Abstract
The mechanisms involved in the coupling between cardiac and locomotor rhythms in patients with heart disease remain unclear. It was speculated that reduced function of the cardiovascular and/or locomotor systems would increase the coupling of these systems to increase energy efficiency in response to increased energy demand during exercise. We investigated the development of cardiolocomotor coupling (CLC) in patients with coronary artery disease. Eight outpatients with coronary artery disease (CAD group) and eight healthy individuals (control group) underwent cardiopulmonary exercise testing involving a cycle ergometer ramp protocol and treadmill walk test to record CLC strength. The coefficient of determination (R2 value) of the interval between R waves on the electrocardiograph and step interval reflects CLC strength intensity. The Student’s t-test was used to compare cardiopulmonary exercise testing data and R2 values between the study groups. The R2 value was significantly higher in the CAD group than the control group. Peak oxygen uptake and oxygen uptake at the anaerobic threshold were significantly lower in the CAD group than the control group. Our findings showed that CLC intensity was higher in patients with CAD, suggesting that CLC is more likely to occur in individuals with reduced exercise tolerance due to cardiorespiratory deterioration.
Key highlights
Eight outpatients with coronary artery disease and eight healthy individuals underwent cardiopulmonary exercise and treadmill walk tests to determine cardiolocomotor coupling strength and physiological characteristics.
This study reports a stronger cardiolocomotor coupling during exercise in patients with coronary artery disease compared with healthy individuals, suggesting that cardiolocomotor coupling is more likely to occur in people with reduced exercise tolerance attributed to impaired cardiorespiratory function.
Our results support the theory that patients with cardiorespiratory impairment due to coronary artery disease have greater dynamic interaction between the locomotor and cardiovascular systems during exercise, which is concurrent with the hypothesis that cardiolocomotor coupling is a compensatory phenomenon for exercise maintenance.
Introduction
The rhythm of the human heart is influenced by various external and internal factors (Glass Citation2001). During cyclical exercise, such as walking, running, or cycling, the heart rate rhythm becomes synchronized with the exercise rhythm (Kirby et al. Citation1989; Nomura et al. Citation2003; Niizeki and Saitoh Citation2014). This phenomenon is known as cardiolocomotor coupling (CLC). CLC has been reported to increase stroke volume and blood flow to skeletal muscle during exercise as a result of optimal phase synchronization between heart rate and exercise rhythms (Niizeki Citation2005; Takeuchi et al. Citation2014; Takeuchi et al. Citation2015; Giuriato et al. Citation2020). Therefore, CLC has been reported to be a biological response that optimizes peripheral circulation during exercise (Niizeki Citation2005).
However, the mechanisms and characteristics of CLC development remain unclear. CLC is thought to occur when the heart rate rhythm is influenced by the exercise rhythm (Kawahara et al. Citation1994). A previous study reported that CLC occurred when both thighs were rhythmically compressed in a resting sitting position using a cuff to simulate intramuscular pressure during walking (Niizeki Citation2005). The effects of body vertical acceleration, central command, and skeletal muscle metabolic receptors during exercise are not considered to be essential to the mechanism involved in the development of CLC. The main mechanisms of CLC are thought to involve suppression of cardiac vagal activity by ascending signals from skeletal muscle mechanoreceptors associated with skeletal muscle contraction (Legramante et al. Citation2000) and acceleration of cardiac rhythm by stretching of the sinus node due to an increased venous return by the skeletal muscle pump (Kohl et al. Citation1999). In addition to these factors, variations in heart rhythm due to respiratory sinus arrhythmias (RSAs) and arterial baroreflex may be modifying factors in the development of CLC (Niizeki et al. Citation1993; Novak et al. Citation2007; Takeuchi et al. Citation2014). Novak et al. reported that CLC was more likely to occur in the elderly than the young due to the reduced appearance of RSAs and reduced baroreceptor reflex with age (Novak et al. Citation2007). We previously investigated the physiological significance of CLC during walking in healthy young people and reported variation in the intensity of CLC during its induction (Takeuchi et al. Citation2014). We subsequently examined factors associated with the intensity of CLC in young healthy individuals using a previously reported method (Novak et al. Citation2007) and found a higher incidence of CLC in individuals with a low exercise tolerance, low cardiac pump function during exercise, and low exercise habits (Takeuchi et al. Citation2018). This suggests that age does not directly affect the development of CLC but that various exercise-related factors influence the development of CLC.
These previous findings suggest that CLC is a compensatory phenomenon that acts to maintain peripheral circulation during exercise in the presence of impaired cardiac and exercise function. Furthermore, the decline in circulatory and motor functions related to the maintenance of exercise may influence the occurrence of CLC, which may have therapeutic and diagnostic applications. However, no previous studies have investigated the characteristics of CLC development in patients with impaired respiratory and circulatory function to maintain exercise. Therefore, the present study examined the development of CLC in patients with impaired cardiac function and exercise tolerance due to heart disease.
Materials and methods
Study participants
Eight male outpatients diagnosed with coronary artery disease (CAD group) and eight healthy age and sex-matched individuals with no history of respiratory or cardiovascular disease (control group) participated in the study. The inclusion criteria for the CAD group were: diagnosis of myocardial infarction or angina pectoris; no clear signs or symptoms of heart failure; at least 1 mo since completion of treatment; at least 2 weeks since the start of outpatient cardiac rehabilitation; no exacerbation of symptoms or change in medication 1 week prior to measurements; no medication for arrhythmia other than beta blockers; and the ability to walk unaided stably for at least 30 min. Individuals with atrial fibrillation were excluded due to the need to analyze heart rhythms derived from the sinus node. The control group participants were recruited from the Silver Human Resources Center. The characteristics of the study participants are shown in Table and the medical and treatment characteristics of the individuals in the CAD group are shown in Table . The purpose and methods of the study were explained to the study participants verbally and in writing and all participants signed a consent form to take part in the study. The experimental protocol for this study was approved by the ethics committee of the Hamamatsu University School of Medicine (R14-132). This study was conducted in accordance with the Declaration of Helsinki. The participants were not allowed to perform strenuous exercise and consume alcohol or caffeine for 24 h before the study. Moreover, they were not allowed to consume any food or fluids except for water for 3 h before the study.
Table 1. Characteristics of the study participants.
Table 2. Medical and treatment characteristics of the CAD group.
Protocols
Data measurements were taken between April 2013 and March 2014. Participants performed two exercise tests spaced approximately 1 week apart. The first experiment was a cardiopulmonary exercise stress test to assess the exercise tolerance of the participant and the second experiment was a treadmill walking test to examine the intensity of CLC development. All experiments were carried out in the presence of a doctor.
The cardiopulmonary exercise stress test was performed using a bicycle ergometer for safety reasons. The participant wore a wireless 12-lead electrocardiograph (Labtech Ltd.), which included a brachial sphygmomanometer and finger-peak pulse oximeter, and a mask attached to an expiratory gas analyzer (AE-310S, Minato Medical Science Co., Ltd.). The participant then cycled a bicycle ergometer (AEROBIKE 75XLIII, Konami Sports Club Co., Ltd) to start the measurements. The protocol for the cardiopulmonary exercise test (CPX) comprised a 5-min rest, 4-min warm-up at 10 W, and exercise with a 10-W ramp load until the end of the test. The pedal speed was set at 50 revolutions per min and participants were instructed to pedal in time with an electronic metronome. Exercise was discontinued if the pedal speed could not be maintained despite instruction, in accordance with the American College of Sports Medicine completion criteria. Oxygen uptake, carbon dioxide emission, and ventilation were measured in each breath. Electrocardiogram waveforms, heart rate, and transcutaneous oxygenation were recorded throughout the cardiopulmonary exercise test. Blood pressure and subjective exercise intensity were recorded every minute. Peak oxygen uptake (peak VO2) and oxygen uptake at the anaerobic threshold (VO2 at AT) were calculated as indicators of exercise tolerance. Anaerobic threshold (AT) was determined using the V-slope method; however, the trend waveform was used as a criterion for determining AT when AT was difficult to determine.
Participants performed a walking test on a treadmill at intervals of at least 1 week after the CPX to examine the degree of CLC during walking. Several previous studies have reported several methods to ascertain the intensity of CLC occurrences (Nomura et al. Citation2003; Novak et al. Citation2007; Niizeki and Saitoh Citation2014; Takeuchi et al. Citation2014; Materko et al. Citation2015; Constantini et al. Citation2018), but several methods induce CLC by forcing subjects to walk or run to a beep. Thus, they fail to assess spontaneous occurrences of CLC. In addition, forcing a walking rhythm might have overloaded the patients in our study, including the elderly, and may have involved a risk of falling. Therefore, we used the protocol and analysis methods of Novak et al. (Novak et al. Citation2007), who investigated the occurrence of CLC in elderly patients. Prior to the test, participants walked on the treadmill as a practice exercise and their normal walking speed was measured. The participant then wore a heart rate sensor (FA-DL-330, 4Assist, Inc.) on their chest and a footswitch sensor (FA-DL-250, 4Assist, Inc.) on each heel. They then performed a resting seated position for 5 min and a resting standing position for 5 min on the treadmill (Autorunner AR-200, Minato Medical Science Co., Ltd.) followed by a gradual loading walk test. The incremental loading protocol was performed as previously described (Novak et al. Citation2007), starting with a treadmill speed of 1.3 km/h and increasing by 0.3 km/h every 30 s until the normal walking speed was reached. The interval between R waves on the electrocardiograph (R–R interval) and timing of the heel–ground contact (step interval) were measured using a heart rate sensor and footswitch sensor, respectively, at a sampling frequency of 1,000 Hz. Each signal was digitized via an analog interface (FA-DL-720, 4Assist, Inc.) and an analog-to-digital converter (ML880 PowerLab 16/30, ADInstruments) and stored using time series data analysis software (Chart 5 for Windows, ADInstruments) on a personal computer. The step intervals at all speeds and corresponding R–R intervals were used to calculate the coefficient of determination (R2). R2 was defined in the present study as the intensity of the occurrence of CLC, in accordance with previous studies (Novak et al. Citation2007). R2 is calculated on a scale of 0–1, with higher values indicating stronger synchronization between heart rate and exercise rhythms, and lower values indicating weaker or no synchronization.
Statistical analyses
Values are expressed as mean ± standard deviation. The Student’s t-test was used for comparisons between groups for participants’ characteristics, walking test data, and cardiopulmonary exercise testing data. Statistical software used was IBM SPSS Statistics 19.0 for Windows (SPSS IBM Japan Inc.). Spearman’s rank correlation coefficient test was also performed on peak VO2 and R2 values to confirm the relationship between exercise tolerance and the occurrence of CLC in the CAD group. A non-parametric method was used because of the low number of patients. The significance level was set at a two-sided risk of >5%.
Results
No measurement-related adverse events were observed in any of the participants. A comparison of participants’ characteristics between the groups showed no significant differences in age, height, weight, body mass index, and normal walking speed (Table ). A comparison between the intensity of CLC development and cardiopulmonary exercise stress test data is shown in Table .
Table 3. Comparison of strength of cardiolocomotor coupling and cardiopulmonary exercise testing data.
Figure shows the relationship between the R–R interval and step interval for one representative of each group. The relationship between the R–R interval and step interval was stronger for the representative of the CAD group than for that of the control group. In terms of R2 values, which reflect the intensity of CLC development, the value was significantly higher in the CAD group than in the control group (0.67 ± 0.17 vs. 0.33 ± 0.26, P < 0.05).
Figure 1. Relationship between R–R intervals and step intervals of representative participants in the coronary artery disease (CAD) and control groups. The R–R interval and step interval during treadmill walking with progressive load are indicated as dots. The coefficient of determination (R2 value) of the R–R interval and step interval represent the intensity of cardiolocomotor coupling. A and B indicate one representative from the CAD and control groups, respectively. A strong relationship can be observed between the R–R interval and step interval in A.
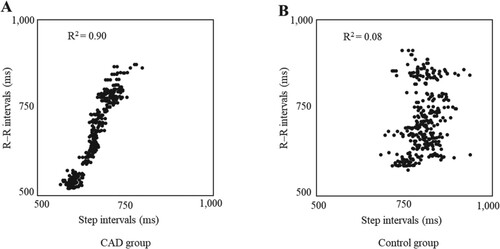
Peak VO2 and its percentage of the predicted value were significantly lower in the CAD group than in the control group (peak VO2: 17.3 ± 1.9 mL/kg/min vs. 21.9 ± 3.9 mL/kg/min, P < 0.05; % predicted peak VO2: 69.4% ± 10.6% vs. 91.3% ± 15.4%, P < 0.05). Additionally, VO2 at AT and its percentage of the predicted value were significantly lower in the CAD group than in the control group (VO2 at AT: 11.4 ± 1.6 mL/kg/min vs. 15.9 ± 3.5 mL/kg/min, P < 0.05; % predicted VO2 at AT: 71.1% ± 10.5% vs. 100.4% ± 21.3%, P < 0.05). Systolic blood pressure, diastolic blood pressure, heart rate, and workload were not significantly different between the two groups.
Figure shows the relationship between the R2 values and peak VO2 in the CAD group. R2 values showed a significant negative correlation with peak VO2 (rs = −0.79, P < 0.05).
Figure 2. Relationship between the R2 values and peak oxygen uptake in the coronary artery disease group. R2 values represent the intensity of cardiolocomotor coupling. Figure shows a significant negative rank correlation between peak oxygen uptake and R2 values in the coronary artery disease group. Statistics are based on Spearman’s rank correlation coefficient test.
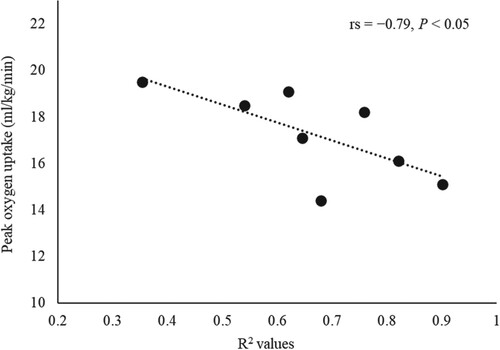
Discussion
Peak VO2 and VO2 at AT were significantly lower in the CAD group, suggesting a reduced exercise tolerance in participants in the CAD group compared with those in the control group. The R2 value was significantly higher in the CAD group, indicating a higher incidence of CLC. Furthermore, in the CAD group, the peak VO2 was negatively correlated with the R2 value, where a lower peak VO2 correlated with a higher R2 value. These results suggest that patients with reduced exercise tolerance due to CAD are more likely to develop CLC during walking than healthy age-matched individuals.
As exercise-induced changes in heart rate are mainly caused by central commands, muscle metabolic receptor reflexes, and muscle mechanoreceptor reflexes (Iellamo Citation2001), these factors have also been the focus as a mechanism for the development of CLC. However, as mentioned in the Introduction, CLC was also observed in cuff-simulated studies of intramuscular pressure during walking without actual exercise, suggesting that the effects of central commands and skeletal muscle metabolic receptor reflexes are not the main mechanism for CLC generation (Niizeki Citation2005). Therefore, we discuss the current leading two hypotheses of ascending signals from skeletal muscle mechanoreceptors (Legramante et al. Citation2000) and stretching of the sinus node due to an increased venous return (Kohl et al. Citation1999), in relation to the pathophysiology of CAD.
First, the association between elevated signals from skeletal muscle mechanoreceptors and patients with CAD or heart failure is discussed. Previous animal studies have reported a more pronounced increase in heart rate due to the muscle mechanoreceptor reflex in groups of myocardial infarction and heart failure models compared to control groups (Smith et al. Citation2003; Li et al. Citation2004; Smith et al. Citation2005; Wang et al. Citation2010). However, recent human studies have shown that skeletal muscle mechanoreceptor reflexes have a small effect on the increase in heart rate and there are no significant differences between heart failure patients and healthy individuals (Witman et al. Citation2015; Ives et al. Citation2016). These findings suggest that inhibition of peripheral vascular distensibility may be involved in the excessive pressure-raising reflex, with little effect on heart rate. Furthermore, skeletal muscle mechanoreceptor reflexes may not play a significant role in the higher intensity of CLC in patients with CAD.
Second, the association between stretching of the sinus node due to an increased venous return and patients with CAD or heart failure is discussed. The effect of sinus node stretch on increased venous return was also examined. Increased heart rate due to stretch stimulation was also reported as an intrinsic mechanism in the sinus node that does not involve the nervous system (Kohl et al. Citation1999). This was also observed in human heart rate and may be responsible for the observation of RSAs in individuals during autonomic blockade (Casadei et al. Citation1996; El-Omar et al. Citation2001) as well as denervated hearts after heart transplantation (Bernardi et al. Citation1990; Radaelli et al. Citation1996). This intrinsic mechanism of heart rate variability was reported to cause around 3% of RSAs in healthy individuals, whereas this rate increased to about 15% in patients with chronic heart failure compared with healthy age-matched individuals (El-Omar et al. Citation2001). Therefore, it is possible that heart rate modulation by intrinsic mechanisms of the sinus node is also greater in patients with CAD than in healthy individuals. These results suggest that the mechanism of sinus node stretching associated with increased venous return may contribute to the increased intensity of CLC development in patients with CAD.
Several studies (O'Rourke and Avolio Citation1992; Niizeki Citation2005; Takeuchi et al. Citation2015) have shown that CLC may reduce myocardial stress during exercise by increasing the effectiveness of blood circulation to contracting muscles. Our results support the theory that patients with cardiorespiratory impairment due to CAD have a greater dynamic interaction between the locomotor and cardiovascular systems during exercise. This supports the hypothesis that CLC is a compensatory response to maintain exercise, a phenomenon that emerges as a result of reduced exercise tolerance due to impaired or reduced circulatory and motor function. Although the results of this study do not alone elucidate the mechanism of CLC development, they do suggest a close relationship between CLC and the biological functions that maintain exercise. In addition, if endogenous mechanisms in the myocardium are involved in the development of CLC, as addressed earlier in the Discussion, the development of CLC could be used for noninvasive diagnosis and assessment of myocardial function. Given the preliminary nature of this study (for safety and ethical reasons), the number of participants was small, and medications, comorbidities, and CAD severity could not be controlled. However, future progression to large-scale epidemiological studies could lead to the establishment of clinical assessment indicators. The results of this study may contribute to the establishment of noninvasive assessment methods for patients with cardiovascular disease and its premorbidities.
The present study has some limitations. First, we were unable to discontinue use of medicines, such as beta blockers and antihypertensive drugs, in terms of risk management for the participants. Beta blockers have been reported to increase RSAs (Niemelä et al. Citation1994); therefore, we cannot exclude the possibility that they may have influenced the occurrence of CLC. However, increased RSAs may attenuate the incidence of CLC (Niizeki et al. Citation1993; Novak et al. Citation2007; Takeuchi et al. Citation2014); therefore, this does not affect our conclusion that CLC is more intense in patients with CAD than healthy individuals. Conversely, there was no significant difference in blood pressure between the CAD and control groups, possibly because blood pressure was controlled to normal levels by the medication. Second, this was a preliminary study, and we were unable to categorize patients according to severity of illness or comorbidity. Further studies with larger numbers of participants will be needed not only to clarify the relationship between CAD characteristics and the incidence of CLC but also the effects of autonomic neuropathy secondary to comorbid diabetes and myocardial damage from comorbid heart failure. Third, this was a cross-sectional study, and we were unable to confirm changes in the incidence of CLC in each individual over time. Further studies are required to control for diurnal variation, the effects of medications, and the relevance of rehabilitation and other treatments. Finally, the present study adopted the protocols and analysis methods of previous studies investigating the occurrence of CLC in elderly individuals, with a view to adapting them to all patients. However, phase synchronization cannot be confirmed using the methods of the present study. Whether the results of the present study can be compared with other CLC studies is debatable. In the future, it will also be necessary to consider a reasonable indicator of CLC occurrence.
Conclusions
The present study compared the incidence of CLC during walking in patients with CAD and healthy individuals. Our results showed a higher CLC intensity in patients with CAD, suggesting that CLC is more likely to occur in individuals with reduced exercise tolerance due to cardiac disease.
Author contributions statement
Shinta Takeuchi contributed to the conception and design of the study; acquisition of data, analysis, and interpretation of data; drafting of the manuscript and revision of the manuscript. Yusuke Nishida contributed to the conception and design of the study. All authors read and approved the final manuscript.
Acknowledgments
We thank the subjects who participated in this study. The authors would like to thank Enago (www.enago.jp) for the English language review.
Data availability statement
The data that support the findings of this study are openly available in figshare at https://doi.org/10.6084/m9.figshare.20205683.v2
Disclosure statement
No potential conflict of interest was reported by the author(s).
Additional information
Funding
References
- Bernardi L, Salvucci F, Suardi R, Soldá PL, Calciati A, Perlini S, Falcone C, Ricciardi L. 1990. Evidence for an intrinsic mechanism regulating heart rate variability in the transplanted and the intact heart during submaximal dynamic exercise? Cardiovasc Res. 24(12):969–981. doi:10.1093/cvr/24.12.969.
- Casadei B, Moon J, Johnston J, Caiazza A, Sleight P. 1996. Is respiratory sinus arrhythmia a good index of cardiac vagal tone in exercise? J Appl Physiol. 81(2):556–564. doi:10.1152/jappl.1996.81.2.556.
- Constantini K, Stickford ASL, Bleich JL, Mannheimer PD, Levine BD, Chapman RF. 2018. Synchronizing gait with cardiac cycle phase alters heart rate response during running. Med Sci Sports Exerc. 50(5):1046–1053. doi:10.1249/MSS.0000000000001515.
- El-Omar M, Kardos A, Casadei B. 2001. Mechanisms of respiratory sinus arrhythmia in patients with mild heart failure. Am J Physiol Heart Circ Physiol. 280(1):H125–H131. doi:10.1152/ajpheart.2001.280.1.H125.
- Giuriato G, Ives SJ, Tarperi C, Bortolan L, Ruzzante F, Pedrinolla A, Martignon C, Laginestra FG, Cevese A, Schena F, et al. 2020. Timed synchronization of muscle contraction to heartbeat enhances muscle hyperemia. J Appl Physiol (Bethesda M.D. 1985). 128:805–812. doi:10.1152/japplphysiol.00898.2019.
- Glass L. 2001. Synchronization and rhythmic processes in physiology. Nature. 410(6825):277–284. doi:10.1038/35065745.
- Iellamo F. 2001. Neural mechanisms of cardiovascular regulation during exercise. Auton Neurosci. 90(1–2):66–75. doi:10.1016/S1566-0702(01)00269-7.
- Ives SJ, Amann M, Venturelli M, Witman MA, Groot HJ, Wray DW, Morgan DE, Stehlik J, Richardson RS. 2016. The mechanoreflex and hemodynamic response to passive leg movement in heart failure. Med Sci Sports Exerc. 48(3):368–376. doi:10.1249/MSS.0000000000000782.
- Kawahara K, Yamauchi Y, Niizeki K, Yoshioka T. 1994. Interactions between respiratory, cardiac and stepping rhythms in decerebrated cats: functional hierarchical structures of biological oscillators. Methods Inf Med. 33(1):129–133. doi:10.1055/s-0038-1634972.
- Kirby RL, Nugent ST, Marlow RW, MacLeod DA, Marble AE. 1989. Coupling of cardiac and locomotor rhythms. J Appl Physiol (Bethesda M.D. 1985). 66(1):323–329. doi:10.1152/jappl.1989.66.1.323.
- Kohl P, Hunter P, Noble D. 1999. Stretch-induced changes in heart rate and rhythm: clinical observations, experiments and mathematical models. Prog Biophys Mol Biol. 71(1):91–138. doi:10.1016/S0079-6107(98)00038-8.
- Legramante JM, Raimondi G, Adreani CM, Sacco S, Iellamo F, Peruzzi G, Kaufman MP. 2000. Group III muscle afferents evoke reflex depressor responses to repetitive muscle contractions in rabbits. Am J Physiol Heart Circ Physiol. 278(3):H871–H877. doi:10.1152/ajpheart.2000.278.3.H871.
- Li J, Sinoway AN, Gao Z, Maile MD, Pu M, Sinoway LI. 2004. Muscle mechanoreflex and metaboreflex responses after myocardial infarction in rats. Circulation. 110(19):3049–3054. doi:10.1161/01.CIR.0000147188.46287.1B.
- Materko W, Nadal J, AMFLMd S. 2015. Investigating cardiolocomotor synchronization during running in trained and untrained males. Res Biomed Eng. 31(2):176–186. doi:10.1590/2446-4740.0623.
- Niemelä MJ, Airaksinen KEJ, Huikuri HV. 1994. Effect of Beta-blockade on heart rate variability in patients with coronary artery disease. J Am Coll Cardiol. 23(6):1370–1377. doi:10.1016/0735-1097(94)90379-4.
- Niizeki K. 2005. Intramuscular pressure-induced inhibition of cardiac contraction: implications for cardiac-locomotor synchronization. Am J Physiol Regul Integr Comp Physiol. 288(3):R645–R650. doi:10.1152/ajpregu.00491.2004.
- Niizeki K, Kawahara K, Miyamoto Y. 1993. Interaction among cardiac, respiratory, and locomotor rhythms during cardiolocomotor synchronization. J Appl Physiol (Bethesda M.D. 1985). 75(4):1815–1821. doi:10.1152/jappl.1993.75.4.1815.
- Niizeki K, Saitoh T. 2014. Cardiolocomotor phase synchronization during rhythmic exercise. J Phys Fit Sports Med. 3(1):11–20. doi:10.7600/jpfsm.3.11.
- Nomura K, Takei Y, Yanagida Y. 2003. Comparison of cardio-locomotor synchronization during running and cycling. Eur J Appl Physiol. 89(3–4):221–229. doi:10.1007/s00421-002-0784-0.
- Novak V, Hu K, Vyas M, Lipsitz LA. 2007. Cardiolocomotor coupling in young and elderly people. J Gerontol A Biol Sci Med Sci. 62(1):86–92. doi:10.1093/gerona/62.1.86.
- O'Rourke M, Avolio A. 1992. Improved cardiovascular performance with optimal entrainment between heart rate and step rate during running in humans. Coron Artery Dis. 3(3):863–869.
- Radaelli A, Valle F, Falcone C, Calciati A, Leuzzi S, Martinelli L, Goggi C, Viganò M, Finardi G, Bernardi L. 1996. Determinants of heart rate variability in heart transplanted subjects during physical exercise. Eur Heart J. 17(3):462–471. doi:10.1093/oxfordjournals.eurheartj.a014881.
- Smith SA, Mammen PP, Mitchell JH, Garry MG. 2003. Role of the exercise pressor reflex in rats with dilated cardiomyopathy. Circulation. 108(9):1126–1132. doi:10.1161/01.CIR.0000084538.40542.56.
- Smith SA, Mitchell JH, Naseem RH, Garry MG. 2005. Mechanoreflex mediates the exaggerated exercise pressor reflex in heart failure. Circulation. 112(15):2293–2300. doi:10.1161/CIRCULATIONAHA.105.566745.
- Takeuchi S, Nishida Y, Mizushima T. 2014. Effects of synchronization between cardiac and locomotor rhythms on oxygen pulse during walking. J Sports Sci Med. 13(4):881–887. doi:10.1589/jpts.27.1819.
- Takeuchi S, Nishida Y, Mizushima T. 2015. Evidence of an association between cardiac-locomotor synchronization and lower leg muscle blood perfusion during walking. J Phys Ther Sci. 27(6):1819–1822. doi:10.1589/jpts.27.1819.
- Takeuchi S, Yokoyama Y, Nishida Y. 2018. Influence of exercise capacity on cardiolocomotor coupling during walking in young people. Int J Sport Exc. Health Res. 2(1):83–87. doi:10.31254/sportmed.2102.
- Wang HJ, Li YL, Gao L, Zucker IH, Wang W. 2010. Alteration in skeletal muscle afferents in rats with chronic heart failure. J Physiol. 588(24):5033–5047. doi:10.1113/jphysiol.2010.199562.
- Witman MA, Ives SJ, Trinity JD, Groot HJ, Stehlik J, Richardson RS. 2015. Heart failure and movement-induced hemodynamics: partitioning the impact of central and peripheral dysfunction. Int J Cardiol. 178:232–238. doi:10.1016/j.ijcard.2014.10.044.