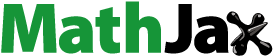
Abstract
Cardiovascular diseases are one of the leading causes of death globally. The primary pathological mechanism in myocardial infarction and heart failure is irreversible cardiomyocyte loss. The conventional knowledge is that mammals have a transient cardiac regenerative potential shortly after birth, but adult cardiomyocytes, as terminally differentiated cells, cannot regenerate. In contrast, some lower vertebrates, such as zebrafish, regenerate cardiac muscle throughout their lifetime. Zebrafish are extremely sensitive to cardiac injury and, within a short period, stimulate the proliferation of cardiomyocytes in numbers that fully regenerate to pre-injury levels; meanwhile, the initial scarring is gradually absorbed until there is little or no visible sign of fibrosis in the regenerating myocardium. The newborn mouse heart has the same remarkable regenerative potential before birth as one week after cardiac injury. The complex process of regeneration restores tissue structure through a series of cascade events, a coordinated process of cell proliferation, differentiation and dedifferentiation, and rearrangement of tissue morphogenesis. Understanding the ontogeny and development of cardiac regeneration and the molecular mechanisms of cardiac regeneration in zebrafish and mice is the cornerstone of regenerative biology and provides clues to investigate the cardiac repair process in adult mammals, resulting in extensive scarring rather than cardiomyocyte regeneration.
Introduction
Lower vertebrates, including salamanders and zebrafish, are promising as alternatives to replace damaged cardiac tissues (Oberpriller and Oberpriller Citation1971; Becker et al. Citation1974; Poss et al. Citation2002; Witman et al. Citation2011; Gamba et al. Citation2014), particularly in zebrafish, which efficiently regenerates the brain (Kroehne et al. Citation2011), retina (Vihtelic and Hyde Citation2000), fins (Johnson and Weston Citation1995), spinal cord (Becker et al. Citation1997), and heart (Poss et al. Citation2002), and other body parts. Mononuclear cardiomyocytes in neonatal mice retain their regenerative potential 7 days after birth, at a frequency comparable to lower vertebrates (Sorbini et al. Citation2023). Zebrafish have remarkable hearts, and despite damage to the heart muscle, they can fully regain heart function within 90 days (Nguyen et al. Citation2023). Patterson et al. (Patterson et al. Citation2017) investigated 120 inbred mouse strains and discovered remarkable frequency variation (>7-fold) in adult mononuclear cardiomyocytes. In contrast, while cardiomyocytes in adult mammals, including humans, can divide and proliferate, the response to infarction is fibrotic repair rather than regeneration because their proliferation frequency is low and insufficient to compensate for the massive cell loss (Lien et al. Citation2012). Simultaneously, an extracellular matrix (ECM) network replaces the damaged muscle tissue (Vujic et al. Citation2020), resulting in nonfunctional scar tissue. The scar that replaces the lost cardiomyocytes impairs the contractility of the remaining myocardium, resulting in cardiac pathogenic remodeling, including associated interstitial fibrosis. These events severely influence the overall contractility and mechanical environment of the cardiac tissue, resulting in impaired cardiac function, stagnation of the pulmonary and/or somatic circulation, continuous deterioration, and, in severe cases, heart failure (HF) and death (Porrello and Olson Citation2014; Sayers and Riley Citation2021). Many cardiac regeneration processes first reported in lower vertebrates have since been verified to play a role in cardiac regeneration in various species (Weinberger and Riley Citation2023). The lower cardiomyocyte (CM) proliferative capacity of adult mammals limits regeneration after cardiac damage; however, some animal species can readily regenerate lost myocardium through processes such as dedifferentiation, which unleashes cellular proliferative capacity (Bergmann et al. Citation2009, Citation2015; Cheng et al. Citation2022).
Cardiac regeneration is a broad term, and any regenerative strategy must overcome two major hurdles to restore function: replacing lost cardiomyocytes and coronary vessels and removing fibrotic tissue to restore cellular contractility (Sayers and Riley Citation2021; Sorbini et al. Citation2023). Current approaches to myocardium regeneration fall into three categories: renewal of existing mature cardiomyocytes by stimulating their dedifferentiation and proliferation, transdifferentiation of non-cardiomyocytes into cardiomyocytes via gene therapy, and delivery of a specific number of stem cell-derived cardiomyocytes via injection or cell-sheet construction techniques (Garbern and Lee Citation2022; Pezhouman et al. Citation2023). The use of cells as a potential cardiac therapeutic approach was originally described in 2001 for patients with severe HF following a myocardial infarction (MI) (Menasché et al. Citation2001). While a previous study at 5 months post-transplantation found no significant improvement in left ventricular systolic function and even increased risk of ventricular arrhythmias (Menasché et al. Citation2008), it sparked further interest in exploring cellular and molecular mechanisms for cardiac regeneration. This renewed focus aimed at restoring cardiac function through interventions targeting cardiomyocyte proliferation and post-injury angiogenesis. Despite investigations into skeletal myoblasts, bone marrow mononuclear cells (Tse et al. Citation2003), and bone marrow mesenchymal stem cells (MSCs) (Chen et al. Citation2004; Gupta et al. Citation2021), these approaches have yielded limited success due to the minimal, if any, observed improvements. Emerging evidence suggests that microRNA networks play a crucial role in regulating cardiomyocyte differentiation of MSCs (Azizidoost and Farzaneh Citation2023).
Myocardial regeneration in classical lower vertebrates: zebrafish
The heart of a zebrafish has a single atrium and single ventricle structure, a developmental process highly comparable to that of mammals, and the genetic and molecular tools, as well as extensive regenerative capacity in adulthood, make the zebrafish the most well-characterized model of cardiac regeneration to date (Azizidoost and Farzaneh Citation2023; Ross Stewart et al. Citation2022). Poss et al. published the first report of scarless regeneration of the zebrafish ventricular tip after resection in 2002 (Poss et al. Citation2002). This study revealed the robustness of cardiac regeneration in zebrafish. Several cardiac injury models for zebrafish have been developed recently, including the apical resection injury model (Lepilina et al. Citation2006; Jopling et al. Citation2010; Kikuchi et al. Citation2010), the cryoinjury model (González-Rosa et al. Citation2011; Schnabel et al. Citation2011), the cardiomyocyte genetic ablation model (Wang et al. Citation2011), and the hypoxia/reoxygenation model (Parente et al. Citation2013). All four models have robust myocardial regeneration but differ in timelines and regeneration processes.
Recent evidence shows a renewed interest in the relationship between the immune response and regeneration, and it is considered that cascade initiation of the immune response is an integral part of triggering myocardial regeneration (Lai et al. Citation2019; Farache Trajano and Smart Citation2021; Li et al. Citation2023). Acute inflammation promotes regeneration, with macrophages integral to cardiac regeneration (Yang et al. Citation2023). Clodronate liposome depletion in macrophages would result in impaired regeneration of the zebrafish heart (Lai et al. Citation2017; Yang et al. Citation2023). Macrophages act as a highly inflammatory cell population early in myocardial injury, releasing proinflammatory cytokines and matrix metalloproteinase (MMP)-9, which work with eosinophils to promote further cellular recruitment and ECM damage. Zebrafish investigations have demonstrated that eosinophils are rapidly recruited to the injured myocardium and are retained at high levels for 7–21 days after injury (Bevan et al. Citation2020). Reparative macrophages begin to release anti-inflammatory cytokines, for example, IL-17 and TFG-, as well as vascular endothelial growth factor (VEGF) upon entry into the proliferative phase promote angiogenesis (Zhao et al. Citation2010), which, along with activated fibroblasts, is implicated in scarring (Sommerfeld et al. Citation2019; Zhao et al. Citation2010). During this phase, the significantly increased neutrophils can promote phagocytosis by macrophages by releasing resolving factors and lipid mediators (Peiseler and Kubes Citation2019). Zebrafish have a stronger complement response compared to mammals, which enhances regenerative repair (Lai et al. Citation2019). Natarajan et al. (Citation2018) found that the complement receptor C5aR1 was activated in a zebrafish cardiac resection model to promote CM proliferation; conversely, inhibition of C5aR1 attenuated CM proliferation. While complement activation is considered beneficial, overstimulation of this pathway may be deleterious, as sustained complement activation will result in continuous inflammation and injury.
Apical resection injury model
Ventricular resection was the first model for investigating regeneration in the adult zebrafish heart (Azizidoost and Farzaneh Citation2023). Collagen and fibrin deposition occurs 2–9 days post-amputation (dpa) in zebrafish; abundant cell proliferation is observed after 14 dpa, and myocardium begins to rebuild, accompanied by a reduction in fibrin during this period, and the collagen/fibrin clot is subsequently replaced by healthy cardiomyocytes after 30–60 dpa (Poss et al. Citation2002; Raya et al. Citation2003). Unlike MI formation in humans, zebrafish do not form a permanent scar in the region of injury but rather undergo a sequential change, forming several clots, which are then replaced by fibrous tissue. Eventually, the fibrous tissue is entirely replaced by neonatal cardiomyocytes, forming a new layer of dense myocardium to complete the regenerative process, with complete restoration of electrical conduction function (Kikuchi et al. Citation2010). Although ventricular resection model does not fully simulate the cardiac pathological process of MI in humans, it is worth emphasizing that it demonstrates the existence of robust myocardial regeneration and provides methods for identifying the relevant acting genes that form this process, laying the groundwork for the development of the models of injury described in the subsequent sections.
Cryoinjury model
Cryoinjury, known as low-temperature injury, is simulated by pressing a liquid nitrogen-immersed probe against the ventricular wall (González-Rosa et al. Citation2011; Schnabel et al. Citation2011). Cryoinjury triggered a significant inflammatory response and cell death, forming 15–18% of the scar area 3–4 days post cryoinjury (dpci). Significant collagen deposition was noted after 7 dpci, and complete replacement of the scar tissue by neoplastic cardiac tissue occurred after 60–120 dpci, with a newly generated layer of cardiomyocytes thicker than that of the uninjured ventricle and its contractile capacity fully restored (Schnabel et al. Citation2011). The degree of cell death differs significantly between the ventricular resection and cryoinjury models, and cellular debris representing necrosis is also detected following cryoinjury. Cryoinjury shows that early macrophage infiltration, rapid formation of neovascular sprouts in the trauma, and transitory fibrosis are required for robust CM proliferation, a critical feature of this model (Marín-Juez et al. Citation2016; Sánchez-Iranzo et al. Citation2018; Yang et al. Citation2023). The cryoinjury model serves as a comparable MI model and demonstrates the robust regenerative capacity of zebrafish.
Genetic ablation
As a model with the dual advantages of excising specific cell types and determining the extent to which they contribute to repairing the myocardium, gene-specific ablation undoubtedly assists in identifying the specific response of CMs to injury and the different cell types that influence their proliferation. Curado et al. (Citation2007) generated a Tg (myl7: CFP-NTR) transgenic zebrafish line in CMs expressing a nitroreductase (NTR) enzyme that transforms the drug precursor metronidazole (Mtz) into a cytotoxic agent and that causes cell death for the attempted ablation of cardiomyocytes from zebrafish larvae. The method could reveal the response of the injured heart by removing Mtz after the initial treatment. The results showed that with the progressive elimination of cardiac edema, the morphology, structure, and contractile function of the juvenile fish heart were fully restored (Curado et al. Citation2007). A follow-up study by Zhang et al. (Citation2013) found that Mtz therapy after ventricular cardiomyocyte ablation by specifically ablating ventricular cardiomyocytes could transdifferentiate atrial cardiomyocytes to ventricular cardiomyocytes. Cardiomyocyte plasticity was revealed as a potential approach to repairing the injured heart through precision cell ablation. Wang et al. (Citation2011) developed a double transgenic cell line with 4-hydroxytamoxifen (4-HT)-inducible Cre recombinase (CreER) and bactin2: loxp-mCherry-STOP-loxp-DTA, respectively, to further investigate whether the adult zebrafish heart regenerates after cardiomyocyte-specific ablation. The first transgenic line was restricted to CMs by the cmlc2 promoter, whereas the second transgenic line targeted expression of the cytotoxin diphtheria toxin A (DTA) after 4-HT injection. Zebrafish cardiomyocytes underwent apoptosis after 3–5 days postinjury (dpi), whereas 42% of cardiomyocytes were detected to be proliferating by 7 dpi, demonstrating a robust regenerative response (Wang et al. Citation2011). Similarly, cytotoxicity by inducing reactive oxygen species (ROS) in cardiomyocytes for specific ablation has confirmed that cardiomyocytes from adult hearts can regenerate after genetic ablation (Wang et al. Citation2011; He et al. Citation2016).
Hypoxia/Reoxygenation model
A hypoxia/reoxygenation model was also included in the simulated mammalian MI model after coronary artery occlusion (Parente et al. Citation2013; Azizidoost and Farzaneh Citation2023). Additionally, oxidative stress in the heart peaked at 2 h, sporadic inflammatory cells emerged at 4 h, and inflammatory cell infiltration peaked at 6 h. The mRNA of the HIF-1α-dependent gene vascular endothelial growth factor Aa (Vegfaa) increased and plateaued at 6 h, with a peak 2.5-fold that of the control. This was followed by a rapid decrease in neutrophil and macrophage infiltration, which reverted to baseline levels within 24 h. Hypoxia/reoxygenation markedly increased apoptosis and necrosis in adult zebrafish cardiomyocytes, with cell death peaking at 14–18 h. Cardiomyocyte proliferation began at 18–24 h, peaked at 3–7 days, and returned to baseline after 30 days. Cardiomyocytes then began to proliferate. The number of cardiomyocytes normalized thirty days later, with no evidence of collagen deposition. Two-dimensional echocardiography assessment of cardiac function revealed that ventricular function returned to baseline (Parente et al. Citation2013). This model more closely mimics reperfusion injury in mammals than other cardiac injury models.
Classical mammalian myocardial regeneration
Rodent
A comparable regenerative response to ventriculotomy was described in neonatal mice barely a decade after zebrafish heart regeneration was reported (Porrello et al. Citation2011). Studies on proliferating cardiomyocytes between species have revealed that mononuclear cardiomyocytes in neonatal mice maintain their regenerative potential at a frequency comparable to that of lower vertebrates during the first 7 days of life (Walsh et al. Citation2010). Mammals have a remarkable regenerative capacity following cardiac injury during fetal development; however, this capacity lasts for a short period after birth. Porrello et al. extended regeneration studies to mammalian models and modeled ventricular resection in 1-day-old neonatal mice after discovering scarless cardiac regeneration in zebrafish (Porrello et al. Citation2011). They found an increased CM proliferation, reduced fibrosis, and new myocardium, similar to zebrafish. However, regenerative capacity was lost in 7-day-old mice. Neonatal mice retain substantial myocardial regenerative capacity after apical resection (Porrello et al. Citation2011) or coronary artery ligation (Porrello et al. Citation2013) in the first week of life, similar to neonatal rats. Lam and Sadek (Citation2018) found the first 2–3 days after birth the potential optimal window for myocardial regeneration. More precise genetic lineage tracing lays a reliable technical foundation for in-depth cardiac development, stem cells, and regeneration research. Genetic lineage tracing is an approach for monitoring and presenting cell fates using site-specific recombination systems to highlight the phenomena of self-renewal, differentiation, and transdifferentiation of specific cell types in development, disease, and regeneration (Liu et al. Citation2020; Wang et al. Citation2023). Senyo et al. (Citation2013) examined mice CMs using genetic lineage tracing and Multi-isotope Imaging Mass Spectrometry (MIMS) and found that new cardiomyocytes developed from preexisting cardiomyocytes during normal myocardial homeostasis. They further revealed that cardiomyogenesis occurred at a low rate (0.76%/year) during normal aging through the division of preexisting CMs and that this rate decreases with age. Many studies have focused on identifying the genes and pathways that reactivate the de novo myocardial regeneration program after adult cardiac injury since the discovery of the transient regenerative capacity of the neonatal mouse heart. A study that comparatively analyzed the transcriptomes of neonatal and adult cardiomyocytes found that the transcriptional correlation between neonatal cardiomyocytes and endothelial cells was higher than that of adult cardiomyocytes (Quaife-Ryan et al. Citation2017). Most transcriptional changes in all cardiac cell types begin with developmental maturation from the neonatal stage to adulthood rather than specific activation of a unique regenerative gene program. This finding implies that neonatal cardiomyocytes are programed to maintain an active cell cycle program from the outset, allowing them to proliferate and regenerate after injury. In the absence of injury, adult mammals maintain cardiac homeostasis by gradually renewing cardiomyocytes at a rate of approximately 1% per year, which decreases to 0.5% or less with age (Mollova et al. Citation2013; Senyo et al. Citation2013; Cheng et al. Citation2022). This begs the issue of whether the adult heart retains a subpopulation of CMs with a permissive developmental program that permits the heart to maintain 1% cardiomyocyte renewal every year.
Human
Clinical evidence shows that human neonates have a higher myocardial regeneration capacity after MI than adults (Haubner et al. Citation2016). Macmahon found early mitotic nuclei in the myocardial fibers of a 6-year-old child who died of diphtheria in 1937 (Macmahon Citation1937). Case reports of human corrective cardiac surgery (Fratz et al. Citation2011) and neonatal MI (Haubner et al. Citation2012) revealed complete myocardial regeneration. These findings indicate a window during which the neonatal heart can be highly regenerative. Clinical case reports have indicated restoration of ventricular function and no detectable scarring of the myocardium after cardiac surgery for congenital heart disease (Fratz et al. Citation2011; Haubner et al. Citation2016) and restoration of blood flow in infants born with MI (Tsang et al. Citation2009). Autopsy examinations of infant hearts have also revealed evidence of regeneration (Macmahon Citation1937). In contrast to the inability of the adult mammalian heart to regenerate, the transient regenerative capacity of the neonatal heart is maintained primarily by the repopulation of preexisting cardiomyocytes, accompanied by expansion of cardiac fibroblasts and changes in the extracellular matrix composition. Whether and how these changes affect cell proliferation and cardiac regeneration warrants further investigation. Feng et al. (Citation2023) recently discovered that Versican, a cardiac fibroblast-derived extracellular matrix component, plays a role in upregulating and promoting cardiomyocyte proliferation after neonatal myocardial injury.
Mechanisms related to myocardial regeneration
Pre-existing cardiomyocytes (CMs) de-differentiate and proliferate to produce new CMs
The proliferation of cardiomyocytes (CMs) is thought to be part of the damage response. Genetic lineage tracing of zebrafish cardiomyocytes revealed that the new CMs are derived from pre-existing CMs rather than progenitor cell populations (Jopling et al. Citation2010; Kikuchi et al. Citation2010; Gupta and Poss Citation2012). These cardiomyocytes regenerate new cardiomyocytes by a ‘de-differentiation-proliferation-re-differentiation’ process, and immature CMs are often more effective in promoting regeneration (Sande-Melón et al. Citation2019; Tang et al. Citation2019) (Figure ). Zebrafish cardiac regeneration may require dedifferentiation of adult CMs. Zebrafish cardiac regeneration may necessitate dedifferentiation of adult CMs, which is the process by which differentiated cells, when subjected to injury or ex vivo culture, resume dividing, and the cells change their original state of differentiation, lose their original structure and function, and become cells with undifferentiated characteristics, also known as dedifferentiation (Xiao et al. Citation2022). This type of dedifferentiation is not a global process but is localized near the injury.
Figure 1. Four pathways of cardiac regeneration of the zebrafish heart.
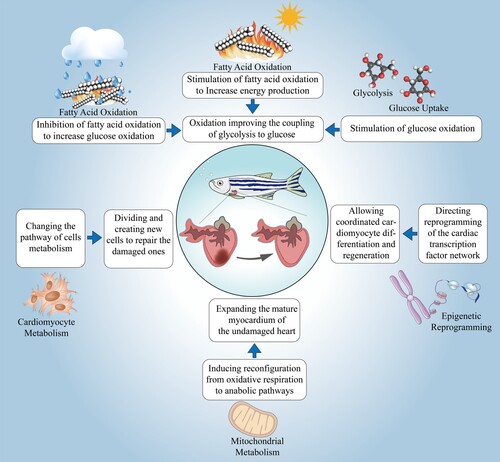
Cardiomyocytes in zebrafish regulate their differentiation state in response to cardiac injury by upregulating the regulatory sequence gata4 (Kikuchi et al. Citation2010; Brezitski et al. Citation2021) and other cardiac transcription factors such as tbx20 and nkx2.5 (Lepilina et al. Citation2006). The bone morphogenetic protein BMP signaling regulates the expression of the above transcription factors (Brezitski et al. Citation2021; de Pater et al. Citation2012), which is activated in proliferating cardiomyocytes after injury to promote cardiomyocyte dedifferentiation. Inhibition of BMP signaling, gata4 signaling, or myocardial NF-κB restricts the proliferation and regeneration of zebrafish CM (Gupta et al. Citation2013; Karra et al. Citation2015; Wu et al. Citation2016). This also applies to the results of cardiac fate localization techniques in neonatal and adult mice (Wang et al. Citation2017). Cmlc2 was extensively employed to lineage trace cardiomyocytes because it is expressed in all cardiomyocytes. In this view, ∼95% of CMs were labeled, and the ∼5% unlabeled would be due to the low efficiency of tamoxifen induction. Jopling et al. (Citation2010) and Kikuchi et al. (Citation2010) found in two independent studies that inducible CreER expression was driven by the cardiac myosin light chain 2 (cmlc2) promoter sequence, that all Cmlc2-expressing cardiomyocytes were labeled with EGFP expression before the cardiac injury, and that ventricular resection 30 days after ventricular resection, most regenerating tissues were labeled for EGFP expression. Appropriate contractility and fluid dynamics are crucial for normal heart development during zebrafish embryogenesis (Auman et al. Citation2007). Chen et al. (Citation2008) found that cmlc2-deficient embryos had fewer cardiomyocytes, and cmlc2 mutants had decreased ventricular volume and failed to dilate the heart. The changes in ventricular volume and lack of cellular response may be an indirect consequence of the defective contractility of the myocardium. The low rate of cardiomyocyte mitosis in mammals can be traced to the division of preexisting CMs, which are more likely to be mononuclear and diploid (Porrello et al. Citation2011; Senyo et al. Citation2013; Ali et al. Citation2014; Elia et al. Citation2023). Senyo et al. (Senyo et al. Citation2013) investigated cardiomyocyte turnover using MIMS and discovered that, in contrast to the normal aging process, where cardiomyocytes divide at a low rate, there was a four-fold increase in cardiomyocytes in the border region of myocardial injury, with polyploidy and multinucleation arising. These data suggest that preexisting CMs constitute a significant source of cardiomyocytes that can be replenished during normal mammalian myocardial homeostasis and after myocardial injury.
Cardiomyocyte cell-cycle re-entry vs. Cardiomyocyte cell-cycle exit
The adult mammalian heart grows via cardiomyocyte division rather than hypertrophic growth before birth (Soonpaa et al. Citation1996). Consistent with retained proliferative capacity, the neonatal mammalian heart consists primarily of mononuclear diploid cardiomyocytes and approximately 30% binucleated cardiomyocytes during early postnatal development; as development proceeds and proliferative capacity is lost, this composition changes, and many cardiomyocytes become binucleated or multinucleated and polyploid (Derks and Bergmann Citation2020). The degree to which mature cardiomyocytes are multinucleated and polyploid varies widely among mammalian species. Cardiomyocyte ploidy increases dynamically during postnatal development in humans, with the majority of cardiomyocytes becoming mononuclear tetraploid (approximately 66%) (Mollova et al. Citation2013; Cheng et al. Citation2022), whereas nearly 80% of mouse and more than 90% of porcine adult cardiomyocytes are polyploid (Derks and Bergmann Citation2020). Evidence shows that the fraction of mononuclear diploid CMs in the adult heart varies across genetic backgrounds, fluctuates significantly in different mouse strains, and positively correlates with regenerative potential after cardiac injury (Patterson et al. Citation2017). Although the degree of cardiomyocyte polyploidization varies between mammals, all mammals investigated exhibit cardiomyocyte cycle exits shortly after birth (Mollova et al. Citation2013). This permanent exit from the cell cycle of most postnatal cardiomyocytes has been linked to widespread alterations in the epigenome and transcriptome, as well as an increased abundance of multinucleated and polyploid cardiomyocytes (Walsh et al. Citation2010; Bergmann et al. Citation2015; Paige et al. Citation2015). In addition, perinatal oxidative stress and the resultant DNA damage at high oxygen levels may be major causes of cardiomyocyte cycle exit (Puente et al. Citation2014). González-Rosa et al. found that diploid cardiomyocytes regenerate the heart preferentially after ventriculotomy in zebrafish using a combination of genetic lineage tracing and genetic approaches to induce polyploidization (González-Rosa et al. Citation2018).
Overcoming the polyploidy barrier may be one of the most effective therapies for cardiac regeneration. It is crucial to highlight that ploidy is not necessarily connected to regenerative capacity, and exceeding the polyploidy threshold impairs cardiac regeneration. In zebrafish hearts, more than 45% of polypoid CMs form a large scar after ventricular resection, and the scar induces polyploidy, establishing a threshold for cardiac regeneration (Brezitski et al. Citation2021). In contrast, in many adult mammals, the tendency towards polyploidization renders the regenerative response after cardiac injury less efficient (Adler et al. Citation1996). Several regulatory factors regulate polyploidy up and down this pathway; for example, viral overexpression of Lmnb2 increases cardiomyocyte proliferation and regeneration after cryoinjury in zebrafish. Conversely, genetic deletion of the nuclear filament protein Lmnb2 in neonatal mouse CMs induces polyploidy and impairs regeneration (Han et al. Citation2020). Cell cycle progression is controlled by systems of proteins that each function at different cell cycle stages to coordinate intracellular biochemical events required for cell division (Brooks et al. Citation1998; Regula et al. Citation2004). More reports have revealed that cardiomyocyte cycle re-entry can be achieved by altering specific cell cycle regulatory proteins. Protein kinase C-α (PKC-α) induced the cell cycle re-entry selectively of neonatal rat ventricular cardiomyocytes, which was associated with the inhibition of runt-related transcription factor 1 (Runx1) and p16 cell cycle inhibitor (CDKN2a) mRNA upregulation (Kebbe et al. Citation2023). Du et al. (Citation2022) found that five small molecules (5SM) containing B3 (phenylephrine), B8 (baricitinib), B10 (harmine), B13 (vo-ohpic trihydrate), and C1 (AZD3965) effectively induced CM cell-cycle re-entry and division after MI in adult rats and promoted CM proliferation and heart regeneration.
MicroRNA networks during cardiomyocyte regeneration
Mature microRNAs (miRNAs) are short, naturally occurring non-coding RNA molecules 18–25 nucleotides in length, and each miRNA is in a many-to-many regulate-and-be-regulated relationship with their target genes based on the mapping relationship, forming a complex regulatory network (Mishra et al. Citation2009; Das and Halushka Citation2015). MiRNAs are important (pathologic) physiological regulators of the cardiovascular system (Condorelli et al. Citation2014). There is mounting evidence that miRNA networks that regulate cardiomyocyte proliferation influence post-transcriptional regulation of cardiac gene expression (Liu and Olson Citation2010; Azizidoost and Farzaneh Citation2023). Six miRNAs (miR-1, miR-21, miR-24, miR-126, miR-133a, and miR-499) have been described as systemically expressed in ischemic heart disease or regulate cardiac regeneration via angiogenesis and apoptosis (Liu and Olson Citation2010; Traxler et al. Citation2023). Gao et al. (Citation2019) discovered that miR-19a and miR-19b play a cardioprotective functional role in MI mice. miR-19a/19b delivery using adeno-associated virus AAV enhances cardiomyocyte proliferation, stimulates myocardial regeneration after MI and exerts critical cardioprotective effects. Other miRNAs, including miR-17–92 cluster, miR-222 cluster, and miR-214 cluster, have been reported to contribute to cardiac repair in vivo (Aurora et al. Citation2012; Chen et al. Citation2013; Liu et al. Citation2015); inhibition of the miR-15 family increases cardiomyocyte proliferation and improves cardiac function after MI (Porrello et al. Citation2013). Borden et al. found that after MI in mice, ectopic transient expression of miR-294 promoted cell cycle re-entry, and treatment showed significant cardiac repair (Borden et al. Citation2019).
The default response of the adult heart to overload or injury is dominated by cardiomyocyte hypertrophy (a form of cell growth without cell division), one of the major contributors to heart failure in later stage (Brodsky et al. Citation1994; Liu et al. Citation2022; Ye et al. Citation2023). This is a severe clinical condition that causes significant morbidity and hospitalization. Conserved epigenetic molecular programs can boost myocardium regeneration, and evolutionarily conserved microRNA clusters regulate the balance between cell proliferation and hypertrophy, efficiently stimulating cardiac regeneration. Raso et al. (Citation2021) found that the miR-106b∼25 cluster was highly expressed in early postnatal myocardium but decreased in adulthood and that it facilitated the transition to cell cycle arrest and cardiac hypertrophy under overload. Heart failure causes eccentric remodeling, which functions through inhibition of the miR-106b∼25 cluster and the pro-hypertrophic transcription factors Hand2 and Mef2d. Viral gene transfection of miR-106b∼25 promotes regeneration of a significant proportion of adult myocardium after ischemic injury using the regulatory potential of miR-106b∼25 on cardiomyocyte proliferation and hypertrophy. MiR-25 has been revealed to promote cardiomyocyte proliferation in zebrafish by inhibiting the cell cycle inhibitor Cdknc1 and the tumor suppressor Lats2 (Raso et al. Citation2021; Wang et al. Citation2020). In addition, Shao et al. (Citation2020) discovered the miR-377/peroxisome proliferator-activated receptor γ (PPARγ) axis using the cardiac hypertrophy mice model. MiR-377 is highly expressed in hypertrophic cardiomyocytes, and its expression negatively correlates with autophagy in hypertrophic cardiomyocytes. These findings suggest that miRNAs play a specific role in cardiomyocyte development and regeneration.
Major signaling pathways regulating cardiomyocyte proliferation
Hippo/Yap signaling pathway
The common endogenous cardiomyocyte proliferation regulatory pathways include the Hippo-YAP signaling pathway, neuromodulin, and the Wnt--catenin signaling pathway: the Hippo/Yap pathway has been intensively investigated in cardiac regeneration (Heallen et al. Citation2013). The Hippo pathway comprises a series of receptors and kinases that promote phosphorylation of the transcriptional co-activator Yes-associated protein (YAP) (Zhao et al. Citation2011) and transcriptional co-activators with PDZ-binding motifs (TAZs). YAP/TAZ remains transcriptionally active until phosphorylation; at this point, YAP entry into the nucleus activates gene expression by interacting with DNA-binding transcription factors such as TEAD, SMAD1, RUNX4, and TBX5. YAP enters the nucleus and promotes gene expression by interacting with DNA-binding transcription factors such as TEAD, SMAD1, RUNX4, and TBX5. Zebrafish regulate the Hippo/Yap signaling pathway to promote normal cardiovascular development and cardiac regeneration after injury (Riley et al. Citation2022). Yap is a critical downstream effector of the Hippo pathway regulating CM proliferation and a component of the IGF, Wnt, and Hippo signaling pathways related to cardiac growth and development program coupling (Zhao et al. Citation2011). YAP is required for fetal cardiomyocyte proliferation, and activated YAP drives adult mouse cardiomyocyte proliferation (Del Re et al. Citation2013). The phosphoinositide 3-kinase (PI3 K)-AKT pathway activation downstream of YAP has increased mouse cardiomyocyte survival, proliferation, and physiological hypertrophy (Xin et al. Citation2011, Citation2013). This finding has been further validated by Lin et al. (Lin et al. Citation2015), whose data suggest that Pik3cb is the direct target of YAP, which is required for mitogenic activity, and its activation is sufficient to drive adult mouse cardiomyocyte proliferation and survival. The mutant YAP5SA of the Hippo pathway was recently implicated in cardiomyocyte proliferation and growth (Xiao et al. Citation2022). Monroe et al. (Citation2019) found that YAP5SA increased chromatin accessibility and fetal mouse gene expression in the mouse model, overexpressing active YAP (YAP5SA), partially reprograming adult mouse CMs to a more fetal and proliferative state. Llgl1 regulates zebrafish cardiac development by mediating Yap stability in cardiomyocytes (Flinn et al. Citation2020). Targeting the Hippo-YAP pathway can increase CM proliferation, and deletion of the YAP gene leads to cardiac hypoplasia and embryonic death, whereas YAP overexpression in the embryo leads to cardiomyocyte overproliferation and grossly disproportionate ventricular and death (Xin et al. Citation2011, Citation2013). Targeting YAP stimulation may modulate maladaptive responses; YAP activation during pressure overload induces cardiomyocyte dedifferentiation and heart failure (Ikeda et al. Citation2019).
Wnt signaling
Wnt signaling regulates stem cell pluripotent differentiation, organ development, and regeneration (Sogo et al. Citation2023; Zimmerman et al. Citation2012). Natural regenerative response to cardiac injury includes the induction of the expression of multicomponent Wnt inhibitors comparable to Dkk3/sFrp1 in epicardium/endocardium and Dkk1/sFrp2 in the myocardium, as well as Notum1b/Wif1 in the endocardium (Zhao et al. Citation2019), which block Wnt signaling and allow for de-differentiation and proliferation of pre-existing CM. Injured cardiac tissue induces p21-activated kinase (PAK2) during regeneration, which promotes CM dedifferentiation and proliferation by increasing -catenin Ser 675 phosphorylation and stability. Pharmacological studies have demonstrated that the Wnt inhibitors Wif1 and Notum1b are up-regulated in zebrafish-damaged myocardium and that inhibiting Wnt signaling and decreasing nuclear
-catenin enhance cardiac regeneration (Zhao et al. Citation2019; Xie et al. Citation2020). Zhao et al. found that multiple Wnt repressor genes (secreted proteins dkk1b, dkk2, and dkk3b, and the secreted frizzled-associated proteins sfrp1a, sfrp1b, and sfrp2) were induced and secreted in response to ventricular injury after ventricular apical resection of the 6-month-old zebrafish. In contrast, Wnt ligand genes (wnt2ba, wnt4a, wnt6b, and wnt8a) were expressed at lower levels during regeneration. Further immunostaining analysis detected sFrp1 and Dkk3 in epicardial cells in the periventricular and wound regions (Zhao et al. Citation2019).
The Wnt/-catenin signaling pathway exhibits dual effects during cardiac development. Unlike inhibition of Wnt signaling, which facilitates cardiac wound healing and post-injury functional recovery, Wnt/
-catenin exerts opposite effects in the adult heart, and upregulation of Wnt/
-catenin activity does not suppress heart regeneration. Instead, inhibition of Wnt/
-catenin signaling suppresses cardiomyocyte proliferation (Bertozzi et al. Citation2022). Exposure of zebrafish to cryoinjury lead to the activation of the Wnt/
-catenin signaling pathway, especially in cardiomyocytes near the wound border. Further analysis of axin2 levels in wound border cardiomyocytes at different time points showed that the Wnt/
-catenin signaling was activated at all stages of cardiomyocyte regeneration compared to sham injury. It has also been shown that the Wnt/
-catenin signaling promotes cardiomyocyte proliferation, scar maturation and absorption, thereby enhancing the regeneration of the injured heart in zebrafish. Heat shock-induced overexpression of Axin1, the intracellular pathway inhibitor, suppressed the Wnt/
-catenin signaling in regenerating zebrafish hearts and inhibited cryoinjured cardiomyocyte cycle activity (Bertozzi et al. Citation2022). The co-receptor for Wnt signaling, low-density lipoprotein receptor-related protein 5 (LRP5), play an essential function in the regeneration of the neonatal heart. Zhou et al. (Citation2022) showed that overexpression of LRP5 promoted cardiomyocyte proliferation in neonatal mouse, whereas specific deletion of LRP5 disrupted regeneration of the myocardium following injury, and aggravated fibrotic scarring and cardiac dysfunction. Deficiency of the Wnt co-receptor low-density lipoprotein receptor-related protein 6 (LRP6), which is required for embryonic heart development, enhanced the CM cycle activity in neonatal, juvenile, and adult mice, and triggered a strong regenerative response after MI, leading to a reduction in the size of infarcted area and improvement in left ventricular contractile function (Wu et al. Citation2021).
Hedgehog signaling pathway
Hedgehog (Hh) signaling plays a critical in normal tissue development, including organ formation, regulation of cell differentiation and cell proliferation (Jia and Jiang Citation2006). For example, Hh signaling regulated the specification and differentiation of endocardial progenitors in zebrafish (Wong et al. Citation2012). However, the role of the Hh signaling pathway in adult cardiac homeostasis has not been clarified partly due to the low expression of this protein in adult cardiomyocytes. A previous study indicated that ischemic cardiac injury can reactivate the Hh pathway to exert cardioprotective effects (Kusano et al. Citation2005). Elsewhere, Singh et al. (Citation2018) compared apical resection models in salamanders and mice and identified a novel HH-Gli1-Mycn gene network that regulates cardiomyocyte proliferation and prolongs the window of cardiac regeneration. Feng et al. (Citation2020) reported that simvastatin treatment relieved myocardial ischemia/reperfusion injury in rats via the Hh signaling pathway. As a key nuclear executor at the distal end of the hedgehog signaling, the transcription factor glioma-associated oncogene 1 (Gli1) modulates diverse biological processes such as cell differentiation, proliferation, and apoptosis, and is involved in the occurrence and progression of myocardial fibrosis (Robbins et al. Citation2012; Wang et al. Citation2018). Unlike the mammalian situation, the fibrosis process in zebrafish is transient. Sánchez-Iranzo et al. (Citation2018) found that fibroblasts were not completely cleared but were inactivated during fibrosis regression. Interestingly, even after clearing scar tissue, some ECM-producing cells remain. This suggests that myocardial regeneration and fibrosis are not opposing forces, but rather co-existing processes where ECM-playing cells have a crucial role. Hh signaling accelerates the CMs proliferation during zebrafish embryo regeneration. Choi et al. (Citation2013) found that the number of proliferating cardiomyocytes in embryos increased by 60% after 24 h of treatment with an agonist of the Hh pathway. This pathway also regulates coronary artery development and vasculogenesis. Targeting the Hh signaling pathway promotes coronary neoangiogenesis (Lavine et al. Citation2008), reduces cardiac fibrosis (Kusano et al. Citation2005), suggesting that Hh signaling may become a new therapeutic target for cardiac repair and regeneration.
Notch signaling pathway
The Notch pathway is a highly conserved signaling pathway known to drive the growth and development in organisms through cell-to-cell interactions, and has precise regulatory functions in processes such as cardiomyocyte maturation, proliferation (Samsa et al. Citation2015; D'Amato et al. Citation2016), and cardioprotective effect (Kachanova et al. Citation2022; Samsa et al. Citation2015), suggesting that it may participate in cardiac regeneration. In an early cardiac regeneration study, Raya et al. (Citation2003) found that the expression of notch1b and deltaC were upregulated in pericytes of myogenic fibers of adult zebrafish hearts at 1 dpa. In zebrafish larvae, endocardial Notch signaling regulates CMs transdifferentiation from atria to ventricles (Zhang et al. Citation2013).
Zhao et al. (Citation2014, Citation2019) investigated the intricate mechanisms of Notch signaling in cardiac regeneration. They used a transgenic heat shock line, Tg (hsp70:dn-Maml), to express a dominant-negative protein that disrupts Notch signaling, and found that the transgenic hearts exhibited reduced Mef2c+/Pcna+ cardiomyocytes at 7 dpa and significant fibrosis at 30 dpa (Zhao et al. Citation2014). These results indicate that the Notch signaling pathway play an essential role in the regulation of cardiomyocyte proliferation. In a follow-up study, it was found that notch deficiency failed to induce the secretion of Wnt antagonists Wif1 and Notum1b (Zhao et al. Citation2019). Wnt signaling suppresses cardiac regeneration and Notch signaling promotes zebrafish CM proliferation by inhibiting myocardial Wnt signaling. The Notch signaling pathway has been implicated in mammalian heart development. The Notch 1 signaling pathway, one of four homologous isoforms in mammals, is the key factor that modulates the proliferation and differentiation of cardiac stem cells (Zhou and Liu Citation2014). Urbanek et al. (Citation2010) reported that overexpression of Notch1 intracellular domain (N1ICD) in neonatal mouse cardiac stem cells (CSCs) enhanced the proportion of transit-amplifying myocytes and that blockade of Notch signaling by a gamma-secretase inhibitor (GSI1) caused a 62% reduction in amplifying myocytes and a 54% reduction in myocyte number. Findings from previous investigations have indicated that high levels of Notch 1 and the Notch ligand Jagged 1 in neonatal rats not only protect immature CMs from apoptosis but also significantly promote their proliferation (Collesi et al. Citation2008). Notch signaling also influences the development of cardiac mesoderm. Del Monte et al. (Citation2011) found that the Notch signaling elements were differentially activated during the proepicardial-epicardial-coronary transition and play crucial roles in coronary vessel wall maturation. The Notch ligand Delta-like 4(Dll4)/Notch signaling enhances the angiogenesis process under ischemic conditions by coordinating the interplay between inflammation and angiogenesis (Al Haj Zen et al. Citation2010). Jabs et al. (Citation2018) found that Notch signaling regulated fatty acid transport across the endothelium and inhibited cardiac angiogenesis in an adult mice model. The Notch signaling participates in the regulation of CMs and mesenchymal stromal cells. By regulating adult cardiac fibrosis, the Notch signaling can switch cardiac regenerative repair from pro-fibrotic default pathway to pro-cardiogenic pathway (Nemir et al. Citation2014).
Mitogen-activated protein kinase signaling
Mitogen-activated protein kinases (MAPKs), among the oldest signal transduction pathways, are protein serine/threonine kinases involved in several physiological processes (Widmann et al. Citation1999). Activation of the MAPK signaling promotes phosphorylation, cell proliferation, growth and survival processes (Cargnello and Roux Citation2011). Each type of MAPK protein has distincts effects on zebrafish cell proliferation. For example, the isoforms Erk1/2 promoted cell cycle and proliferation (Liu and Zhong Citation2017), whereas p38α MAPK inhibited proliferation (Jopling et al. Citation2012). Missinato et al. (Citation2018) also reported that the Erk pathway was involved in cardiac regeneration, where genetic disruption and chemical inhibition of dual-specificity phosphatase 6 (Dusp6) enhanced the cardiac regeneration process. Wang et al. (Citation2005) also found that the p38 MAPK pathway regulated apoptosis of cardiomyocytes, triggered activation of caspase-1, caspase-3, and caspase-11 as well as the production of TNF-α, IL-I, and IL-6. Inhibition of p38 MAPK exerted beneficial effects on myocardial ischemic diseases. Multiple intracellular signaling pathways, including the Ras-MAPK pathway, play a role in the occurrence of cardiac hypertrophy (Clerk et al. Citation2007). In a transgenic mouse model of Ras activation targeting the heart, activation of Ras promoted the downstream ERK1/2 MAPK pathway, upregulated the expression of atrial naturetic factor (ANF), and induced pathological cardiomyocyte hypertrophy which progressed to congestive heart failure (CHF) (Wei et al. Citation2011). An overview of major signaling pathways regulating cardiomyocyte proliferation are presented in Table .
Table 1. Major signaling pathways regulating cardiomyocyte proliferation
Exogenous therapeutic strategies for myocardial regeneration
Cardiosphere-derived cells
Cardiosphere-derived cells (CDCs) originate from heterogeneous cell populations (including c-kit+, CD31/CD34+, and CD90+ cell subsets) in the in vitro culture and expansion of cells isolated from endomyocardial biopsy from patients (Davis et al. Citation2009; Wei et al. Citation2011). Cells with cardiomyogenic potential have been shown to act primarily through paracrine mechanisms (López et al. Citation2020). The therapeutic value of CDCs varies across different animal models (Malliaras et al. Citation2012; Kasai-Brunswick et al. Citation2017). A recent study by Guo et al. isolated CDCs directly from the epicardium of adult mouse hearts overexpressing T-box transcription factor 18 (Tbx18) and demonstrated high pluripotent differentiation capacity in vitro (Guo et al. Citation2021). Although the ALLSTAR clinical trial did not show a reduction in scar size as did the CADUCEUS trial, left ventricular end-diastolic volume and NT-proBNP were improved following CDC administration (Malliaras et al. Citation2014; Makkar et al. Citation2020). Application of the CDC subpopulation of c-kit+ cardiac-derived cells combined with mesenchymal stem cells (MSCs) to treat patients with ischemic cardiomyopathy, led to a significant improvement of the quality-of-life scores of the patients in the CONCERT-HF trial at 12 months, although there was no significant improvement in left ventricular ejection fraction (LVEF) or left ventricular volume (Bolli et al. Citation2021).
Sca-1+ cells
Previous studies showed that Sca-1+ cells are the source of new CMs in the adult mouse heart (Uchida et al. Citation2013; Noseda et al. Citation2015), although the percentage of Sca-1+ cells may have been overestimated. Evidence from multiple studies show that Sca-1+ cells themselves do not contribute significantly to CMs, but rather are precursors of endothelial cells, and that Sca-1+ cardiac stem cells cannot transdifferentiate into cardiomyocytes (Noseda et al. Citation2015; Soonpaa et al. Citation2018; Zhang et al. Citation2018). Although several genetic lineage tracing studies have ruled out the contribution of Sca-1+ progenitors to newly regenerated cardiomyocytes, Sca-1+ progenitors contributed more to the formation of vascular endothelial cells (Soonpaa et al. Citation2018; Vagnozzi et al. Citation2018). Researchers constructed models of aortic constriction-induced fibrosis and femoral artery injury in tamoxifen-induced Sca1-2A-CreER; R26-GFP mice, and illustrated that adult Sca-1+ endothelial progenitor cells can significantly proliferate and form more vascular endothelial cells in following injury stress, contributing to the repair of tissue injury through neovascularization (Tang et al. Citation2021).
Pluripotent stem cell-derived cardiomyocytes
It has been reported that pluripotent stem cells (iPSCs) can improve cardiac regeneration. They can re-myocardialize damaged myocardial regions and their derived cardiomyocytes show typical characteristics of cardiomyocytes, such as contractility, spontaneous beating, and ion channel expression (Rikhtegar et al. Citation2019; Cheng et al. Citation2023). Human pluripotent stem cell-derived cardiomyocytes (PSC-CMs) have been transplanted into various animal models including mice, rats, guinea pigs, swine, and nonhuman primates. Researchers have demonstrated that the transplanted CMs can survive and exhibit functional benefits in the injured heart (Shiba et al. Citation2016; Liu et al. Citation2018; Ishida et al. Citation2019; Wong et al. Citation2021). However, transient ventricular arrhythmias were detected after injection into ischemic hearts of non-human primates and pigs, and most preclinical studies showed that the benefits were detected in the acute (Gao et al. Citation2018) or subacute models of ischemic injury, but not in chronic ischemia models following direct cell injection (Fernandes et al. Citation2010; Shiba et al. Citation2014; Gao et al. Citation2018). Overall, the application of iPSCs derived from adult cells allow researchers worldwide to study laboratory-cultured cardiomyocytes and has overcome the ethical limitations associated with the use of embryonic stem cell-derived cardiomyocytes in research (Takahashi and Yamanaka Citation2006).
Non-cardiomyocyte reprograming
Direct reprograming of non-cardiomyocyte provides another suitable method for enhancing cardiomyocytes repair. Given that this method does not require in vitro culture and expansion, and immunosuppression, it is a promising therapeutic approach for cardiac regeneration (Perveen et al. Citation2023; Takahashi and Yamanaka Citation2006). Ieda et al. (Citation2010) demonstrated, through viral overexpression of three transcription factors, Gata4, Mef2c, and Tbx5 (GMT combinations), that neonatal murine cardiac and dermal fibroblasts can transdifferentiate into cardiomyocytes in vitro. During the transdifferentiation, the cells bypassed the pluripotent or mesodermal progenitor state and differentiated from fibroblasts to induced cardiomyocytes. Song et al. (Citation2012) found that four transcription factors, GHMT combinations, i.e. the addition of Hand2 to the GMT combinations, synergistically reprogramed mouse tail-tip fibroblasts and cardiac fibroblasts in vitro and transformed them into pulsating cardiac myocyte-like cells. In vivo application of GMT or similar GMHT, although less efficient, stimulated a more complete transdifferentiation of cells in terms of morphology and electrophysiological properties. In 2012, Qian et al. (Citation2012, Citation2013) reported that 4 weeks after cardiac injury, GMT treatment lead to the generation of new cardiomyocytes in approximately 35% of the junctional zone. the cardiomyocytes were produced by the transdifferentiation process. The experimental results of Mathison et al. (Citation2012) indicated that vascular endothelial growth factor increased the efficiency of GMT reprograming in murine hearts. In contrast, the study by Song's team found that approximately 6% of the junctional zone cardiomyocytes became neoplastic after 3 weeks of GMHT treatment and more than 10,000 new cardiomyocytes were generated (Song et al. Citation2012).
Conclusion
Cardiovascular diseases carry high morbidity and mortality risks, with failure of myocardial regeneration being the main cause of heart failure. In recent years, researchers have explored effective strategies to treat heart failure, including the development of novel drugs, mechanical device design, and cardiac transplantation. However, these strategies only delay cardiac remodeling but do not satisfactorily prevent or treat heart failure. In this regard, research on regenerative approaches for prevention of heart failure has intensified. In the last two decades, significant advances have been made in the field of cardiac regeneration with studies demonstrating that lower vertebrate zebrafish can regenerate. In addition, a neonatal mouse has been established as a prototypical mammal that can regenerate. There has been significant advances in the formulation of endogenous cardiac regeneration, exogenous cellular therapy based on stem cell differentiation and cell delivery systems. In this review, we summarized the developments and updates in research on zebrafish and mouse over the past 20 years, including the construction of multiple models, to provide a more comprehensive understanding of the mechanisms of cardiac regeneration, microRNA regulatory networks, and the signaling pathways. Our review is expected to provide renewed focus into investigations aimed at developing strategies to stimulate cardiomyocyte proliferation, stimulate cell cycle re-entry, identify barriers limiting the efficiency of cardiomyocyte proliferation, and uncover potential therapeutic targets for cardiac regeneration. This research aims to shed light on the intricate molecular and cellular mechanisms governing cardiac regeneration in adult mammals. Although true regeneration is a complex and highly regulated process, several challenges need to be overcome, such as arrhythmia during the healing process. A deeper understanding of the molecular pathways controlling cardiomyocyte differentiation, proliferation, and maturation is crucial to unlocking the robust regeneration pathways we seek for therapeutic exploration in humans. While drugs, mechanical devices, and cardiac transplantation will remain the mainstay of cardiac therapy in the short term, ultimately regenerative therapies based on the biological basis of regeneration may provide a more sustainable solution for patients with heart failure.
Author Contribution
Lijun Jin contributed to the conception and design of the manuscript. Lekun Gui carried out the literature search and drafted the paper. Huangjun Liu revised the paper for intellectual content. All authors agree to be accountable for all aspects of the work.
Disclosure statement
No potential conflict of interest was reported by the author(s).
Data availability statement
Data sharing not applicable to this article as no datasets were generated or analysed during the current study.
References
- Adler CP, Friedburg H, Herget GW, Neuburger M, Schwalb H. 1996. Variability of cardiomyocyte DNA content, ploidy level and nuclear number in mammalian hearts. Virchows Arch. 429:159–164. doi:10.1007/bf00192438.
- Al Haj Zen A, Oikawa A, Bazan-Peregrino M, Meloni M, Emanueli C, Madeddu P. 2010. Inhibition of delta-like-4-mediated signaling impairs reparative angiogenesis after ischemia. Circ Res. 107:283–293. doi:10.1161/CIRCRESAHA.110.221663.
- Ali SR, Hippenmeyer S, Saadat LV, Luo L, Weissman IL, Ardehali R. 2014. Existing cardiomyocytes generate cardiomyocytes at a low rate after birth in mice. Proc Natl Acad Sci U S A. 111:8850–8855. doi:10.1073/pnas.1408233111.
- Auman HJ, Coleman H, Riley HE, Olale F, Tsai H-J, Yelon D. 2007. Functional modulation of cardiac form through regionally confined cell shape changes. PLoS Biol. 5:e53. doi:10.1371/journal.pbio.0050053.
- Aurora AB, Mahmoud AI, Luo X, Johnson BA, van Rooij E, Matsuzaki S, Humphries KM, Hill JA, Bassel-Duby R, Sadek HA, Olson EN. 2012. MicroRNA-214 protects the mouse heart from ischemic injury by controlling Ca2+ overload and cell death. J Clin Invest. 122:1222–1232. doi:10.1172/JCI59327.
- Azizidoost S, Farzaneh M. 2023. MicroRNAs as a novel player for differentiation of mesenchymal stem cells into cardiomyocytes. Curr Stem Cell Res Ther. 18:27–34. doi:10.2174/1574888X17666220614160957.
- Becker RO, Chapin S, Sherry R. 1974. Regeneration of the ventricular myocardium in amphibians. Nature. 248:145–147. doi:10.1038/248145a0.
- Becker T, Wullimann MF, Becker CG, Bernhardt RR, Schachner M. 1997. Axonal regrowth after spinal cord transection in adult zebrafish. J Comp Neurol. 377:577–595. doi:10.1002/(SICI)1096-9861(19970127)377:4<577::AID-CNE8>3.0.CO;2-#.
- Bergmann O, Bhardwaj Ratan D, Bernard S, Zdunek S, Barnabé-Heider F, Walsh S, Zupicich J, Alkass K, Buchholz BA, Druid H, et al. 2009. Evidence for cardiomyocyte renewal in humans. Science. 324:98–102. doi:10.1126/science.1164680.
- Bergmann O, Zdunek S, Felker A, Salehpour M, Alkass K, Bernard S, Sjostrom S, Szewczykowska M, Jackowska T, dos Remedios C, et al. 2015. Dynamics of cell generation and turnover in the human heart. Cell. 161:1566–1575. doi:10.1016/j.cell.2015.05.026.
- Bertozzi A, Wu CC, Hans S, Brand M, Weidinger G. 2022. Wnt/β-catenin signaling acts cell-autonomously to promote cardiomyocyte regeneration in the zebrafish heart. Dev Biol. 481:226–237. doi:10.1016/j.ydbio.2021.11.001.
- Bevan L, Lim ZW, Venkatesh B, Riley PR, Martin P, Richardson RJ. 2020. Specific macrophage populations promote both cardiac scar deposition and subsequent resolution in adult zebrafish. Cardiovasc Res. 116:1357–1371. doi:10.1093/cvr/cvz221.
- Bolli R, Mitrani RD, Hare JM, Pepine CJ, Perin EC, Willerson JT, Traverse JH, Henry TD, Yang PC, Murphy MP, et al. 2021. A Phase II study of autologous mesenchymal stromal cells and c-kit positive cardiac cells, alone or in combination, in patients with ischaemic heart failure: the CCTRN CONCERT-HF trial. Eur J Heart Fail. 23:661–674. doi:10.1002/ejhf.2178.
- Borden A, Kurian J, Nickoloff E, Yang Y, Troupes CD, Ibetti J, Lucchese AM, Gao E, Mohsin S, Koch WJ, et al. 2019. Transient Introduction of miR-294 in the Heart Promotes Cardiomyocyte Cell Cycle Reentry After Injury. Circ Res. 125:14–25. doi:10.1161/CIRCRESAHA.118.314223.
- Brezitski KD, Goff AW, DeBenedittis P, Karra R. 2021. A roadmap to heart regeneration through conserved mechanisms in zebrafish and mammals. Curr Cardiol Rep. 23:29. doi:10.1007/s11886-021-01459-6.
- Brodsky V, Sarkisov DS, Arefyeva AM, Panova NW, Gvasava IG. 1994. Polyploidy in cardiac myocytes of normal and hypertrophic human hearts; range of values. Virchows Arch. 424:429–435. doi:10.1007/bf00190566.
- Brooks G, Poolman RA, Li JM. 1998. Arresting developments in the cardiac myocyte cell cycle: role of cyclin-dependent kinase inhibitors. Cardiovasc Res. 39:301–311. doi:10.1016/S0008-6363(98)00125-4.
- Cargnello M, Roux PP. 2011. Activation and function of the MAPKs and their substrates, the MAPK-activated protein kinases. Microbiol Mol Biol Rev. 75:50–83. doi:10.1128/MMBR.00031-10.
- Chen J, Huang Z-P, Seok HY, Ding J, Kataoka M, Zhang Z, Hu X, Wang G, Lin Z, Wang S, et al. 2013. mir-17-92 cluster is required for and sufficient to induce cardiomyocyte proliferation in postnatal and adult hearts. Circ Res. 112:1557–1566. doi:10.1161/CIRCRESAHA.112.300658.
- Chen SL, Fang W-w, Ye F, Liu Y-H, Qian J, Shan S-j, Zhang J-j, Chunhua RZ, Liao L-m, Lin S, Sun J-p. 2004. Effect on left ventricular function of intracoronary transplantation of autologous bone marrow mesenchymal stem cell in patients with acute myocardial infarction. Am J Cardiol. 94:92–95. doi:10.1016/j.amjcard.2004.03.034.
- Chen Z, Huang W, Dahme T, Rottbauer W, Ackerman MJ, Xu X. 2008. Depletion of zebrafish essential and regulatory myosin light chains reduces cardiac function through distinct mechanisms. Cardiovasc Res. 79:97–108. doi:10.1093/cvr/cvn073.
- Cheng YY, Gregorich Z, Prajnamitra RP, Lundy DJ, Ma T-Y, Huang Y-H, Lee Y-C, Ruan S-C, Lin J-H, Lin P-J, et al. 2022. Metabolic changes associated with cardiomyocyte dedifferentiation enable adult mammalian cardiac regeneration. Circulation. 146:1950–1967. doi:10.1161/CIRCULATIONAHA.122.061960.
- Cheng YY, Hu YF, Hsieh PC. 2023. The role of large animal models in cardiac regeneration research using human pluripotent stem cell-derived cardiomyocytes. Curr Cardiol Rep. 25:325–331. doi:10.1007/s11886-023-01857-y.
- Choi WY, Gemberling M, Wang J, Holdway JE, Shen M-C, Karlstrom RO, Poss KD. 2013. In vivo monitoring of cardiomyocyte proliferation to identify chemical modifiers of heart regeneration. Development. 140:660–666. doi:10.1242/dev.088526.
- Clerk A, Cullingford TE, Fuller SJ, Giraldo A, Markou T, Pikkarainen S, Sugden PH. 2007. Signaling pathways mediating cardiac myocyte gene expression in physiological and stress responses. J Cell Physiol. 212:311–322. doi:10.1002/jcp.21094.
- Collesi C, Zentilin L, Sinagra G, Giacca M. 2008. Notch1 signaling stimulates proliferation of immature cardiomyocytes. J Cell Biol. 183:117–128. doi:10.1083/jcb.200806091.
- Condorelli G, Latronico MV, Cavarretta E. 2014. microRNAs in cardiovascular diseases: current knowledge and the road ahead. J Am Coll Cardiol. 63:2177–2187. doi:10.1016/j.jacc.2014.01.050.
- Curado S, Anderson RM, Jungblut B, Mumm J, Schroeter E, Stainier DYR. 2007. Conditional targeted cell ablation in zebrafish: a new tool for regeneration studies. Dev Dyn. 236:1025–1035. doi:10.1002/dvdy.21100.
- D'Amato G, Luxán G, del Monte-Nieto G, Martínez-Poveda B, Torroja C, Walter W, Bochter MS, Benedito R, Cole S, Martinez F, et al. 2016. Sequential Notch activation regulates ventricular chamber development. Nat Cell Biol. 18:7–20. doi:10.1038/ncb3280.
- Das S, Halushka MK. 2015. Extracellular vesicle microRNA transfer in cardiovascular disease. Cardiovasc Pathol. 24:199–206. doi:10.1016/j.carpath.2015.04.007.
- Davis DR, Zhang Y, Smith RR, Cheng K, Terrovitis J, Malliaras K, Li T-S, White A, Makkar R, Marbán E. 2009. Validation of the cardiosphere method to culture cardiac progenitor cells from myocardial tissue. PLoS One. 4:e7195. doi:10.1371/journal.pone.0007195.
- del Monte G, Casanova JC, Guadix JA, MacGrogan D, Burch JBE, Pérez-Pomares JM, de la Pompa JL. 2011. Differential Notch signaling in the epicardium is required for cardiac inflow development and coronary vessel morphogenesis. Circ Res. 108:824–836. doi:10.1161/CIRCRESAHA.110.229062.
- Del Re DP, Yang Y, Nakano N, Cho J, Zhai P, Yamamoto T, Zhang N, Yabuta N, Nojima H, Pan D, Sadoshima J. 2013. Yes-associated protein isoform 1 (Yap1) promotes cardiomyocyte survival and growth to protect against myocardial ischemic injury. J Biol Chem. 288:3977–3988. doi:10.1074/jbc.M112.436311.
- de Pater E, Ciampricotti M, Priller F, Veerkamp J, Strate I, Smith K, Lagendijk AK, Schilling TF, Herzog W, Abdelilah-Seyfried S, et al. 2012. Bmp signaling exerts opposite effects on cardiac differentiation. Circ Res. 110:578–587. doi:10.1161/CIRCRESAHA.111.261172.
- Derks W, Bergmann O. 2020. Polyploidy in Cardiomyocytes: roadblock to Heart Regeneration? Circ Res. 126:552–565. doi:10.1161/CIRCRESAHA.119.315408.
- Du J, Zheng L, Gao P, Yang H, Yang W-J, Guo F, Liang R, Feng M, Wang Z, Zhang Z, et al. 2022. A small-molecule cocktail promotes mammalian cardiomyocyte proliferation and heart regeneration. Cell Stem Cell. 29:545–558.e513. doi:10.1016/j.stem.2022.03.009.
- Elia A, Mohsin S, Khan M. 2023. Cardiomyocyte ploidy, metabolic reprogramming and heart repair. Cells. 12. doi:10.3390/cells12121571.
- Farache Trajano L, Smart N. 2021. Immunomodulation for optimal cardiac regeneration: insights from comparative analyses. NPJ Regen Med. 6(8). doi:10.1038/s41536-021-00118-2.
- Feng J, et al. 2023. Versican promotes cardiomyocyte proliferation and cardiac repair. Circulation. doi:10.1161/circulationaha.123.066298.
- Feng L, et al. 2020. Simvastatin relieves myocardial ischemia/reperfusion injury in rats through hedgehog signaling pathway. Eur Rev Med Pharmacol Sci. 24:6400–6408. doi:10.26355/eurrev_202006_21538.
- Fernandes S, Naumova AV, Zhu WZ, Laflamme MA, Gold J, Murry CE. 2010. Human embryonic stem cell-derived cardiomyocytes engraft but do not alter cardiac remodeling after chronic infarction in rats. J Mol Cell Cardiol. 49:941–949. doi:10.1016/j.yjmcc.2010.09.008.
- Flinn MA, et al. 2020. Llgl1 regulates zebrafish cardiac development by mediating Yap stability in cardiomyocytes. Development. 147. doi:10.1242/dev.193581.
- Fratz S, Hager A, Schreiber C, Schwaiger M, Hess J, Stern HC. 2011. Long-term myocardial scarring after operation for anomalous left coronary artery from the pulmonary artery. Ann Thorac Surg. 92:1761–1765. doi:10.1016/j.athoracsur.2011.06.021.
- Gamba L, Harrison M, Lien CL. 2014. Cardiac regeneration in model organisms. Curr Treat Options Cardiovasc Med. 16:288. doi:10.1007/s11936-013-0288-8.
- Gao F, Kataoka M, Liu N, Liang T, Huang Z-P, Gu F, Ding J, Liu J, Zhang F, Ma Q, et al. 2019. Therapeutic role of miR-19a/19b in cardiac regeneration and protection from myocardial infarction. Nat Commun. 10:1802. doi:10.1038/s41467-019-09530-1.
- Gao L, Gregorich ZR, Zhu W, Mattapally S, Oduk Y, Lou X, Kannappan R, Borovjagin AV, Walcott GP, Pollard AE, et al. 2018. Large cardiac muscle patches engineered from human induced-pluripotent stem cell-derived cardiac cells improve recovery from myocardial infarction in swine. Circulation. 137:1712–1730. doi:10.1161/CIRCULATIONAHA.117.030785.
- Garbern JC, Lee RT. 2022. Heart regeneration: 20 years of progress and renewed optimism. Dev Cell. 57:424–439. doi:10.1016/j.devcel.2022.01.012.
- González-Rosa JM, Martín V, Peralta M, Torres M, Mercader N. 2011. Extensive scar formation and regression during heart regeneration after cryoinjury in zebrafish. Development. 138:1663–1674. doi:10.1242/dev.060897.
- González-Rosa JM, Sharpe M, Field D, Soonpaa MH, Field LJ, Burns CE, Burns CG. 2018. Myocardial polyploidization creates a barrier to heart regeneration in zebrafish. Dev Cell. 44:433–446.e437. doi:10.1016/j.devcel.2018.01.021.
- Guo Z, Geng M, Qin L, Hao B, Liao S. 2021. Epicardium-derived Tbx18(+) CDCs transplantation improve heart function in infarcted mice. Front Cardiovasc Med. 8:744353. doi:10.3389/fcvm.2021.744353.
- Gupta S, Sharma ASA, Verma RS. 2021. Mesenchymal stem cells for cardiac regeneration: from differentiation to cell delivery. Stem Cell Rev Rep. 17:1666–1694. doi:10.1007/s12015-021-10168-0.
- Gupta V, Gemberling M, Karra R, Rosenfeld G, Evans T, Poss K. 2013. An injury-responsive gata4 program shapes the zebrafish cardiac ventricle. Curr Biol. 23:1221–1227. doi:10.1016/j.cub.2013.05.028.
- Gupta V, Poss KD. 2012. Clonally dominant cardiomyocytes direct heart morphogenesis. Nature. 484:479–484. doi:10.1038/nature11045.
- Han L, Choudhury S, Mich-Basso JD, Ammanamanchi N, Ganapathy B, Suresh S, Khaladkar M, Singh J, Maehr R, Zuppo DA, et al. 2020. Lamin B2 levels regulate polyploidization of cardiomyocyte nuclei and myocardial regeneration. Dev Cell. 53:42–59.e11. doi:10.1016/j.devcel.2020.01.030.
- Haubner BJ, et al. 2012. Complete cardiac regeneration in a mouse model of myocardial infarction. Aging (Albany NY). 4:966–977. doi:10.18632/aging.100526.
- Haubner BJ, Schneider J, Schweigmann U, Schuetz T, Dichtl W, Velik-Salchner C, Stein J-I, Penninger JM. 2016. Functional recovery of a human neonatal heart after severe myocardial infarction. Circ Res. 118:216–221. doi:10.1161/CIRCRESAHA.115.307017.
- He J, Wang Y, Missinato MA, Onuoha E, Perkins LA, Watkins SC, St Croix CM, Tsang M, Bruchez MP. 2016. A genetically targetable near-infrared photosensitizer. Nat Methods. 13:263–268. doi:10.1038/nmeth.3735.
- Heallen T, Morikawa Y, Leach J, Tao G, Willerson JT, Johnson RL, Martin JF. 2013. Hippo signaling impedes adult heart regeneration. Development. 140:4683–4690. doi:10.1242/dev.102798.
- Ieda M, Fu J-D, Delgado-Olguin P, Vedantham V, Hayashi Y, Bruneau BG, Srivastava D. 2010. Direct reprogramming of fibroblasts into functional cardiomyocytes by defined factors. Cell. 142:375–386. doi:10.1016/j.cell.2010.07.002.
- Ikeda S, Mizushima W, Sciarretta S, Abdellatif M, Zhai P, Mukai R, Fefelova N, Oka S-i, Nakamura M, Del Re DP, et al. 2019. Hippo deficiency leads to cardiac dysfunction accompanied by cardiomyocyte dedifferentiation during pressure overload. Circ Res. 124:292–305. doi:10.1161/CIRCRESAHA.118.314048.
- Ishida M, Miyagawa S, Saito A, Fukushima S, Harada A, Ito E, Ohashi F, Watabe T, Hatazawa J, Matsuura K, Sawa Y. 2019. Transplantation of human-induced pluripotent stem cell-derived cardiomyocytes is superior to somatic stem cell therapy for restoring cardiac function and oxygen consumption in a porcine model of myocardial infarction. Transplantation. 103:291–298. doi:10.1097/TP.0000000000002384.
- Jabs M, Rose AJ, Lehmann LH, Taylor J, Moll I, Sijmonsma TP, Herberich SE, Sauer SW, Poschet G, Federico G, et al. 2018. Inhibition of endothelial Notch signaling impairs fatty acid transport and leads to metabolic and vascular remodeling of the adult heart. Circulation. 137:2592–2608. doi:10.1161/CIRCULATIONAHA.117.029733.
- Jia J, Jiang J. 2006. Decoding the Hedgehog signal in animal development. Cell Mol Life Sci. 63:1249–1265. doi:10.1007/s00018-005-5519-z.
- Johnson SL, Weston JA. 1995. Temperature-sensitive mutations that cause stage-specific defects in Zebrafish fin regeneration. Genetics. 141:1583–1595. doi:10.1093/genetics/141.4.1583.
- Jopling C, Sleep E, Raya M, Martí M, Raya A, Belmonte JCI. 2010. Zebrafish heart regeneration occurs by cardiomyocyte dedifferentiation and proliferation. Nature. 464:606–609. doi:10.1038/nature08899.
- Jopling C, Suñè G, Morera C, Izpisua Belmote JC. 2012. p38α MAPK regulates myocardial regeneration in zebrafish. Cell Cycle. 11:1195–1201. doi:10.4161/cc.11.6.19637.
- Kachanova O, Lobov A, Malashicheva A. 2022. The role of the Notch signaling pathway in recovery of cardiac function after myocardial infarction. Int J Mol Sci. 23. doi:10.3390/ijms232012509.
- Karra R, Knecht AK, Kikuchi K, Poss KD. 2015. Myocardial NF-κB activation is essential for zebrafish heart regeneration. Proc Natl Acad Sci U S A. 112:13255–13260. doi:10.1073/pnas.1511209112.
- Kasai-Brunswick TH, Costa ARd, Barbosa RAQ, Farjun B, Mesquita FCP, Silva dos Santos D, Ramos IP, Suhett G, Brasil GV, Cunha STd, et al. 2017. Cardiosphere-derived cells do not improve cardiac function in rats with cardiac failure. Stem Cell Res Ther. 8:36. doi:10.1186/s13287-017-0481-x.
- Kebbe M, Naud P, Assous I, Gagnon E, McCall A, Villeneuve L, Leblanc CA, Nguyen QT, Calderone A. 2023. Distinct protein kinase C isoforms drive the cell cycle re-entry of two separate populations of neonatal rat ventricular cardiomyocytes. Am J Physiol Cell Physiol. 325:C406–c419. doi:10.1152/ajpcell.00506.2022.
- Kikuchi K, Holdway JE, Werdich AA, Anderson RM, Fang Y, Egnaczyk GF, Evans T, MacRae CA, Stainier DYR, Poss KD. 2010. Primary contribution to zebrafish heart regeneration by gata4(+) cardiomyocytes. Nature. 464:601–605. doi:10.1038/nature08804.
- Kroehne V, Freudenreich D, Hans S, Kaslin J, Brand M. 2011. Regeneration of the adult zebrafish brain from neurogenic radial glia-type progenitors. Development. 138:4831–4841. doi:10.1242/dev.072587.
- Kusano KF, Pola R, Murayama T, Curry C, Kawamoto A, Iwakura A, Shintani S, Ii M, Asai J, Tkebuchava T, et al. 2005. Sonic hedgehog myocardial gene therapy: tissue repair through transient reconstitution of embryonic signaling. Nat Med. 11:1197–1204. doi:10.1038/nm1313.
- Lai SL, et al. 2017. Reciprocal analyses in zebrafish and medaka reveal that harnessing the immune response promotes cardiac regeneration. Elife. 6. doi:10.7554/eLife.25605.
- Lai SL, Marín-Juez R, Stainier DYR. 2019. Immune responses in cardiac repair and regeneration: a comparative point of view. Cell Mol Life Sci. 76:1365–1380. doi:10.1007/s00018-018-2995-5.
- Lam NT, Sadek HA. 2018. Neonatal heart regeneration. Comprehensive Literature Review. Circulation. 138:412–423. doi:10.1161/circulationaha.118.033648.
- Lavine KJ, Kovacs A, Ornitz DM. 2008. Hedgehog signaling is critical for maintenance of the adult coronary vasculature in mice. J Clin Invest. 118:2404–2414. doi:10.1172/jci34561.
- Lepilina A, Coon AN, Kikuchi K, Holdway JE, Roberts RW, Burns C Poss KD. 2006. A dynamic epicardial injury response supports progenitor cell activity during zebrafish heart regeneration. Cell. 127:607–619. doi:10.1016/j.cell.2006.08.052.
- Li R, Xiang C, Li Y, Nie Y. 2023. Targeting immunoregulation for cardiac regeneration. J Mol Cell Cardiol. 177:1–8. doi:10.1016/j.yjmcc.2023.02.003.
- Lien CL, Harrison MR, Tuan TL, Starnes VA. 2012. Heart repair and regeneration: recent insights from zebrafish studies. Wound Repair Regen. 20:638–646. doi:10.1111/j.1524-475X.2012.00814.x.
- Lin Z, Zhou P, von Gise A, Gu F, Ma Q, Chen J, Guo H, van Gorp PRR, Wang D-Z, Pu WT. 2015. Pi3kcb links Hippo-YAP and PI3K-AKT signaling pathways to promote cardiomyocyte proliferation and survival. Circ Res. 116:35–45. doi:10.1161/CIRCRESAHA.115.304457.
- Liu J, Li W, Deng K-Q, Tian S, Liu H, Shi H, Fang Q, Liu Z, Chen Z, Tian T, et al. 2022. The E3 ligase TRIM16 is a key suppressor of pathological cardiac hypertrophy. Circ Res. 130:1586–1600. doi:10.1161/CIRCRESAHA.121.318866.
- Liu K, Jin H, Zhou B. 2020. Genetic lineage tracing with multiple DNA recombinases: a user's guide for conducting more precise cell fate mapping studies. J Biol Chem. 295:6413–6424. doi:10.1074/jbc.REV120.011631.
- Liu N, Olson EN. 2010. MicroRNA regulatory networks in cardiovascular development. Dev Cell. 18:510–525. doi:10.1016/j.devcel.2010.03.010.
- Liu P, Zhong TP. 2017. MAPK/ERK signalling is required for zebrafish cardiac regeneration. Biotechnol Lett. 39:1069–1077. doi:10.1007/s10529-017-2329-y.
- Liu X, Xiao J, Zhu H, Wei X, Platt C, Damilano F, Xiao C, Bezzerides V, Boström P, Che L, et al. 2015. miR-222 is necessary for exercise-induced cardiac growth and protects against pathological cardiac remodeling. Cell Metab. 21:584–595. doi:10.1016/j.cmet.2015.02.014.
- Liu YW, Chen B, Yang X, Fugate JA, Kalucki FA, Futakuchi-Tsuchida A, Couture L, Vogel KW, Astley CA, Baldessari A, et al. 2018. Human embryonic stem cell-derived cardiomyocytes restore function in infarcted hearts of non-human primates. Nat Biotechnol. 36:597–605. doi:10.1038/nbt.4162.
- López E, Marinaro F, de Pedro MdlÁ, Sánchez-Margallo FM, Gómez-Serrano M, Ponath V, Pogge von Strandmann E, Jorge I, Vázquez J, Fernández-Pereira LM, et al. 2020. The immunomodulatory signature of extracellular vesicles from cardiosphere-derived cells: a proteomic and miRNA profiling. Front Cell Dev Biol. 8:321. doi:10.3389/fcell.2020.00321.
- Macmahon HE. 1937. Hyperplasia and regeneration of the myocardium in infants and in children. Am J Pathol. 13:845–854.
- Makkar RR, Kereiakes DJ, Aguirre F, Kowalchuk G, Chakravarty T, Malliaras K, Francis GS, Povsic TJ, Schatz R, Traverse JH, et al. 2020. Intracoronary ALLogeneic heart STem cells to Achieve myocardial Regeneration (ALLSTAR): a randomized, placebo-controlled, double-blinded trial. Eur Heart J. 41:3451–3458. doi:10.1093/eurheartj/ehaa541.
- Malliaras K, Li T-S, Luthringer D, Terrovitis J, Cheng K, Chakravarty T, Galang G, Zhang Y, Schoenhoff F, Van Eyk J, et al. 2012. Safety and efficacy of allogeneic cell therapy in infarcted rats transplanted with mismatched cardiosphere-derived cells. Circulation. 125:100–112. doi:10.1161/CIRCULATIONAHA.111.042598.
- Malliaras K, Makkar RR, Smith RR, Cheng K, Wu E, Bonow RO, Marbán L, Mendizabal A, Cingolani E, Johnston PV, et al. 2014. Intracoronary cardiosphere-derived cells after myocardial infarction: evidence of therapeutic regeneration in the final 1-year results of the CADUCEUS trial (CArdiosphere-Derived aUtologous stem CElls to reverse ventricUlar dySfunction). J Am Coll Cardiol. 63:110–122. doi:10.1016/j.jacc.2013.08.724.
- Marín-Juez R, Marass M, Gauvrit S, Rossi A, Lai S-L, Materna SC, Black BL, Stainier DYR. 2016. Fast revascularization of the injured area is essential to support zebrafish heart regeneration. Proc Natl Acad Sci U S A. 113:11237–11242. doi:10.1073/pnas.1605431113.
- Mathison M, et al. 2012. In vivo cardiac cellular reprogramming efficacy is enhanced by angiogenic preconditioning of the infarcted myocardium with vascular endothelial growth factor. J Am Heart Assoc. 1:e005652. doi:10.1161/jaha.112.005652.
- Menasché P, Alfieri O, Janssens S, McKenna W, Reichenspurner H, Trinquart L, Vilquin J-T, Marolleau J-P, Seymour B, Larghero J, et al. 2008. The Myoblast Autologous Grafting in Ischemic Cardiomyopathy (MAGIC) trial: first randomized placebo-controlled study of myoblast transplantation. Circulation. 117:1189–1200. doi:10.1161/CIRCULATIONAHA.107.734103.
- Menasché P, Hagège AA, Scorsin M, Pouzet B, Desnos M, Duboc D, Schwartz K, Vilquin J-T, Marolleau J-P. 2001. Myoblast transplantation for heart failure. Lancet. 357:279–280. doi:10.1016/S0140-6736(00)03617-5.
- Mishra PK, Tyagi N, Kumar M, Tyagi SC. 2009. MicroRNAs as a therapeutic target for cardiovascular diseases. J Cell Mol Med. 13:778–789. doi:10.1111/j.1582-4934.2009.00744.x.
- Missinato MA, et al. 2018. Dusp6 attenuates Ras/MAPK signaling to limit zebrafish heart regeneration. Development. 145. doi:10.1242/dev.157206.
- Mollova M, Bersell K, Walsh S, Savla J, Das LT, Park S-Y, Silberstein LE, dos Remedios CG, Graham D, Colan S, Kühn B. 2013. Cardiomyocyte proliferation contributes to heart growth in young humans. Proc Natl Acad Sci U S A. 110:1446–1451. doi:10.1073/pnas.1214608110.
- Monroe TO, Hill MC, Morikawa Y, Leach JP, Heallen T, Cao S, Krijger PHL, de Laat W, Wehrens XHT, Rodney GG, Martin JF. 2019. YAP partially reprograms chromatin accessibility to directly induce adult cardiogenesis in vivo. Dev Cell. 48:765–779.e767. doi:10.1016/j.devcel.2019.01.017.
- Natarajan N, Abbas Y, Bryant DM, Gonzalez-Rosa JM, Sharpe M, Uygur A, Cocco-Delgado LH, Ho NN, Gerard NP, Gerard CJ, et al. 2018. Complement receptor C5aR1 plays an evolutionarily conserved role in successful cardiac regeneration. Circulation. 137:2152–2165. doi:10.1161/CIRCULATIONAHA.117.030801.
- Nemir M, Metrich M, Plaisance I, Lepore M, Cruchet S, Berthonneche C, Sarre A, Radtke F, Pedrazzini T. 2014. The Notch pathway controls fibrotic and regenerative repair in the adult heart. Eur Heart J. 35:2174–2185. doi:10.1093/eurheartj/ehs269.
- Nguyen PD, Gooijers I, Campostrini G, Verkerk AO, Honkoop H, Bouwman M, de Bakker DEM, Koopmans T, Vink A, Lamers GEM, et al. 2023. Interplay between calcium and sarcomeres directs cardiomyocyte maturation during regeneration. Science. 380:758–764. doi:10.1126/science.abo6718.
- Noseda M, Harada M, McSweeney S, Leja T, Belian E, Stuckey DJ, Abreu Paiva MS, Habib J, Macaulay I, de Smith AJ, et al. 2015. PDGFRα demarcates the cardiogenic clonogenic Sca1 + stem/progenitor cell in adult murine myocardium. Nat Commun. 6:6930. doi:10.1038/ncomms7930.
- Oberpriller J, Oberpriller JC. 1971. Mitosis in adult newt ventricle. J Cell Biol. 49:560–563. doi:10.1083/jcb.49.2.560.
- Paige SL, Plonowska K, Xu A, Wu SM. 2015. Molecular regulation of cardiomyocyte differentiation. Circ Res. 116:341–353. doi:10.1161/CIRCRESAHA.116.302752.
- Parente V, Balasso S, Pompilio G, Verduci L, Colombo GI, Milano G, Guerrini U, Squadroni L, Cotelli F, Pozzoli O, Capogrossi MC. 2013. Hypoxia/reoxygenation cardiac injury and regeneration in zebrafish adult heart. PLoS One. 8:e53748. doi:10.1371/journal.pone.0053748.
- Patterson M, Barske L, Van Handel B, Rau CD, Gan P, Sharma A, Parikh S, Denholtz M, Huang Y, Yamaguchi Y, et al. 2017. Frequency of mononuclear diploid cardiomyocytes underlies natural variation in heart regeneration. Nat Genet. 49:1346–1353. doi:10.1038/ng.3929.
- Peiseler M, Kubes P. 2019. More friend than foe: the emerging role of neutrophils in tissue repair. J Clin Invest. 129:2629–2639. doi:10.1172/JCI124616.
- Perveen S, Vanni R, Lo Iacono M, Rastaldo R, Giachino C. 2023. Direct reprogramming of resident non-myocyte cells and its potential for in vivo cardiac regeneration. Cells. 12. doi:10.3390/cells12081166.
- Pezhouman A, Nguyen NB, Kay M, Kanjilal B, Noshadi I, Ardehali R. 2023. Cardiac regeneration - past advancements, current challenges, and future directions. J Mol Cell Cardiol. 182:75–85. doi:10.1016/j.yjmcc.2023.07.009.
- Porrello ER, Mahmoud AI, Simpson E, Hill JA, Richardson JA, Olson EN, Sadek HA. 2011. Transient regenerative potential of the neonatal mouse heart. Science. 331:1078–1080. doi:10.1126/science.1200708.
- Porrello ER, Mahmoud AI, Simpson E, Johnson BA, Grinsfelder D, Canseco D, Mammen PP, Rothermel BA, Olson EN, Sadek HA. 2013. Regulation of neonatal and adult mammalian heart regeneration by the miR-15 family. Proc Natl Acad Sci U S A. 110:187–192. doi:10.1073/pnas.1208863110.
- Porrello ER, Olson EN. 2014. A neonatal blueprint for cardiac regeneration. Stem Cell Res. 13:556–570. doi:10.1016/j.scr.2014.06.003.
- Poss KD, Wilson LG, Keating MT. 2002. Heart regeneration in zebrafish. Science. 298:2188–2190. doi:10.1126/science.1077857.
- Puente BN, Kimura W, Muralidhar S, Moon J, Amatruda J, Phelps K, Grinsfelder D, Rothermel B, Chen R, Garcia J, et al. 2014. The oxygen-rich postnatal environment induces cardiomyocyte cell-cycle arrest through DNA damage response. Cell. 157:565–579. doi:10.1016/j.cell.2014.03.032.
- Qian L, Berry EC, Fu JD, Ieda M, Srivastava D. 2013. Reprogramming of mouse fibroblasts into cardiomyocyte-like cells in vitro. Nat Protoc. 8:1204–1215. doi:10.1038/nprot.2013.067.
- Qian L, Huang Y, Spencer CI, Foley A, Vedantham V, Liu L, Conway SJ, Fu J-d, Srivastava D. 2012. In vivo reprogramming of murine cardiac fibroblasts into induced cardiomyocytes. Nature. 485:593–598. doi:10.1038/nature11044.
- Quaife-Ryan GA, Sim CB, Ziemann M, Kaspi A, Rafehi H, Ramialison M, El-Osta A, Hudson JE, Porrello ER. 2017. Multicellular transcriptional analysis of mammalian heart regeneration. Circulation. 136:1123–1139. doi:10.1161/CIRCULATIONAHA.117.028252.
- Raso A, Dirkx E, Sampaio-Pinto V, el Azzouzi H, Cubero RJ, Sorensen DW, Ottaviani L, Olieslagers S, Huibers MM, de Weger R, et al. 2021. A microRNA program regulates the balance between cardiomyocyte hyperplasia and hypertrophy and stimulates cardiac regeneration. Nat Commun. 12:4808. doi:10.1038/s41467-021-25211-4.
- Raya A, et al. 2003. Activation of Notch signaling pathway precedes heart regeneration in zebrafish. Proc Natl Acad Sci U S A. 100(Suppl 1):11889–11895. doi:10.1073/pnas.1834204100.
- Regula KM, Rzeszutek MJ, Baetz D, Seneviratne C, Kirshenbaum LA. 2004. Therapeutic opportunities for cell cycle re-entry and cardiac regeneration. Cardiovasc Res. 64:395–401. doi:10.1016/j.cardiores.2004.09.003.
- Rikhtegar R, Pezeshkian M, Dolati S, Safaie N, Afrasiabi Rad A, Mahdipour M, Nouri M, Jodati AR, Yousefi M. 2019. Stem cells as therapy for heart disease: iPSCs, ESCs, CSCs, and skeletal myoblasts. Biomed Pharmacother. 109:304–313. doi:10.1016/j.biopha.2018.10.065.
- Riley SE, Feng Y, Hansen CG. 2022. Hippo-Yap/Taz signalling in zebrafish regeneration. NPJ Regen Med. 7:9. doi:10.1038/s41536-022-00209-8.
- Robbins DJ, Fei DL, Riobo NA. 2012. The Hedgehog signal transduction network. Sci Signal. 5:re6. doi:10.1126/scisignal.2002906.
- Ross Stewart KM, Walker SL, Baker AH, Riley PR, Brittan M. 2022. Hooked on heart regeneration: the zebrafish guide to recovery. Cardiovasc Res. 118:1667–1679. doi:10.1093/cvr/cvab214.
- Samsa LA, Givens C, Tzima E, Stainier DYR, Qian L, Liu J. 2015. Cardiac contraction activates endocardial Notch signaling to modulate chamber maturation in zebrafish. Development. 142:4080–4091. doi:10.1242/dev.125724.
- Sánchez-Iranzo H, Galardi-Castilla M, Sanz-Morejón A, González-Rosa JM, Costa R, Ernst A, Sainz de Aja J, Langa X, Mercader N. 2018. Transient fibrosis resolves via fibroblast inactivation in the regenerating zebrafish heart. Proc Natl Acad Sci U S A. 115:4188–4193. doi:10.1073/pnas.1716713115.
- Sande-Melón M, Marques IJ, Galardi-Castilla M, Langa X, Pérez-López M, Botos M-A, Sánchez-Iranzo H, Guzmán-Martínez G, Ferreira Francisco DM, Pavlinic D, et al. 2019. Adult sox10(+) Cardiomyocytes Contribute to Myocardial Regeneration in the Zebrafish. Cell Rep. 29:1041–1054.e1045. doi:10.1016/j.celrep.2019.09.041.
- Sayers JR, Riley PR. 2021. Heart regeneration: beyond new muscle and vessels. Cardiovasc Res. 117:727–742. doi:10.1093/cvr/cvaa320.
- Schnabel K, Wu CC, Kurth T, Weidinger G. 2011. Regeneration of cryoinjury induced necrotic heart lesions in zebrafish is associated with epicardial activation and cardiomyocyte proliferation. PLoS One. 6:e18503. doi:10.1371/journal.pone.0018503.
- Senyo SE, Steinhauser ML, Pizzimenti CL, Yang VK, Cai L, Wang M, Wu T-D, Guerquin-Kern J-L, Lechene CP, Lee RT. 2013. Mammalian heart renewal by pre-existing cardiomyocytes. Nature. 493:433–436. doi:10.1038/nature11682.
- Shao J, Lin W, Lin B, Wang Q, Chen Y, Fan C. 2020. MiR-377 accelerates cardiac hypertrophy by inhibiting autophagy via targeting PPARγ. All Life. 13:456–465. doi:10.1080/26895293.2020.1808083.
- Shiba Y, Filice D, Fernandes S, Minami E, Dupras SK, Biber BV, Trinh P, Hirota Y, Gold JD, Viswanathan M, Laflamme MA. 2014. Electrical integration of human embryonic stem cell-derived cardiomyocytes in a guinea pig chronic infarct model. J Cardiovasc Pharmacol Ther. 19:368–381. doi:10.1177/1074248413520344.
- Shiba Y, Gomibuchi T, Seto T, Wada Y, Ichimura H, Tanaka Y, Ogasawara T, Okada K, Shiba N, Sakamoto K, et al. 2016. Allogeneic transplantation of iPS cell-derived cardiomyocytes regenerates primate hearts. Nature. 538:388–391. doi:10.1038/nature19815.
- Singh BN, Koyano-Nakagawa N, Gong W, Moskowitz IP, Weaver CV, Braunlin E, Das S, van Berlo JH, Garry MG, Garry DJ. 2018. A conserved HH-Gli1-Mycn network regulates heart regeneration from newt to human. Nat Commun. 9:4237. doi:10.1038/s41467-018-06617-z.
- Sogo T, Nakao S, Tsukamoto T, Ueyama T, Harada Y, Ihara D, Ishida T, Nakahara M, Hasegawa K, Akagi Y, et al. 2023. Canonical Wnt signaling activation by chimeric antigen receptors for efficient cardiac differentiation from mouse embryonic stem cells. Inflamm Regen. 43:11. doi:10.1186/s41232-023-00258-6.
- Sommerfeld SD, et al. 2019. Interleukin-36γ-producing macrophages drive IL-17-mediated fibrosis. Sci Immunol. 4. doi:10.1126/sciimmunol.aax4783.
- Song K, Nam Y-J, Luo X, Qi X, Tan W, Huang GN, Acharya A, Smith CL, Tallquist MD, Neilson EG, et al. 2012. Heart repair by reprogramming non-myocytes with cardiac transcription factors. Nature. 485:599–604. doi:10.1038/nature11139.
- Soonpaa MH, Kim KK, Pajak L, Franklin M, Field LJ. 1996. Cardiomyocyte DNA synthesis and binucleation during murine development. Am J Physiol. 271:H2183–H2189. doi:10.1152/ajpheart.1996.271.5.H2183.
- Soonpaa MH, Lafontant PJ, Reuter S, Scherschel JA, Srour EF, Zaruba M-M, Rubart-von der Lohe M, Field LJ. 2018. Absence of cardiomyocyte differentiation following transplantation of adult cardiac-resident Sca-1(+) cells into infarcted mouse hearts. Circulation. 138:2963–2966. doi:10.1161/CIRCULATIONAHA.118.035391.
- Sorbini M, Arab S, Soni T, Frisiras A, Mehta S. 2023. How can the adult zebrafish and neonatal mice teach us about stimulating cardiac regeneration in the human heart? Regen Med. 18:85–99. doi:10.2217/rme-2022-0161.
- Takahashi K, Yamanaka S. 2006. Induction of pluripotent stem cells from mouse embryonic and adult fibroblast cultures by defined factors. Cell. 126:663–676. doi:10.1016/j.cell.2006.07.024.
- Tang J, et al. 2021. Sca1 marks a reserve endothelial progenitor population that preferentially expand after injury. Cell Discov. 7:88. doi:10.1038/s41421-021-00303-z.
- Tang W, Martik ML, Li Y, Bronner ME. 2019. Cardiac neural crest contributes to cardiomyocytes in amniotes and heart regeneration in zebrafish. Elife. 8. doi:10.7554/eLife.47929.
- Traxler D, et al. 2023. Plasma small extracellular vesicle cardiac miRNA expression in patients with ischemic heart failure, randomized to percutaneous intramyocardial treatment of adipose derived stem cells or placebo: subanalysis of the science study. Int J Mol Sci. 24. doi:10.3390/ijms241310647.
- Tsang V, Yacoub M, Sridharan S, Burch M, Radley-Smith R, Khaghani A, Savoldo B, Amrolia PJ. 2009. Late donor cardiectomy after paediatric heterotopic cardiac transplantation. Lancet. 374:387–392. doi:10.1016/S0140-6736(09)61201-0.
- Tse HF, Kwong Y-L, Chan JK, Lo G, Ho C-L, Lau C-P. 2003. Angiogenesis in ischaemic myocardium by intramyocardial autologous bone marrow mononuclear cell implantation. Lancet. 361:47–49. doi:10.1016/S0140-6736(03)12111-3.
- Uchida S, De Gaspari P, Kostin S, Jenniches K, Kilic A, Izumiya Y, Shiojima I, grosse Kreymborg K, Renz H, Walsh K, Braun T. 2013. Sca1-derived cells are a source of myocardial renewal in the murine adult heart. Stem Cell Rep. 1:397–410. doi:10.1016/j.stemcr.2013.09.004.
- Urbanek K, Cabral-da-Silva MC, Ide-Iwata N, Maestroni S, Delucchi F, Zheng H, Ferreira-Martins J, Ogórek B, D'Amario D, Bauer M, et al. 2010. Inhibition of NOTCH1-dependent cardiomyogenesis leads to a dilated myopathy in the neonatal heart. Circ Res. 107:429–441. doi:10.1161/CIRCRESAHA.110.218487.
- Vagnozzi RJ, Sargent MA, Lin S-CJ, Palpant NJ, Murry CE, Molkentin JD. 2018. Genetic lineage tracing of Sca-1(+) cells reveals endothelial but not myogenic contribution to the murine heart. Circulation. 138:2931–2939. doi:10.1161/CIRCULATIONAHA.118.035210.
- Vihtelic TS, Hyde DR. 2000. Light-induced rod and cone cell death and regeneration in the adult albino zebrafish (Danio rerio) retina. J Neurobiol. 44:289–307. doi:10.1002/1097-4695(20000905)44:3<289::AID-NEU1>3.0.CO;2-H.
- Vujic A, Natarajan N, Lee RT. 2020. Molecular mechanisms of heart regeneration. Semin Cell Dev Biol. 100:20–28. doi:10.1016/j.semcdb.2019.09.003.
- Walsh S, Pontén A, Fleischmann BK, Jovinge S. 2010. Cardiomyocyte cell cycle control and growth estimation in vivo–an analysis based on cardiomyocyte nuclei. Cardiovasc Res. 86:365–373. doi:10.1093/cvr/cvq005.
- Wang BB, Xu M, Li M, Wu F, Hu S, Chen X, Zhao L, Huang Z, Lan F, Liu D, Wang Y. 2020. miR-25 Promotes cardiomyocyte proliferation by targeting FBXW7. Mol Ther Nucleic Acids. 19:1299–1308. doi:10.1016/j.omtn.2020.01.013.
- Wang CC, et al. 2018. MicroRNA-325 alleviates myocardial fibrosis after myocardial infarction via downregulating GLI1. Eur Rev Med Pharmacol Sci. 22:5339–5346. doi:10.26355/eurrev_201808_15735.
- Wang J, Panáková D, Kikuchi K, Holdway JE, Gemberling M, Burris JS, Singh SP, Dickson AL, Lin Y-F, Sabeh MK, et al. 2011. The regenerative capacity of zebrafish reverses cardiac failure caused by genetic cardiomyocyte depletion. Development. 138:3421–3430. doi:10.1242/dev.068601.
- Wang M, Tsai BM, Turrentine MW, Mahomed Y, Brown JW, Meldrum DR. 2005. p38 mitogen activated protein kinase mediates both death signaling and functional depression in the heart. Ann Thorac Surg. 80:2235–2241. doi:10.1016/j.athoracsur.2005.05.070.
- Wang T, Chen X, Wang K, Ju J, Yu X, Wang S, Liu C, Wang K. 2023. Cre-loxP-mediated genetic lineage tracing: unraveling cell fate and origin in the developing heart. Front Cardiovasc Med. 10:1085629. doi:10.3389/fcvm.2023.1085629.
- Wang WE, Li L, Xia X, Fu W, Liao Q, Lan C, Yang D, Chen H, Yue R, Zeng C, et al. 2017. Dedifferentiation, proliferation, and redifferentiation of adult mammalian cardiomyocytes after ischemic injury. Circulation. 136:834–848. doi:10.1161/CIRCULATIONAHA.116.024307.
- Wei BR, et al. 2011. Capacity for resolution of Ras-MAPK-initiated early pathogenic myocardial hypertrophy modeled in mice. Comp Med. 61:109–118.
- Weinberger M, Riley PR. 2023. Animal models to study cardiac regeneration. Nat Rev Cardiol. doi:10.1038/s41569-023-00914-x.
- Widmann C, Gibson S, Jarpe MB, Johnson GL. 1999. Mitogen-activated protein kinase: conservation of a three-kinase module from yeast to human. Physiol Rev. 79:143–180. doi:10.1152/physrev.1999.79.1.143.
- Witman N, Murtuza B, Davis B, Arner A, Morrison JI. 2011. Recapitulation of developmental cardiogenesis governs the morphological and functional regeneration of adult newt hearts following injury. Dev Biol. 354:67–76. doi:10.1016/j.ydbio.2011.03.021.
- Wong KS, Rehn K, Palencia-Desai S, Kohli V, Hunter W, Uhl JD, Rost MS, Sumanas S. 2012. Hedgehog signaling is required for differentiation of endocardial progenitors in zebrafish. Dev Biol. 361:377–391. doi:10.1016/j.ydbio.2011.11.004.
- Wong LY, Glatz JFC, Wang S, Geraets IME, Vanherle S, Wijngaard Avd, Brunner H, Luiken JJFP, Nabben M. 2021. Comparison of human and rodent cell models to study myocardial lipid-induced insulin resistance. Prostaglandins Leukot Essent Fatty Acids. 167:102267. doi:10.1016/j.plefa.2021.102267.
- Wu CC, Kruse F, Vasudevarao MD, Junker JP, Zebrowski DC, Fischer K, Noël ES, Grün D, Berezikov E, Engel FB, et al. 2016. Spatially resolved genome-wide transcriptional profiling identifies bmp signaling as essential regulator of zebrafish cardiomyocyte regeneration. Dev Cell. 36:36–49. doi:10.1016/j.devcel.2015.12.010.
- Wu Y, Zhou L, Liu H, Duan R, Zhou H, Zhang F, He X, Lu D, Xiong K, Xiong M, et al. 2021. LRP6 downregulation promotes cardiomyocyte proliferation and heart regeneration. Cell Res. 31:450–462. doi:10.1038/s41422-020-00411-7.
- Xiao S, Liang R, Muili AB, Cao X, Navran S, Schwartz RJ, Iyer D. 2022. Mutant SRF and YAP synthetic modified mRNAs drive cardiomyocyte nuclear replication. J Cardiovasc Aging. 2:29. doi:10.20517/jca.2022.17.
- Xie S, Fu W, Yu G, Hu X, Lai KS, Peng X, Zhou Y, Zhu X, Christov P, Sawyer L, et al. 2020. Discovering small molecules as Wnt inhibitors that promote heart regeneration and injury repair. J Mol Cell Biol. 12:42–54. doi:10.1093/jmcb/mjz023.
- Xin M, et al. 2011. Regulation of insulin-like growth factor signaling by Yap governs cardiomyocyte proliferation and embryonic heart size. Sci Signal. 4:ra70. doi:10.1126/scisignal.2002278.
- Xin M, Kim Y, Sutherland LB, Murakami M, Qi X, McAnally J, Porrello ER, Mahmoud AI, Tan W, Shelton JM, et al. 2013. Hippo pathway effector Yap promotes cardiac regeneration. Proc Natl Acad Sci U S A. 110:13839–13844. doi:10.1073/pnas.1313192110.
- Yang P, Chen Z, Huang W, Zhang J, Zou L, Wang H. 2023. Communications between macrophages and cardiomyocytes. Cell Commun Signal. 21:206. doi:10.1186/s12964-023-01202-4.
- Ye B, Zhou H, Chen Y, Luo W, Lin W, Zhao Y, Han J, Han X, Huang W, Wu G, et al. 2023. USP25 ameliorates pathological cardiac hypertrophy by stabilizing SERCA2a in cardiomyocytes. Circ Res. 132:465–480. doi:10.1161/CIRCRESAHA.122.321849.
- Zhang L, Sultana N, Yan J, Yang F, Chen F, Chepurko E, Yang F-C, Du Q, Zangi L, Xu M, et al. 2018. Cardiac Sca-1(+) cells are not intrinsic stem cells for myocardial development, renewal, and repair. Circulation. 138:2919–2930. doi:10.1161/CIRCULATIONAHA.118.035200.
- Zhang R, Han P, Yang H, Ouyang K, Lee D, Lin Y-F, Ocorr K, Kang G, Chen J, Stainier DYR, et al. 2013. In vivo cardiac reprogramming contributes to zebrafish heart regeneration. Nature. 498:497–501. doi:10.1038/nature12322.
- Zhao B, Tumaneng K, Guan KL. 2011. The Hippo pathway in organ size control, tissue regeneration and stem cell self-renewal. Nat Cell Biol. 13:877–883. doi:10.1038/ncb2303.
- Zhao L, Ben-Yair R, Burns CE, Burns CG. 2019. Endocardial Notch signaling promotes cardiomyocyte proliferation in the regenerating zebrafish heart through Wnt pathway antagonism. Cell Rep. 26:546–554.e545. doi:10.1016/j.celrep.2018.12.048.
- Zhao L, Borikova AL, Ben-Yair R, Guner-Ataman B, MacRae CA, Lee RT, Burns CG, Burns CE. 2014. Notch signaling regulates cardiomyocyte proliferation during zebrafish heart regeneration. Proc Natl Acad Sci U S A. 111:1403–1408. doi:10.1073/pnas.1311705111.
- Zhao T, Zhao W, Chen Y, Ahokas RA, Sun Y. 2010. Vascular endothelial growth factor (VEGF)-A: role on cardiac angiogenesis following myocardial infarction. Microvasc Res. 80:188–194. doi:10.1016/j.mvr.2010.03.014.
- Zhou H, Zhang F, Wu Y, Liu H, Duan R, Liu Y, Wang Y, He X, Zhang Y, Ma X, et al. 2022. LRP5 regulates cardiomyocyte proliferation and neonatal heart regeneration by the AKT/P21 pathway. J Cell Mol Med. 26:2981–2994. doi:10.1111/jcmm.17311.
- Zhou XL, Liu JC. 2014. Role of Notch signaling in the mammalian heart. Braz J Med Biol Res. 47:1–10. doi:10.1590/1414-431(20133177.
- Zimmerman ZF, Moon RT, Chien AJ. 2012. Targeting Wnt pathways in disease. Cold Spring Harb Perspect Biol. 4. doi:10.1101/cshperspect.a008086.