district of Bangladesh (24° 22’ 31.57"N 88° 38’ 10.61"E) in 2013 and planted and maintained in the Plant Conservatory of the National Institute of Biotechnology, Dhaka Bangladesh (23°57'43.3"N 90°16'45.9"E). Plant species identity is based on morphological features as previously described by Ahmed et al (Ahmed Citation2008).
DNA isolation and PCR
Young leaves were used to isolate DNA. A half gram of fresh leaf tissue was ground to fine powder in liquid nitrogen in a chilled mortar and pestle and mixed with 2% CTAB (cetyltrimethyl ammonium bromide) extraction buffer [2% w/v CTAB, 1.4 M NaCl, 0.5 M EDTA, 1 M Tris-HCl (pH 8.0), 0.2% (v/v) 2-marcaptoethanol]. Samples were homogenized in a 1.5 ml centrifuge tube and incubated in a water bath at 65°C for 25 min with occasional gentle swirling. Followed by centrifugation at 12,000 rpm for 15 min, the supernatant was mixed with phenol: chloroform: iso-amyl alcohol (25:24:1) in a new tube. The subsequent mixture was centrifuged and 1/10 volume of sodium acetate (3M) was added to the supernatant in another tube. The addition of ice-cold isopropanol revealed DNA precipitation. After incubation at −20°C for 30 min, the sample was centrifuged again and the upper aqueous layer was discarded. The pellet was washed in 70% ice-cold ethanol and centrifuged again for 10 min and the liquid was discarded. The DNA was then air-dried and re-suspended in 20 µl ultrapure water and stored at −20°C for future use (Tiwari et al. Citation2012). The quality and quantity of DNA were checked by measuring the absorbance of extracted DNA solution at 260 and 280 nm using a NanoDrop™ Spectrophotometer TM (Thermo Scientific) (Bhadra and Bandyopadhyay Citation2015).
PCR amplification of rbcL and matK
PCR amplification of matK and rbcL was carried out in 25 µL reaction containing nuclease-free water of 5 µl, master mix (2x) 12.50, 1 µl of each amplification primer, 5.50 µl of template DNA (50 ng) in a PCR-program (Gradient Thermal Cycler, Takara Bio Inc, Japan) meeting following conditions: 35 cycles 5 min at 94°C, 45 sec at 94°C, 45sec at 54°C, 1 min at 72, subsequent final elongation of 7 min at 72°C and extension for 1 min at 25°C for rbcL and 5 min at 94°C, 45 sec at 94°C, 45sec at 46°C, 1 min at 72, subsequent final elongation of 7 min at 72°C and extension for 1 min at 25°C for matK (Sahu et al. Citation2012). Primer sequences are as follows: rbcL (gtrbcL-F: 5’-TCT GTT ACT AAC ATG TTT ACT TC-3’; gtrbcL-R: 5’-TCC CTC ATT ACG AGC TTG TAC ACA-3’) and matK (Zin.matK-F: 5’-CGA TCT ATT CAT TCA ATA TTT C-3’; Zin.matK-R: 5’-TCT AGC ACA CGA AAG TCG AAG T-3’). The amplified products were separated by electrophoresis in 0.8% agarose gel buffered with 1X TBE. Gel was stained with RedSafe™ (iNtRON Biotechnology, Korea), and bands were observed in gel documentation system (FluorChem™ System, Bio-Techne). The size and number of the amplified DNA bands were determined by comparing with 1 kb DNA ladder (Promega, USA). The PCR products were sequenced by Sanger sequencing (3500 Genetic Analyzer, Applied Biosystems, USA). The 2 newly generated sequences were complemented with the NCBI nucleotide database using the nucleotide BLAST algorithm.
Micropropagation
Young rhizomes containing buds were collected from A. zerumbet strands and were used as the initial material for micropropagation. They were washed under running tap water to remove soils and treated with 3.25% NaOCl for 10 min under gentle shaking. Then the cleaned buds were sterilized by soaking it with intermittent agitation in 2% (w/v) Bavistin for 15 min. Then, the explants were washed in 0.1% (w/v) mercuric chloride for 5 min. The rhizome buds were subsequently washed six times (∼1 min each time) with sterile distilled water. Sterilized explants were then dissected to remove layers of leaf sheaths. Finally, the rhizomes were cut into pieces that consist of at least one active bud and inoculated onto the sterile shoot induction media. The shoot induction media was composed of MS (Murashige and Skoog) basal medium (Murashige and Skoog Citation1962) containing myo-inositol (100 mg L−1) and 30 gm L−1 sucrose and different concentrations of kinetin (Kin; 2.0–5.0 mg L−1) or 6-benzyl amino purine (BAP; 0.5 - 8.0 mg L−1) alone or in combinations of BAP and Kn with naphthalene acetic acid (NAA; 1.0-6.0 mg L−1) (Table ).
Table 1. Effects of different concentrations and combinations of BAP, Kin, and NAA on multiple shoot induction from the rhizome bud explant in Alpinia zerumbet grown in MS medium using 3% w/v sucrose. Shoot number and length were counted after 12 weeks of the respondents’ culture, while induction frequency was recorded in a separate experiment after four weeks of culture. Values are the means ± standard error. Different superscript in the same column indicates significant differences (P < 0.05) within treatments.
Six to ten weeks after culturing in shoot induction medium, they were sub-cultured in the same or plant growth regulators (PGR)-free MS medium for shoot multiplication. Multiple shoot clusters were divided into smaller shoot clumps with 2–3 shoots for continued shoot induction. For root induction, individually regenerated shoots were isolated from the cluster including small pieces of rhizome, and cultured in the rooting medium. Tested rooting media include full, and half strength of MS supplemented with various concentrations of IBA (0.5-3.0 mg L−1), or NAA (0.5-4.0 mg L−1; Table ).
Table 2. Effects of different concentrations of IBA and NAA on root induction of in vitro-grown micro-shoots of A. zerumbet. Data were recorded after four weeks of culture on the MS medium using 3% (w/v) sucrose. Values are the means ± standard error. Different superscript in the same column indicates significant differences (P < 0.05) within treatments.
In all cases, the pH of the medium was adjusted to 5.8 ± 0.05 with 0.1N NaOH or HCl before adding agar (0.8%, w/v) and was autoclaved at 121°C and 15 psi of pressure for 15 min. In some cases, PPM™ was added to the post-autoclaved culture medium (fixed aliquot). All the cultures were incubated at 25 ± 1°C under white fluorescent light during a photoperiod of 16:8 h light and dark cycles.
Well-rooted plants were removed from the culture medium, rinsed in water to remove media, and transferred to pots containing garden soil. A transparent polyethylene bag covered the plants to maintain high humidity avoiding dehydration. The plants were acclimatized in a growth chamber for about three weeks. The physical environment was maintained as follows: at 25 ± 1°C, 70-80% relative humidity and 45 µmol m–2 s–1 of light intensity with a photoperiod of 16:8 h light and dark cycles. The plants were watered regularly to maintain high humidity. The polyethylene bags were then opened gradually after 1–2 weeks when new leaf growth was observed. After 2–3 weeks, the potted plants were transferred to an outdoor condition in larger pots and subsequently to the field.
Data recording and statistical analysis
To test the effects of each treatment, eight explants were cultured and the whole experiment was repeated thrice. A one-way analysis of variance (ANOVA) was carried out to evaluate the effect of growth regulators on shoot formation and root induction. Results were expressed as the mean ± SE and differences in mean values are considered statistically significant at P < 0.05. Within the treatment groups, ANOVA was followed by Tukey’s honestly significant difference (HSD) test as a post hoc comparison. Data analyses were performed using SPSS statistical software (v 18.0).
Results
Taxonomic identification
Observation of the mature plants over two successive years in the germplasm conservation field revealed that the plant is a perennial rhizomatous herb. Morphological details were photographed and shown in Figure . Plant blooms from March to April in Bangladesh. Fruits rounded, 2.5 cm across, densely hairy. Based on the morphologic features, the plant is identified as A. zerumbet (Pers.) Burtt. & Smith (Ahmed Citation2008) Figure .
Figure 1. Morphological traits of A. zerumbet (a) portion of a stand at its full blossom (b) closer look of a flower (c) plant bearing immature fruits (d) different floral parts (e) enlarged view of stamen and stigma (f) cross-section of the ovary. Images are taken from the plant grown at the experimental field.
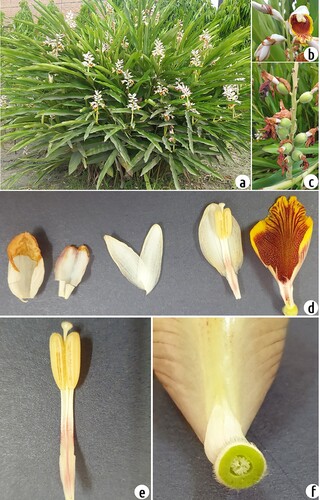
Molecular identification
DNA-based identification was carried out to link the morphological identity with molecular data. The genomic DNA extracted from the leaf sample had little contamination by polysaccharides and proteins, as indicated by the ratio of A260/A280 (1.78). Following PCR using the specified primers, clear banding patterns were observed for both matK and rbcL genes approximately at 850 and 950 bp, respectively (Figure ). Purified PCR products were subjected to Sanger sequencing followed by bioinformatics through the Standard Nucleotide Basic Local Alignment Search Tool (BLAST). For matK, the length of the sequence (NCBI accession MZ701800) was 762 bp, which has four BLAST hits with coverage 100% and 99.74 percent identity within A. zerumbet lineage with the accession numbers- MH603400.1, KX088467.1, JX088668.1, and HM850590.1. For rbcL, the sequence (NCBI accession MZ701799) was 792 bp long, which has two BLAST hits with coverage of 100% and 99.75 percent identity within A. zerumbet lineage with the accessions- MH603400.1 and JX088668.1.
Figure 3. Experimental results of multiple shoot induction and plant regeneration from rhizome buds explants of A. zerumbet (a) rhizome buds used as a source of explants, (b) shoot initiation from the inoculated rhizome buds in MS medium, (c-d) shoot proliferation in MS medium supplemented with 6.0 mg L−1 BAP, (e) shoot multiplication, (f) plantlets rooted on MS medium amended with 1.5 mg L−1 NAA (g) hardened plants of A. zerumbet in plastic pot.
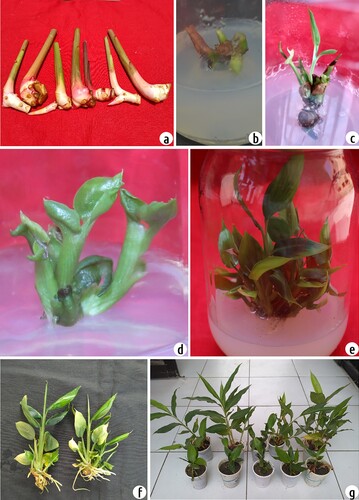
In vitro shoot multiplication
In vitro cultures of A. zerumbet were set up from the rhizome buds of field-grown plants. The explants were cultured on MS medium augmented with various concentrations of kinetin or BAP solely or combined with NAA to induce multiple shoots. Initiation of shoot induction was observed after two weeks of culture. Shoot clusters were sub-cultured at 30-day intervals in the same medium with 2–3 shoots per culture medium (Figure a-d).
Depending on growth regulator treatment, three types of morphogenesis were observed. Firstly, various concentrations of kinetin alone or combined with NAA produced long and single shoots. Secondly, the short and variable number of multiple shoot initiation represents a high micropropagation rate when cultured at ≥5.0 mg L−1 BAP and the absence of NAA. Finally, some explants showed no development or rarely formed shoot or amorphous structure without any growth regulator (MS0) (Table ). These observations suggest that the BAP at high concentration is essential and kinetin is not suitable for the micropropagation of A. zerumbet.
As shown in Table , the highest number of shoots was formed in 6.0 mg L−1 BAP and 30 g L−1 sucrose. The addition of NAA is not helpful in further increasing the shoot development. The highest percentage of the shoot (91.7%) was induced on a medium supplemented with 6.0 mg L−1 BAP followed by 5.0 and 4.0 mg L−1 BAP (83.3%). On the contrary, 0.5 mg L−1 BAP produced the lowest rate of shoots (25.0%). The highest mean number of shoots per explant (11.9) was also observed on medium supplemented with 6.0 mg L−1 BAP (Figure e). However, the longest shoot (4.48 cm) was generated on a medium containing 3.0 mg L−1 kinetin.
Upon subculture, their shoot formation pattern was the same as the primary culture. This multiplication rate was not declined in the successive subcultures on the same medium. Bacterial contamination appears in some culture vessels, and 0.5-5 ml L−1 of Plant preservative mixture (PPM™) was tested in a post-autoclaved culture medium to prevent this. It was found that 1 ml L−1 is the most suitable for preventing bacterial growth without compromising plant health.
In vitro and ex vitro rooting
During shoot cultures in BAP, root formation did not occur. Therefore, regenerated shoots with 3–5 cm height were isolated with a rhizome portion and transferred to rooting media amended with auxins (IBA, and NAA) for root induction. The cultured shoots induced root formation after three weeks of culture (Figure f). Among all the auxins tested, NAA was superior to the indole-3-butyric acid (IBA) for root induction rate and mean number of roots, while IBA was more effective than NAA in the case of the mean length of roots per culture (Table ).
The highest frequency of root formation (100%) was induced on MS medium supplemented with IBA (1.5 and 2.0 mg L−1) and also with NAA (1.5 mg L−1). The highest mean number of roots (13.04) per culture was observed in MS medium amended with NAA (1.5 mg L−1), subsequently followed by IBA (1.0 mg L−1) with 10.54 mean number of roots. The longest root (5.79 cm) was generated in a medium supplemented with NAA (1.5 mg L−1) and the shortest (3.38 cm) was also produced on a medium containing NAA with a concentration of 3.0 mg L−1 (Table ).
Fewer shoots that were contaminated by fungi were transferred directly to potting soil instead of rooting media. These shoots were irrigated with 5 mg L−1 IBA or NAA solution (∼2 ml) at 5-day intervals along with regular watering. Those irrigated shoots began to produce roots after 2–3 weeks and established themselves in the soil.
Acclimatization of the regenerated plantlets
Six weeks after transfer to an acclimatization environment, rooted plantlets showed a 95% survival rate. Compared with the mother plants from which they were derived, these acclimatized in vitro-regenerated plants showed no evident variation with respect to either growth characteristics or morphology (Figure g).
Genetic uniformity of micropropagated plants
Genetic variation is sometimes observed in plants that originated from tissue cultures, which is usually not desirable. Inter simple sequence repeats (ISSR) and random amplified polymorphic DNA (RAPD) markers were used to verify the genetic fidelity of the micropropagation procedure. Five randomly selected plants (regenerated from different explants) were chosen for the genetic homogeneity test. Eight RAPD primers amplified 53 scorable amplicons with the approximate range from 150 to 1700bp size (Table ; Figure a). The average of 6.63 PCR bands per one RAPD primer was amplified. In case eight ISSR primers amplified 60 scorable amplicons with an approximate range of 180–2990 bp size (Table ; Figure b). The average of 7.5 PCR bands per one ISSR primer was amplified. The amplification products were monomorphic across all the five micropropagated plants and mother plants produced by all ISSR and RAPD primers applied. These data suggest that the fidelity of the clonal plants obtained through the present protocol is very high.
Figure 4. (a) Representative agarose gel electrophoresis image of an amplified sequence from a RAPD reaction directed by primer OPA-13. Lane L: 1 kb plus ladder. Lane M: PCR amplification of mother plant DNA. Lane 1-5: PCR amplification of in vitro regenerated plants DNA. (b) Agarose gel electrophoresis of an amplified sequence from an ISSR reaction directed by primer UBC 822. Lane L: 1 kb plus ladder. Lane M: PCR amplification of mother plant DNA. Lane 1-5: PCR amplification of in vitro regenerated plants DNA.
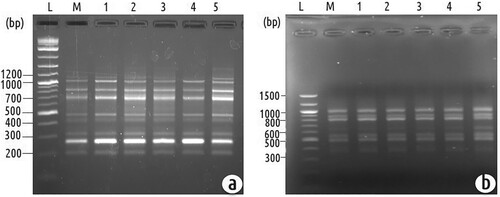
Table 3. List of random amplified polymorphic DNA (RAPD) and inter simple sequence repeat (ISSR) primers, their resultant amplicon sizes, and numbers.
Discussion
The correct identity of medicinal plants is essential to harness their actual benefit while minimizing negative effects. Zingiberaceae is a large family that had been characterized based on both vegetative and floral characteristics. However, in most cases, the identifying traits are either not unique to a particular group or are not universal for all taxa within a tribe. Therefore, molecular data are essential to establish species identity and explore the Zingiberaceae family’s phylogenetic relationships. In the present study, PCR amplification of matK and rbcL was carried out. Bioinformatics analysis of the matK and rbcL gene sequences indicated that the plant samples were A. zerumbet. In a previous study, researchers used the internal transcribed spacer 2 (ITS2) sequence for species identification of Alpinia species (Tan et al. Citation2020). However, analysis of the entire genome of chloroplast of A. oxyphy1lla suggests that the rbcL was found to be more useful in delimiting five species in the Zingiberaceae family, including A. zerumbet (Gao et al. Citation2019).
The natural propagation of this plant is slow and season-dependent. Moreover, industries often demand the production of true-to-type seedlings to fit a specific purpose once a desirable clone is identified. Micropropagation is very effective in meeting such demand, particularly for the rhizomatous Zingiberaceae species. In vitro regeneration of several medicinally important Zingiberaceae species has been reported (Stanly and Keng Citation2007; Kochuthressia et al. Citation2012).
Generally, microbial contamination is a major obstacle to starting aseptic cultures when using field-grown rhizomes. In Zingiber species, the contamination rate in in vitro cultures has been primarily attributed to the presence of outer leaf sheaths in rhizomes and the time of their collection (Bhowmik et al. Citation2016). However, proper washing procedures can reduce the contamination rate significantly due to the appropriate steps for surface sterilization. This study solved the issue by using 0.1% mercuric chloride. The efficacy of this agent has also been described for other rhizomatous species (Sharmin et al. Citation2013; Miri Citation2020).
The direction of in vitro morphogenesis depends on a balance of exogenously applied growth regulators and endogenous hormones. The growth hormone composition of the culture medium significantly affected shoot induction rate, number of shoots, as well as shoot length of plants in the present study. Higher rates of shoot induction and multiplication were observed in cultures on medium containing a high BAP level alone. The retardation of induction and proliferation was observed in shoots cultured upon the addition of NAA (Punyarani and Sharma Citation2012). BAP in in vitro culture has been reported to weaken apical dominance, break the dormancy of axillary buds in many Zingiber species (Thohirah et al. Citation2010; Punyarani and Sharma Citation2012). In the present study, the shoot length was higher on a medium containing solely kinetin. In some closely related species, the combination of two cytokinins (BAP and Kin) was helpful in shoot elongation and multiplication (Kochuthressia et al. Citation2012; Malhotra et al. Citation2021). However, adverse effects of multiple cytokinin or auxin combination have been reported in galanga species, where sole use of cytokinin was the most optimal (Rao et al. Citation2011).
In vitro microshoots of A. zerumbet were rooted in IBA and NAA. Microshoots did not produce roots in high BAP-containing media. Hence, additional treatment was required for root induction. It was observed that root induction occurred at a higher rate on MS media containing NAA. Root number was also high in NAA. While the mean length of roots was higher on MS media supplemented with IBA. Acclimatization of plantlets to soils is one of the most critical stages of a micropropagation protocol. In this investigation, the mortality rate of the plantlets with well-developed roots that were acclimatized and hardened was low. This is perhaps due to the formation of microrhizome, which contains sufficient storage compounds. A total of 23 plants were transferred to soils for acclimatization. Among these, 20 plants survived (87%).
Maintaining genetic stability is one of the most critical criteria among micropropagated plants. The occurrence of somaclonal variation due to in vitro culture is a significant problem that is often encountered. This causes a loss of properties of the micropropagated plants. The explants source, regeneration mode (Goto et al. Citation1998), culture age and the number of subcultures (Malhotra et al. Citation2021), changes in auxin-cytokinin concentrations and their ratio, in vitro stress due to unnatural conditions, altered nutritional conditions together or independently may contribute to somaclonal variations (Lakshmanan et al. Citation2007). Many scientists reported using molecular markers to confirm the genetic stability of micropropagated plants. DNA-based molecular markers have emerged as a potent practice for the purpose and are used in many plant species (Agarwal et al. Citation2015; Khatun et al Citation2018). A more effective analysis for the genetic homogeneity of plants was recommended using several marker systems as they target diverse sections of the genome (Lakshmanan et al. Citation2007). Generally, the non-coding sections of DNA are used in both ISSR and RAPD. They are semi-arbitrary to arbitrary, dominant, and show medium to high reproducibility. As a result, the clonal integrity of the in vitro-produced plant materials was confirmed in the current investigation by using two PCR-based DNA marker approaches, RAPD and ISSR. In this study, any variability among the micropropagated plantlets of A. zerumbet was not detected, which demonstrates the reliability of the in-vitro propagation system for this plant. In the study, plantlets were obtained from the rhizomatous buds. These findings support the fact that a rhizome-based micropropagation system is much more stable genetically than those in which regeneration occurs via the callus phase. Both RAPD and ISSR marker analysis revealed genetic polymorphism in Jatropha curcas plants regenerated from leaf-derived callus (El-Sayed et al. Citation2020).
Conclusion
The morphological and molecular identities of A. zerumbet are described in this paper. This paper also described an efficient micropropagation protocol followed by PCR-based DNA marker approaches that showed no genetic variation within micropropagated plants. This high-fidelity method can be employed for commercial-scale multiplication and in vitro conservation of the desired germplasm of A. zerumbet.
Author contributions
I. Alam conceived the idea and designed the research. KM Ahmed, SS Sarker, and T Tanny performed the experiments. AHMM Rahman conducted taxonomic identification. S. Nasrin and KC Das provided technical assistance. SS Sarker and I. Alam analyzed data and wrote the manuscript.
Availability of data and materials
The sequence data presented in the manuscript are submitted to the NCBI Nucleotide database (https://www.ncbi.nlm.nih.gov/nuccore) under the accession numbers MZ701799 and MZ701800. Raw data regarding the images used in the manuscript are available under the following DOIs: http://www.doi.org/10.17632/7nwgrxtx64.3; http://www.doi.org/10.17632/y44hrk82mx.1; http://www.doi.org/10.17632/4vggkbtf8w.1; http://www.doi.org/10.17632/md84km2rwr.2.
Compliance with ethical standards
Ethical approval is not applicable.
Special note
This work is dedicated to the memory of Professor Shamsun Nahar (1949-2021).
Disclosure statement
No potential conflict of interest was reported by the author(s).
Additional information
Funding
References
- Abubakar BM, Salleh FM, Omar MSS, Wagiran A. 2017. DNA barcoding and chromatography fingerprints for the authentication of botanicals in herbal medicinal products. Evidence-Based Complementary Altern Med. 2017:Article ID 1352948.
- Agarwal T, Gupta AK, Patel AK, Shekhawat N. 2015. Micropropagation and validation of genetic homogeneity of alhagi maurorum using Scot, ISSR and RAPD markers. Plant Cell Tissue Organ Culture (PCTOC). 120(1):313–323.
- Ahmed ZU, Hassan MA, Begum ZNT, Khondker M, Kabir SMH, Ahmad M, Ahmed ATA, Rahman AKA, Haque EU (eds.). 2008. Encyclopedia of flora and fauna of Bangladesh, Vol. 12. angiosperms: monocotyledones (Orchidaceae-Zingiberaceae). Dhaka: Asiatic Society of Bangladesh. pp. 461-462.
- Alam I, Sharmin SA, Naher M, Alam M, Anisuzzaman M, Alam MF. 2013. Elimination and detection of viruses in meristem-derived plantlets of sweetpotato as a low-cost option toward commercialization. 3 Biotech. 3:153–164.
- Anis M, Ahmad N. 2016. Plant tissue culture: propagation, conservation and crop improvement. Singapore: Springer, pp 15-44.
- Bhadra S, Bandyopadhyay M. 2015. A fast and reliable method for DNA extraction from different plant parts of Zingiberaceae. J Bot Soc Bengal. 69(2):91–98.
- Bhowmik SSD, Basu A, Sahoo L. 2016. Direct shoot organogenesis from rhizomes of medicinal zingiber alpinia calcarata rosc. and evaluation of genetic stability by RAPD and ISSR markers. J Crop Sci Biotechnol. 19(2):157–165.
- Chan EWC, Lim YY, Chong KL, Tan JBL, Wong SK. 2010. Antioxidant properties of tropical and temperate herbal teas. J Food Compos Anal. 23(2):185–189.
- Chompoo J, Upadhyay A, Fukuta M, Tawata S. 2012. Effect of alpinia zerumbet components on antioxidant and skin diseases-related enzymes. BioMed Central Complementary and Alternative Medicine. 12(1):106.
- Chompoo J, Upadhyay A, Kishimoto W, Makise T, Tawata S. 2011. Advanced glycation end products inhibitors from alpinia zerumbet rhizomes. Food Chem. 129(3):709–715.
- de Moura RS, Emiliano A, de Carvalho LCM, Souza MA, Guedes D, Tano T, Resende A. 2005. Antihypertensive and endothelium-dependent vasodilator effects of alpinia zerumbet, a medicinal plant. J Cardiovasc Pharmacol. 46(3):288–294.
- El-Sayed M, Aly UI, Mohamed MS, Rady MR. 2020. In vitro regeneration and molecular characterization of jatropha curcas plant. Bulletin of the National Research Centre. 44(1):1–12.
- Elzaawely AA, Xuan TD, Tawata S. 2007. Essential oils, kava pyrones and phenolic compounds from leaves and rhizomes of alpinia zerumbet (pers.) BL burtt. & RM Sm. and their antioxidant activity. Food Chem. 103(2):486–494.
- Gao B, Yuan L, Tang T, Hou J, Pan K, Wei N. 2019. The complete chloroplast genome sequence of alpinia oxyphylla Miq. and comparison analysis within the Zingiberaceae family. PLoS One. 14(6):e0218817.
- Goto S, Thakur R, Ishii K. 1998. Determination of genetic stability in long-term micropropagated shoots of pinus thunbergii parl. using RAPD markers. Plant Cell Rep. 18(3):193–197.
- Grazina L, Amaral JS, Mafra I. 2020. Botanical origin authentication of dietary supplements by DNA-based approaches. Compr Rev Food Sci Food Saf. 19(3):1080–1109.
- Khatun MM, Tanny T, Yesmin S, Salimullah MD, Alam I. 2018. Evaluation of genetic fidelity of in vitro-propagated aloe vera plants using DNA-based markers. Sci Asia. 44:87–91.
- Kochuthressia K, Britto SJ, Jaseentha M. 2012. In vitro multiplication of kaempferia galanga L. an endangered species. Int Res J Biotechnol. 3(2):027–031.
- Kress WJ, Prince LM, Williams KJ. 2002. The phylogeny and a new classification of the gingers (Zingiberaceae): evidence from molecular data. Am J Bot. 89(10):1682–1696.
- Kumagai M, Mishima T, Watanabe A, Harada T, Yoshida I, Fujita K, Watai M, Tawata S, Nishikawa K, Morimoto Y. 2016. 5, 6-Dehydrokawain from alpinia zerumbet promotes osteoblastic MC3T3-E1 cell differentiation. Biosci Biotechnol Biochem. 80(7):1425–1432.
- Lahlou S, Interaminense LFL, Leal-Cardoso JH, Duarte GP. 2003. Antihypertensive effects of the essential oil of alpinia zerumbet and its main constituent, terpinen-4-ol, in DOCA-salt hypertensive conscious rats. Fundam Clin Pharmacol. 17(3):323–330.
- Lakshmanan V, Reddampalli Venkataramareddy S, Neelwarne B. 2007. Molecular analysis of genetic stability in long-term micropropagated shoots of banana using RAPD and ISSR markers. Electron J Biotechnol. 10(1):106–113.
- Lin M, Li J, Tang Q, Gong J, Jiang J, Zhong C, Wang H. 2016. Establishment of in vitro propagation technology of alpinia zerumbet variegata. J South Agric. 47(11):1909–1913.
- Malhotra EV, Kamalapriya M, Bansal S, Meena D, Agrawal A.. 2021. Improved protocol for micropropagation of genetically uniform plants of commercially important cardamom (Elettaria cardamomum maton). Vitro Cell Dev Biol-Plant. 57:409–17.
- Miri SM. 2020. Micropropagation, callus induction and regeneration of ginger (zingiber officinale rosc.). Open Agric. 5(1):75–84.
- Murashige T, Skoog F. 1962. A revised medium for rapid growth and bio assays with tobacco tissue cultures. Physiol Plant. 15(3):473–497.
- Pečnikar ŽF, Buzan EV. 2014. 20 years since the introduction of DNA barcoding: from theory to application. J Appl Genet. 55(1):43–52.
- Punyarani K, Sharma JG. 2012. Micropropagation and microrhizome induction in costus pictus D. Don using in vitro and ex vitro nodal segments as explant. Notulae Scientia Biologicae. 4(2):72–78.
- Rakkimuthu R, Jacob J, Aravinthan K. 2011. In vitro micropropagation of alpinia zerumbet variegate, an important medicinal plant, through rhizome bud explants. Res Biotechnol. 2(1):7–10.
- Rao K, Chodisetti B, Gandi S, Mangamoori LN, Giri A. 2011. Direct and indirect organogenesis of alpinia galanga and the phytochemical analysis. Appl Biochem Biotechnol. 165(5):1366–1378.
- Sahu SK, Thangaraj M, Kathiresan K. 2012. DNA extraction protocol for plants with high levels of secondary metabolites and polysaccharides without using liquid nitrogen and phenol. Int Sch Res Notices. 2012:Article ID 205049.
- Sharmin SA, Alam MJ, Sheikh MMI, Sarker KK, Khalekuzzaman M, Haque MA, Alam MF, Alam I. 2014. Somatic embryogenesis and plant regeneration in wedelia calendulacea less., an endangered medicinal plant. Braz Arch Biol Technol. 57:394–401.
- Sharmin SA, Alam MJ, Sheikh MMI, Zaman R, Khalekuzzaman M, Mondal SC, Haque MA, Alam MF, Alam I. 2013. Micropropagation and antimicrobial activity of curcuma aromatica salisb., a threatened aromatic medicinal plant. Turk J Biol. 37(6):698–708. 21.
- Stanly C, Keng CL. 2007. Micropropagation of curcuma zedoaria roscoe and zingiber zerumbet smith. Biotechnology. 6(4):555–560.
- Tan WH, Chai LC, Chin CF. 2020. Efficacy of DNA barcode internal transcribed spacer 2 (ITS 2) in phylogenetic study of alpinia species from peninsular Malaysia. Physiol Mol Biol Plants. 26(9):1889–1896.
- Thohirah L, Flora C, Kamalakshi N. 2010. Breaking bud dormancy and different shade levels for production of pot and cut cucurma alismatifolia. Amer J Agric Biol Sci. 5(3):285–388.
- Tiwari K, Jadhav S, Gupta S. 2012. Modified CTAB technique for isolation of DNA from some medicinal plants. Res J Med Plant. 6(1):65–73.
- Tu PTB, Tawata S. 2014. Anti-obesity effects of hispidin and alpinia zerumbet bioactives in 3T3-L1 adipocytes. Molecules. 19(10):16656–16671.
- Upadhyay A, Chompoo J, Kishimoto W, Makise T, Tawata S. 2011. HIV-1 integrase and neuraminidase inhibitors from alpinia zerumbet. J Agric Food Chem. 59(7):2857–2862.
- Victório CP. 2011. Therapeutic value of the genus alpinia, Zingiberaceae. Braz J Pharmacognosy. 21(1):194–201.
- Victório CP, Kuster RM, Lage CLS. 2011. Leaf and root volatiles produced by tissue cultures of alpinia zerumbet (pers.) burtt & smith under the influence of different plant growth regulators. New Chem. 34:430–433.
- Wang YC, Huang TL. 2005. Screening of anti-helicobacter pylori herbs deriving from Taiwanese folk medicinal plants. Fed Eur Microbiol Societies (FEMS) Immunol Med Microbiol. 43(2):295–300.
- Xuan T, Quan N, Quan N, Rayee R, Khanh T, Tran H, Trung N. 2019. Allelopathic plants: 26. alpinia zerumbet (pers.) BL burtt & RM Sm. (Zingiberaceae). Allelopath J. 48(1):1–13.
- Xuan TD, Teschke R. 2015. Dihydro-5, 6-dehydrokavain (DDK) from alpinia zerumbet: Its isolation, synthesis, and characterization. Molecules. 20(9):16306–16319.
- Zinger L, Philippe H. 2016. Coalescing molecular evolution and DNA barcoding. Mol Ecol. 25(9):1908–1910.