ABSTRACT
This investigation delineates the environmental ramifications of the adaptive reuse of historic buildings through a comprehensive life cycle analysis (LCA) posed against traditional demolition and construction approaches. The principal research question probes the extent of environmental conservation achieved through adaptive reuse. Objectives include the empirical quantification of adaptive reuse benefits across five impact categories using a historical edifice in Zabrze, Poland, as a case study. The LCA methodology facilitates a comparative assessment of the benefits of adaptive reuse. The outcomes reveal a substantial diminution in environmental impacts, notably an 82% reduction in global warming, 51% smog formation, 27% acidification, and 21% eutrophication potential. These insights substantiate adaptive reuse as a pivotal strategy for environmental impact mitigation in the construction sector. The study highlights the challenges of maintaining the historical, cultural, and social value of old buildings while improving their sustainability and usability.
1. Introduction
According to ICOMOS, adaptation means the process(es) of modifying a place for a compatible use while retaining its cultural heritage value (Wong, Citation2016). Adaptation processes include alteration and addition. Alternation can be in different forms, such as retrofit, renovation, reconstruction, rehabilitation, and restoration. However, some previous studies have different definitions. For example, Holyoake and Watt (Citation2002) defined adaptation as not a change of use, and Vardopoulos (Citation2019) thinks reuse can be translated to something exceptional and exclusive. To align with its research objective, this paper frames adaptive reuse within the context of the ICOMOS definition and previous studies: it is characterized as a process of converting an existing building to a new use while retaining as much of the original structure as possible and upgrading its performance to meet current standards (Vardopoulos & Theodoropoulou, Citation2018).
Adaptive reuse is a process of renovating existing (old) buildings for new use (Rodrigues & Freire, Citation2017). In Europe, more than one-quarter of the existing building stock has some historical building designation, which will play a critical role in achieving future carbon-neutral goals (Hao et al., Citation2020). Adaptive reuse of historical buildings sits at the intersection of sustainable development and cultural preservation and is inherently complex (Barthel-Bouchier, Citation2016; De et al., Citation2019; Yıldırım & Turan, Citation2012). Since 2016, adaptive reuse has attracted significant attention from the scientific community and general public, with the primary reason being the cost benefits, as reusing existing buildings can be less costly than constructing new buildings (Wilkinson et al., Citation2009). The potential energy savings and environmental benefits are drivers for adaptive reuse projects and research, especially within the context of a circular economy (Marika et al., Citation2021).
Extensive studies have been conducted on the energy efficiency and energy retrofits of historical buildings, with the primary target being to reduce operational carbon (Lidelöw et al., Citation2019). For example, Rodrigues and Freire (Citation2017) studied the energy retrofit strategies (e.g. adding insulation) for a historical building in Portugal; they concluded that exterior-wall insulation with 80 mm and roof without insulation is the most eco-efficient retrofit strategy. Other factors—such as the embodied carbon, cost, culture, and aesthetics of adaptative reuse—are less integrated into the research and policy consideration Field (Lidelöw et al., Citation2019). The benefits of avoided carbon emissions from adaptative reuse have not been widely studied, and quantitative values should be more fully explored (Lidelöw et al., Citation2019), both in the context of adaptive reuse versus demolition/new construction and in evaluating the embodied social and cultural values.
One of the biggest challenges of adaptive reuse is maintaining the historical, cultural, and social value of an old building while improving its usability and sustainability (Djebbour & Wided Biara, Citation2020). This process involves restoring significant structures that would otherwise sit vacant and risk deterioration, as well as gaining community support and increasing interest in a project. Historical buildings often embody traditional construction techniques and materials that are unique to a construction period and geographical location (Rodrigues & Freire, Citation2017). Consequently, a major building retrofit for reuse can be complicated and costly due to the complexity and uncertainty surrounding the existing building. A major retrofit involves the primary building systems upgrade and alteration, such as building envelope replacement or building mechanical system upgrade (Hu & Milner, Citation2020). Therefore, addressing the benefits of a building’s adaptive reuse from a life cycle perspective with quantitative environmental measures can provide crucial evidence for design teams and policy-makers. While life cycle assessments (LCAs) have been extensively used to assess the environmental impacts of new and existing buildings, they have mainly focused on residential buildings (Rodrigues & Freire, Citation2017). However, the application of Life Cycle Assessment (LCA) as an assessment tool for historical building adaptive reuse is limited. (Lidelöw et al., Citation2019) noted that the adaptive reuse of historical buildings contributes to sustainable development, but there is a lack of sufficient evidence detailing the nature of these contributions (Pereira Roders & Van Oers, Citation2014) researched cultural heritage management and found that it primarily focuses on maintaining heritage values. Meanwhile, other studies addressing the environmental sustainability of adaptive reuse tend to focus almost exclusively on energy-efficiency measures; these approaches are not necessarily compatible.
Poland has a considerable number of post-industrial buildings. The nation’s industrial history, particularly from the 19th and 20th centuries, was robust in sectors such as steel, coal, and textiles. The transition to a market economy after the fall of communism, coupled with significant changes in global industrial dynamics, has led to the decline of many of these industrial operations. This decline has resulted in a legacy of post-industrial structures, such as factories, warehouses, and other facilities, especially in regions such as Upper Silesia, which was a center of heavy industry (Pieczka & Wowrzeczka, Citation2021).
In the contemporary context, there has been a growing interest in the adaptive reuse of these buildings in Poland, transforming them into cultural centers, commercial spaces, and residential areas (Pieczka & Wowrzeczka, Citation2021). Adaptive reuse in Poland is part of a broader European trend that values the regeneration of urban spaces with an eye towards sustainability and historical preservation. There has been increasing interest in the adaptive reuse of historical buildings and places of cultural heritage as a catalyst for urban revitalization. The need to revive the architectural heritage of post-industrial cities has been recognized as an important element of cities’ identities (Sowińska-Heim, Citation2020). For example, significant efforts have been made to revitalize Piotrkowska Street in Lodz, a ‘factory city’ and the second-largest city in the former Kingdom of Poland (Haase et al., Citation2021). Numerous post-industrial buildings were converted into mixed-use complexes, art incubators, and educational centers (Sowińska-Heim, Citation2020). Another example is Krakow’s downtown area, which is currently the second-largest city in Poland. Exemplary adaptive reuse projects include the Browar Lubicz project, which converted a small brewery (built in 1840) into a housing and office complex, and a former tin products factory, which was retrofitted into a residential and commercial complex (Gyurkovich & Gyurkovich, Citation2021).
However, in comparison to the active adaptive reuse development in Western European countries, in Poland, adaptive reuse has been adopted at a slower pace and with certain delays, reflecting the general political and historical situation (Gyurkovich & Gyurkovich, Citation2021). Moreover, most projects have focused on the cultural and societal value of historic buildings, despite the fact that the adaptive reuse approach aligns with sustainable development principles by reducing the need for new construction, thereby conserving resources and reducing environmental impact. However, research into the value of adaptive reuse in sustainable development in Poland is very limited. To address this knowledge gap, this study focuses on quantifying the environmental sustainability value of the adaptive reuse of cultural heritage in Poland from a life cycle perspective.
In this study, the definition of a historic building from European standard EN 16,883:2017, ‘Conservation of cultural heritage—Guidelines for improving the energy performance of historic buildings’, was adopted. A historic building refers to any building that is deemed worthy of preservation, regardless of whether it is formally registered. The adaptive reuse of a historic building includes the energy retrofit, rehabilitation, and redevelopment of one or more buildings to reflect the changing needs of society (Foster, Citation2020).
2. Existing literature
The concept of adaptive reuse of buildings extends significant benefits beyond mere climate and resource efficiency. It constitutes a vital contribution to the social and economic fabric, leveraging cultural heritage and community assets. This modality not only augments the commercial viability and diminishes the upkeep expenditures of structures as indicated by Gosling et al. (Citation2013), but it also satisfies local communal necessities and propels sustainable development along economic, social, and environmental spectra (De Silva et al., Citation2019; Wijesiri et al., Citation2022). In addition, adaptive reuse is pivotal in revitalizing the built environment and serves as a fundamental strategy in the pursuit of comprehensive sustainability (Zulkanain et al., Citation2021).
Investigations into the adaptive reuse of buildings have spanned various dimensions. Langston et al. (Citation2008) introduced a set of criteria encompassing physical, economic, functional, technological, social, and legal aspects of obsolescence to elucidate this subject. Remøy and Van Der Voordt (Citation2014) delved into the transformation of office edifices into residential spaces, scrutinizing the attendant opportunities and perils. Çevik et al. (Citation2008) underscored the impetus for spatial re-utilization, particularly given the urban milieu’s insufficiency in fulfilling daily needs, alongside economic and environmental considerations. Shen and Langston (Citation2010) compared adaptive reuse projects in urban versus non-urban settings. Günçe and Mısırlısoy (Citation2019) appraised adaptive reuse practices via user experiences.
When focusing on historical edifices, adaptive reuse embodies distinct benefits and challenges. A comprehensive literature review conducted by Owojori et al. (Citation2021), spanning 2006 to 2021, unearthed 227 studies, predominantly hailing from Italy, the United Kingdom, Australia, China, the United States, and the Netherlands. They concluded these studies coalesced into four thematic clusters: environmental impacts of adaptive reuse, decision-making processes, theoretical frameworks and stakeholder analyses, and contributions to sustainable urban development and neighborhood rejuvenation (Owojori et al., Citation2021). Of these, the environmental cluster boasts a preponderance of quantitative case studies substantiating the environmental advantages of adaptive reuse, often highlighting energy, resource savings, and waste reduction through building repurposing.
The corpus of research on the energy performance of historical buildings post-renovation is extensive, with a spotlight on the benefits of refurbishment strategies, such as building enclosure enhancements (Mosha et al., Citation2022), mechanical system modernizations (Webb, Citation2017), and integration of renewable energy solutions. Mukhopadhyay et al. (Citation2019) discerned that retrofitting a historical building could result in energy savings of up to 81%, with a return on investment occurring within four to eight years. Moreover, Mora and colleagues posited that employing a holistic approach to renovation could transform a historical building into a net-zero energy entity, concurrently achieving an 81% decrease in carbon emissions (Mora et al., Citation2015). Such studies collectively imply the potential for energy and cost savings through the renovation and retrofitting of historical buildings and encompass research on optimization criteria, procedures, policies, and barriers (Besen et al., Citation2020; Galatioto et al., Citation2019; Jahed et al., Citation2020; Roberti et al., Citation2017).
Adaptive reuse has emerged as a sustainable strategy aimed at extending the life cycle of buildings, thus contributing to sustainable development objectives. This strategy requires a rigorous identification and evaluation of the embedded physical life of obsolete historical buildings, which is pivotal for timing adaptive reuse interventions appropriately (Aigwi et al., Citation2018; Mohamed & Alauddin, Citation2016). The efficacy of such interventions, particularly in the redevelopment of underutilized historical structures, has garnered research attention, with a focus on pinpointing and elucidating the key determinants of its effectiveness (Aigwi et al., Citation2018). In tandem, the application of green adaptive reuse, specifically within the confines of UNESCO cities, has been scrutinized. These studies foreground the insights of practitioners garnered through semi-structured interviews, thereby enriching our understanding of the challenges and potentials of this approach (Alauddin et al., Citation2022). The sustainability and feasibility of restoring and adaptively reusing historical edifices have been rigorously evaluated, with notable inquiries into Sikh Era Havelis in Lahore, Pakistan (Farooq & Khalid Qureshi, Citation2020). This recent discourse has highlighted adaptive reuse as not only a conservation tactic but also as a viable alternative to new construction, reflecting its alignment with sustainability and circular economy principles (Broniewicz et al., Citation2023)
However, the exploration of the broader environmental impacts of adaptive reuse, particularly from a life cycle assessment (LCA) standpoint, remains relatively nascent. While some studies have applied LCA methodologies to specific cases—like Rodrigues and (Rodrigues & Freire, Citation2017) examination of a Portuguese house repurposed as an office—the focus has predominantly been on individual structural systems. These studies affirm the sustainability benefits of renovation in terms of embodied energy conservation and carbon emission reduction, yet they are limited in scope and number (Rodrigues & Freire, Citation2017) (Gaspar and Santos Citation2015); (Gaspar & Lobato Santos, Citation2015; Marique & Rossi, Citation2018).
The integration of LCA into adaptive reuse projects is fraught with challenges. A standardized methodology for such LCAs is conspicuously absent (Frossard et al., Citation2023) complicating the quantification of environmental benefits when compared to new constructions. This complexity arises from factors including embodied energy in preexisting structures, the potential for material reuse, and the impact on surrounding ecosystems (Hamida & Hassanain, Citation2020; Kyaw et al., Citation2023). The scarcity of comprehensive environmental performance data for adaptive reuse projects further impedes robust LCAs, emphasizing the necessity for high-quality data (Adi et al., Citation2022; Núñez et al., Citation2013).
Moreover, the environmental dividends of adaptive reuse extend into qualitative realms, including cultural heritage preservation and social inclusion—domains that are challenging to encapsulate and quantify within the traditional LCA framework (Bosone et al., Citation2021; Kyrö & Lundgren, Citation2022). The absence of a standard framework for considering the use phase in circular economy LCAs further muddies the waters, complicating the comprehensive assessment of adaptive reuse’s environmental benefits (André & Björklund, Citation2023).
Existing literature on adaptive reuse and LCA mainly focuses on theoretical constructs, case studies, and qualitative assessments, with a notable lack of robust, quantitative analyses to empirically substantiate the environmental benefits (Bullen & Love, Citation2011; Tu, Citation2020). This gap hampers showcasing the measurable advantages of adaptive reuse through LCA outcomes. Addressing this, the proposed case study provides empirical evidence of adaptive reuse’s environmental benefits, detailing an LCA that quantifies mitigated impacts, particularly in Poland. The study also demonstrates the viability of an integrated LCA—BIM workflow, offering a pioneering methodological blueprint in adaptive reuse.
3. Case study project
According to documents provided by the City Conservation office the main building at 2 ks. Józefa Londzina Street was built in 1879 as a school for boys of steelworker families, with the long sides facing north and south (refer to Figure ). In 1910, a school building for girls was added to the north side of the plot at 1 Niedzialowskiego Street. Initially, the boys’ school was a two-story building with a usable attic. The brick façade is a load-bearing wall sitting on a stone plinth (refer to Figure ). The façade was decorated with brick window lintels, first floor sub-window panels, and brick cornices. Two wooden entrance doors were decorated with stained glass. The three-story east wing attached to the main building was erected in the late nineteenth century. The brick work (e.g. cornices and lintels) and stone plinth mimic the original style of the main building. The last part of the complex to be preserved was the single-story building with a triple-pitched roof on the west side of the historic building. It was built in 1912, and its walls were decorated with a stone plinth and blind arcades on the side elevation. The roof of the main (oldest) part has six dormer windows, with three on each side. There is one dormer on the roof of the three-story east wing. As illustrated in Figures , the interior of building is plastered brick wall, and the floor is either concrete with clay tiles or wood joists with clay tiles (‘Letter from the Municipal Conservator of Monuments Mgr Inż. Arch. Agnieszka Gerlic (under the Authority of the Mayor) BPP-I.410.63.2020 3–25200–2020 Dated 26 August 2020.’, n.d.).
Figure 1. Case project: (a) exterior view credited to Krzysztof Skrzypiec, (b) interior 2nd floor credited to Jakub Świerzawski, (c) interior 1st floor credited to Krzysztof Skrzypiec, (d) ground floor plan.
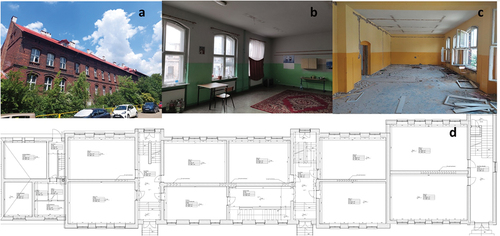
The preserved historical form and style of the building, along with the original brick finishes and decorations, contribute to its recognition as a valuable cultural heritage site in Zabrze. Agnieszka Gerlic, from the Zabrze Conservator Office, has expressed this sentiment, referring to the complex of school buildings as an undeniable asset. The building was officially listed in the municipal registry of monuments in 2018 (Order No. 1138/BZK/2013 of the President of the City of Zabrze on the establishment of the municipal registry of monuments of the City of Zabrze, amended by Order No. 1128/BPP/2016 of 6 December 2016 and Order No. 779/BPP/2018 of 3 October 2018). After the decline of the steel industry, the building was converted into an elementary school for local residents and used until around 2015–2016. Since then it remains empty. The building was acquired by the Academy of Silesia (former name University of Technology in Katowice) in 2020 in order to adapt the building as a faculty building of the Faculty of Medicine in Zabrze.
Designed in 2021 by Tomasz Bradecki of Studio BB Architekci, the architectural adaptation project aims to convert a historical building into a medical simulation center for the Faculty of Medical Sciences at the Academy of Silesia. The functional program, developed by the investor team in consultation with the architects, includes specialized teaching facilities such as seminar rooms, medical labs, and simulation rooms for surgery, obstetrics, and gynecology. Additional facilities include an office, hotel, hygiene rooms, an ambulance garage, technical and storage spaces, and a basement bistro with kitchen facilities. Access to the basement will be from the street and via an internal staircase. Most renovations will occur inside the building, while the exterior will be preserved, repaired, and restored to maintain its cultural significance. This comprehensive adaptation aims to provide students with realistic medical training environments while preserving the historical and cultural integrity of the building (Tomasz Bradecki Architekci, Citation2021).
The urban renewal project in Zabrze is crucial as it addresses both technical and ecological aspects while revitalizing the district. The adjacent workers’ estate also requires renewal, contrasting with the nearby area that hosts cultural and commercial attractions such as a theater, concert hall, shopping mall, restaurants, and the Medical Faculty. According to Polish law sanctioned in 2015, revitalization is a comprehensive process designed to uplift degraded areas, integrating local communities, space, and economy through well-planned programs involving broad participation from residents, property owners, entrepreneurs, and other stakeholders. Zabrze’s strategy is based on the ‘Delimitation Diagnosis for the Purpose of Determining the Degraded Area and Revitalization Area of the City of Zabrze’, which highlights challenges and potential strategies for improvement. The Śródmieście district, where the building is located, has been designated part of the ‘revitalization area’ by the local government due to social problems and unemployment, despite its central location and cultural landmarks. The district faces issues such as building degradation, limited green spaces, and safety concerns. The ‘Municipal Revitalization Program for the City of Zabrze until 2030’, adopted on 15 January 2024, sets social, economic, functional, and spatial goals for the adaptive reuse of 2 Londzina Street. The project aims to enhance urban space competitiveness through the development of scientific, educational, social, and economic functions. Renovating the historic building will bolster Zabrze’s status as an academic hub, supporting the Academy of Silesia Faculty of Medical Sciences. New facilities will provide employment opportunities and support social activities such as conferences, preventive programs, television production, youth training, and scientific research. The renovation will improve the city center’s aesthetic appeal, increase accessibility, and enhance educational quality in the revitalization area, fostering community relationships (Rada miasta Zabrze, Citation2024). The refurbished historic facade is expected to attract tourists, contributing to Zabrze’s post-industrial heritage, including coal mines and water towers, as tourist attractions (‘Zabrze Raport za 2020 rok, Citation2021).
To encapsulate this complex process and avoid ambiguity, which arises from local legislation terms and sometimes vague meanings of revitalization, retrofitting, and rebuilding, the described building process is named adaptive reuse in this article. The intricate nature and potential for urban, social, and economic changes of the described project have been described in detail by (Świerzawski et al., Citation2024, Citationn.d.). There the name ‘revitalization’ was used mainly to align with Polish legislation terms.
4. Methodology and materials
4.1. Research steps, data, and tools
Life Cycle Assessment (LCA) is a systematic analysis of the potential environmental impacts of a product throughout its entire life cycle. This includes the evaluation of environmental impacts during the distribution, use, and end-of-life phases, as well as the upstream and downstream processes associated with the production, use, and disposal of the product (Ilgin & Gupta, Citation2010; ISO, Citation2020). LCA is often considered as support for decision-making within product comparison and optimization applied in the context of the ‘environmental pillar’ of sustainability. The environmental impact assessment workflow, which comprises three steps, is illustrated in Figure .
The first step was to create two BIM models using Autodesk Revit® to represent the historical building and the adaptive reuse project separately. The historical building BIM model was generated based on the original technical drawings and data provided by Studio BB Architekci’s Tomasz Bradecki (who was hired for the adaptive reuse project) to develop an accurate representation of as-built models before adaptive reuse. The adaptive reuse BIM model was created based on the design documents provided by Studio BB Architekci’s Tomasz Bradecki as well. The schedule of material quantities was done in Revit and later used for the LCA.
After creating the two BIM models, the LCA was performed in the second step (refer to 4.2 for a detailed explanation of LCA). The software used for conducting the LCA was Tally®, which has a plug-in interface that is fully integrated in Autodesk Revit®. Tally® complies with the ISO 14,040–14044 LCA requirements (KT Innovations 2015). The LCA calculation method is explained in detail in the LCA Tally® report (refer to supplementary materials). The life cycle inventory database used in this study was derived from the Gabi 2018 database, which is part of the Tally® package.
In the third step, the avoided environmental impacts (measured as percentages) were calculated using the two LCAs from the second step. The avoided environmental impact is derived from the reuse of the building components (e.g. exterior walls and roof) and calculated as follows:
Environmental impact avoidance = Impact from reused historical building components
(e.g. walls)/Total impact of renovated building
4.2. Goal and scope of life cycle assessment
The LCA conducted in this study followed the ISO 14,040 guidelines that comprise three steps: the goal and scope definitions, the inventory analysis and impact assessment (ISO, Citation2020), and the interpretations, which are discussed in section 5.
The goal of the LCA is to assess the avoided environmental impacts through adaptive reuse. As illustrated in Figure , the scope of study includes the building’s primary structure (foundation, load-bearing walls, and roof structure), secondary structure (floors, interior walls, ceiling, and stairs), and enclosure (exterior walls, roof, windows, and doors). This assessment used a building life span of 50 years, which is commonly used in major renovation projects for European buildings (Rodrigues & Freire, Citation2017). It is assumed that the energy supply mix does not change during the whole life span of the building.
Figure 3. LCA system boundary and scope (historical building) (image credited to dieu merci bustseme).
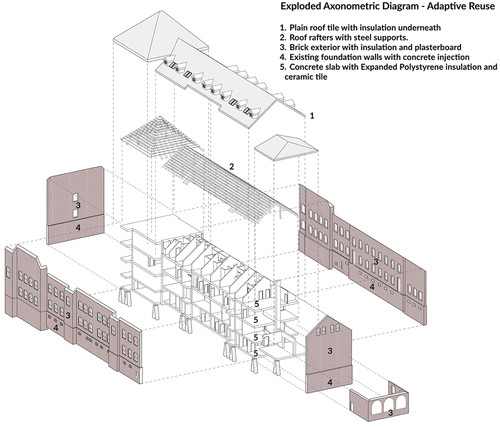
The ISO 14,040 standards define a function unit in LCA as the quantification of the identified function and studied object (de Simone Souza et al., Citation2021). Choosing the appropriate function unit substantially influences the results of the study. Since the goal of the study is to understand the environmental impacts of a building, building performance is often measured by floor area; therefore, the function unit is defined as building gross floor area, in square meters (m2). Four environmental impact categories were used in this study: 1) global warming potential (kg CO2eq/m2), 2) acidification potential (kg SO2eq/m2) (Potting et al., Citation1998), 3) eutrophication potential (Kg PO4eq/m2) (Norris, Citation2002) and, 4) smog formation potential (kg O3eq/m2) (Carter, Citation2008). These are commonly used impact categories within the building and construction sector due to their close attribution to the life cycle impact of buildings (Hu, Citation2019). The life cycle stages included in this study comply with EN 15,978, which are described as follows: product stage (A1–A3), construction stage (A4–A5), use stage (B1–B5), end-of-life stage (C2–C4), and beyond life stage (D) (EN, Citation2012).
4.3. Life cycle inventory analysis
A detailed comparison of the building components, systems, and materials for the historical and adaptive reuse buildings are listed in Table . In the following sections, detailed descriptions are provided for the primary structure, secondary structure, and building enclosure.
Table 1. Building materials and assemblies
4.3.1. Primary structural system
The primary structure of the building encompasses three above-ground floors, a basement level, and an attic accessible via the staircase. The building features a partial basement, underpinned by foundations comprising brick or stone benches, which notably lack insulation in the partial basement walls. A total of 29 open-pit foundations were constructed beneath the load-bearing walls. An expert on-site inspection of the primary structure—including the load-bearing walls, basement, and roof truss—was carried out in 2021 by Fullbet Pracownia Projektowa based in Katowice. The assessment concluded that there was no significant loosening or cracking in the basement and foundation elements.
Even though the building inspector determined the existing ground conditions in the building’s foundation to be adequate, due to the increased load associated with adaptive reuse, it is necessary to strengthen the existing foundation by using a technique called jet grouting. This is a ground improvement method that creates structural elements by injecting a high-pressure jet of grout (a mixture of cement, water, and other additives) into the soil to create soil cement composite structural piles (Burke, Citation2004). The existing stone and brick foundation will be topped with 80 cm diameter jet-grouting columns at 40 cm intervals. Partition walls and part of the load-bearing walls in the basement will be removed. Additionally, a lift shaft will be introduced, and the stairs will be replaced, as the existing ones do not meet modern code requirements. The depth of the column foundation is recommended to end in the bearing ground approximately 6 meters below the level of the existing foundation, which will increase the loads transferred to the foundation.
The main load-bearing structure (aboveground) of the building consists of solid brick walls. The basic load-bearing system of the building is a two-bay structure, divided by two internal staircases and one exterior staircase. The load-bearing brick wall was constructed with more than three layers of bricks without a cavity and mortar in between layers (Figure ). There are three thickness levels of brick walls: three layer (44 cm), four layer (56 cm), and five layer (73 cm) As shown in Figure ), a wooden roof truss was built as a hanger system: two hangers with an elbow wall with additional support of the ridge purlins on the strut of the hanger.
Figure 4. Building primary structural system: (a) three-layer brick, (b) wooden roof truss (photo credited to K,Skrzypiec).
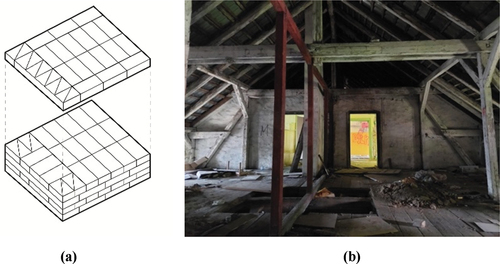
The 2021 inspection revealed numerous cracks in window and door lintels, brick vault wedging, and erosion of external walls. Expert opinions suggest that the building can be adapted for a new function if these damages are reinforced and repaired. Cracks should be stitched, filled, or rebricked. Restoration must preserve the building’s stylistic features, with cavities repaired, the brick façade cleaned, and the roof structure and covering replaced to maintain existing geometry and restore dormers, according to city council guidelines.
4.3.2. Secondary structural system
The original ceilings of the aboveground part of the building were made of wood and are laid out based on the longitudinal walls, with the exception being parts within staircases where the ceilings are based on transverse walls separating staircases. Basement ceilings were made in the form of brick vaults based on walls or brick arches. The interior walls in the historical building were made of solid brick as well, and they will be completely replaced by metal stud walls with one layer of gymnasium board on each side (refer to Table ).
The 2021 inspection found some damage in the historical wooden ceiling structures. As a result, it was recommended that the old wooden ceilings be replaced with either ribbed reinforced concrete ceilings or ribbed ceilings with steel ribs, onto which a reinforced concrete floor slab would be placed. It is possible to preserve and utilize the existing wooden beam sockets for embedding the new ceiling ribs, thereby achieving a relatively light ceiling construction. Ceilings above the basement floor are to be constructed as reinforced concrete, either monolithic, flat, or ribbed. These measures will ensure the preservation of the structural integrity of the building while also providing a sound and durable solution for its future use.
The original wooden floor in the historical building will be replaced with a concrete floor with wood decking, and the original wood stairs will be replaced with concrete stairs.
4.3.3. Building enclosure system
The building enclosure consists of the exterior walls, windows, doors, and roof. The exterior wall currently lacks insulation. The original double-layer casement window featured a wooden frame, with an air gap measuring approximately 10 cm between the two layers. Plans have been made to replace the window frames with white Polyvinyl Chloride (PVC), which will replicate the original frames in terms of geometry, dimensions, and divisions. The wooden entrance doors are slated to be preserved through cleaning or restoration efforts. To facilitate access for an ambulance vehicle, a garage door will be added to the single-story building on the west side, with the bricked-up arcade walls being replaced with glazing. The historical roofing material of the building is made of clay tile, which will be replaced with a new clay tile roof featuring additional insulation. These measures will enhance the building’s energy efficiency and promote sustainable building practices while also preserving its historical characteristics (refer to Table ).
5. Results
5.1. Life cycle environmental impact of building systems
As illustrated in Figure , the building enclosure (including the exterior walls and roof) dominates in all environmental impact indicators except eutrophication potential, due to its use of a brick masonry wall. In sum, the building enclosure contributes to approximately 86% of global warming potential, 72% of smog formation potential, and 64% of acidification potential. Therefore, preserving and maximizing the reuse of the existing building’s exterior walls and roof system can help to avoid significant environment impacts accumulated in the demolition and building of the new building’s enclosure.
The superstructure, which refers to the primary and secondary structural systems, is the dominating contributor to eutrophication potential (53%). Eutrophication is the measure of the impacts of excessively high levels of macronutrients, the most important of which are nitrogen and phosphorus (Conley et al., Citation2009). Nutrient enrichment can lead to significant alterations in species composition and increased biomass production in both aquatic and terrestrial ecosystems, with potentially negative consequences (Dorgham, Citation2014). In this adaptive reuse project, most of the superstructure is preserved, with limited enhancements to the foundation, hence related environmental impacts can be avoided.
In the historical building, the timber frame (roof) and wood columns are two primary sources of eutrophication potential; together, they contribute to approximately 24% of the environmental burdens of eutrophication potential. Particularly high single contributions due to those two components also occur with acidification (11%). The relevance of the wood frame and columns is remarkable, as the mass contribution of this material to the whole building materials amounts to less than 2%. One potential explanation of wood’s major contribution to eutrophication potential is the treatment of the wood. The pressure treatment of wood was first developed in the mid-nineteenth century as a means of protecting wood from decay and insect damage. The earliest recorded patent for pressure-treated wood was granted in 1838 to John Bethell, a British inventor who developed a process for impregnating wood with a solution of copper sulfate and arsenic trioxide under pressure (Freeman et al., Citation2003). Over time, pressure treatment techniques have been refined and improved, with new chemicals and processes developed to enhance the durability and performance of treated wood products. Today, pressure-treated wood is widely used in construction, landscaping, and other applications where durability and resistance to decay and insect damage are important.
5.2. Life cycle stage contribution
The life cycle stages of the building present varied environmental implications as depicted in Figure . The end-of-life stage, including transportation to disposal, waste processing, and final disposal activities (C2–C4 according to EN 15,978), imposes considerable environmental impacts. It is responsible for 16% of both global warming and smog formation potentials, and an even more pronounced 23% and 46% of acidification and eutrophication potentials, respectively. The substantial role of this life cycle stage accentuates the environmental significance of each phase in the building’s lifespan.
5.3. Life cycle environmental impact of building components
When examining the building systems and components, it is not surprising that the walls (exterior and interior walls) are the primary contributor to all environmental impact categories, especially global warming potential (85%). The dominating effect can be explained by the quantities of materials used—as shown in the left bar in Figure , walls account for 83% of the total mass (measured by weight, kg) of the building, hence a predetermined factor to environmental impacts. The second biggest contributor is the floor system, also mainly due to the material quantities and types. Different floor materials are used in the historical building, including concrete, brick, wood, and clay tiles (refer to Table ), altogether accounting for 12% of the building’s total mass, consequently contributing to environmental impact categories in a similar portion: 10% of global warming potential, 15% of acidification potential, 22% of eutrophication potential, and 13% of smog formation potential. The third contributor worth mentioning is the (roof) structural system—by weight, it accounts for less than 2% of the building, but it contributes to 13% of acidification potential, 29% of eutrophication potential, and 8% of smog formation potential. This effect is mainly associated with the types of materials used in the roof structure. As illustrated in Figure , the historical building’s roof system comprises several components: thin clay tiles, wood rafters, and wood purlins. Compared to brick and concrete, wood weighs much less (kg), but the volume (measured in cubic feet) is not small.
5.4. Avoided environmental impacts
Figure shows the avoided environmental impacts through adaptive reuse, which are calculated according to the method described in section 4. The avoid impacts are mainly associated with the reuse of existing materials, such as masonry walls and the foundation. These strategies can reduce the environmental impact in all life cycle stages, A1–C. Among all categories, global warming potential sees the largest benefit, with 82% avoided, followed by smog formation potential (51%), acidification potential (27%), and eutrophication potential (21%); the effect on ozone depletion potential is negligible. According to these findings, the research team also reached the preliminary conclusion that adaptative reuse significantly contributes to reducing the built environment’s impact by avoiding unnecessary new construction activities. The quantifiable benefit is particularly obvious in avoided global warming potential, at over 80%.
6. Discussion
6.1. Comparative analysis with existing literature
The environmental implications observed in our study are contextualized within the broader discourse of sustainable development and conservation practices. The preservation of the superstructure, which significantly mitigates the eutrophication potential, resonates with the findings of Conley et al. (Citation2009) regarding nutrient enrichment and its ecological ramifications. The contributions of wood treatments to eutrophication and acidification potentials align with historical accounts of wood preservation methods and their environmental impact, as discussed by Freeman et al. (Citation2003)
Our examination of the building’s life cycle stages has elucidated that the end-of-life stage, inclusive of disposal processes, is a substantial contributor to environmental impacts. This finding is consistent with Quéheille et al. (Citation2022), who highlight the variability and significance of demolition processes in life cycle assessments. The material contributions analysis further aligns with the principles of material conservation and the push towards resource efficiency in sustainable architecture.
6.2. Synthesis of findings and their implications
In synthesizing the results, we observe a significant reduction in various environmental impacts through the strategic preservation and reuse of existing building elements. These observations are in concert with the broader literature that advocates for adaptive reuse as a means to achieve environmental sustainability goals (Rodrigues & Freire, Citation2017). The reduction in global warming potential by 82% is particularly noteworthy, as it corroborates the assertions by Mukhopadhyay et al. (Citation2019) regarding the potential for energy savings through building retrofitting.
6.3. Sensitivity analysis
The sensitivity analysis was conducted to estimate the uncertainty in the LCA results due to input data quality and assumptions. Using a linear regression model approach (Ardente et al., Citation2005), three variables were investigated: i) quantities of reused and preserved materials, focusing on wood and concrete; ii) material quality, maintenance, and replacement (B2–B5); and iii) resource availability, indicated by transportation (A4). The additional concrete foundation in the adaptive reuse program accounts for the new building’s structural load, which can vary with different functions, affecting the quantity of added concrete. The same applies to the added wood for new floors and interior walls. Material changes were set at −5% to 10% in volume changes by mass. Figure shows changes to the concrete foundation have a more significant impact on global warming potential than wood materials. Increased raw material availability impacts transportation distance and method, adding uncertainty to the environmental impact, primarily reflected in life stage A4. Transportation distance changes were also set at −5% to 10% (refer to Figure ). Additionally, raw material availability influences the quality of building materials, affecting maintenance and replacement needs. The analysis showed that transportation distance has a more significant impact on global warming potential compared to maintenance and replacement needs.
6.4. Contribution and limitation
The adaptive reuse of historical buildings, as demonstrated by our life cycle environmental impact assessment, offers a tangible reduction in environmental impacts, supporting the notion that such practices are not only culturally and historically significant but also environmentally beneficial. The findings from this study contribute to the growing body of evidence that underscores the importance of adaptive reuse in sustainable urban development and offers insights that could inform policy and regulatory frameworks.
This study has three limitations. First, the energy supply mix is assumed to stay the same during the building’s whole life span, which is not the case in real life. Changes in the energy supply resources and mix can have a significant effect on a building’s embodied energy; for example, the energy supply can directly impact the carbon emissions associated with energy used in building production, transportation, and other life cycle stages. Second, multiple assumptions have been made about the historical building due to a lack of both complete documents and an understanding of historical construction methods, hence the accuracy of the assessment can be affected by those assumptions. In addition, the research team attempted to verify the historical building’s construction details with building engineers working on the project, but confirmation was not obtained. In the next step of the study, the research team can use uncertainty analysis to understand the different historical construction methods and materials. The third limitation is related to the scope of the study. This study excludes C1 (demolition) in the proposed adaptive reuse design; most interior floors and walls will be demolished and rebuilt; and they are made of wood. The exclusion of C1 stage is mainly caused by the lack of data on demolition techniques used in the project. According to previous studies and other literature, the end-of-life stage, especially the demolition stage, is not included since C1 only has a marginal contribution to the environmental impacts of a building’s life cycle (Ivanica et al., Citation2022), ranging between 1% to 3% (Ortiz et al., Citation2010; Quéheille et al., Citation2022). In addition, compared to other end-of-life stages (C2-C4), C1 has (Quéheille et al., Citation2022; Zakerhosseini et al., Citation2023). Because of the minor influence from the C1 stage, the exclusion of C1 has a minimal impact on the analysis results.
7. Conclusion
The conclusion of this study encapsulates the significant environmental benefits conferred by the adaptive reuse of historical buildings in Poland, as substantiated through a life cycle assessment (LCA) methodology. The empirical evidence presented, derived from a comprehensive comparison of building components, systems, and materials for the historical and adaptive reuse scenarios, suggests that adaptive reuse can effectively reduce environmental impacts across all life cycle stages (A—C).
Specifically, the LCA results indicate that the adaptive reuse strategy, which centers on the preservation of existing materials such as masonry walls and the foundation, has yielded a substantial reduction in environmental impacts. The empirical evidence demonstrates that adaptive reuse can result in an 82% reduction in global warming potential, a 51% reduction in smog formation potential, a 27% reduction in acidification potential, and a 21% reduction in eutrophication potential. Notably, the effect on ozone depletion potential was found to be negligible. These findings are pivotal, as they elucidate that adaptive reuse can significantly diminish the built environment’s impact by averting unnecessary new construction activities, with the most pronounced benefit observed in the reduction of global warming potential. Such empirical analysis based on an actual project starts to fill the knowledge gap about the environmental benefit of adaptive reuse in Poland.
Further reinforcing these findings, a sensitivity analysis was conducted to address the uncertainty inherent in LCA results, which may arise from the quality of input data and the assumptions utilized within the LCA. By employing a linear regression model, this analysis scrutinized variables such as the quantities of materials reused (particularly focusing on wood and concrete), the quality of materials in terms of their maintenance and replacement cycles, and the availability of resources impacting transportation. The sensitivity analysis thus provides a nuanced understanding of the factors influencing the LCA outcomes, offering additional depth to the empirical evidence and bolstering the study’s scientific contribution.
The case study’s scientific contributions are threefold: it quantifies the environmental benefits of adaptive reuse in a post-industrial city in Poland, it enhances the methodological framework for environmental impact assessment in the field of sustainable urban development, and it offers a replicable model for future empirical studies aiming to support policy and regulatory development. The study’s methodological advancements, particularly the application of the BIM-LCA workflow, present a significant stride towards the standardization of environmental impact assessments in adaptive reuse projects, thereby informing sustainable practices and policy at a critical juncture of urban development and environmental conservation.
Public interest statement
This investigation examines the environmental impact of adaptive reuse of historic buildings through a comprehensive life cycle analysis (LCA) compared to traditional demolition and construction. The main research question explores the environmental conservation achieved through adaptive reuse. Objectives include quantifying the benefits of adaptive reuse across five impact categories, using a historical building in Zabrze, Poland, as a case study. The LCA method enables a comparative assessment of adaptive reuse’s advantages. The findings show significant reductions in environmental impacts: 82% in global warming, 51% in smog formation, 27% in acidification, and 21% in eutrophication potential. These results confirm adaptive reuse as a crucial strategy for reducing environmental impacts in the construction sector. The study also addresses the challenges of preserving the historical, cultural, and social value of old buildings while enhancing their sustainability and usability.
Author bio.docx
Download MS Word (19.8 KB)Ming Hu Jakub Swierzawski photo.jpg
Download JPEG Image (1.4 MB)Acknowledgements
M.H. and J.S. designed and directed the project; M.H. analyzed data; J.S. collected the data; M.H and J.S. wrote the article.
Disclosure statement
No potential conflict of interest was reported by the author(s).
Data availability statement
Necessary data have been included in the manuscript.
Supplementary material
Supplemental data for this article can be accessed online at https://doi.org/10.1080/27658511.2024.2375439
Correction Statement
This article has been corrected with minor changes. These changes do not impact the academic content of the article.
Additional information
Notes on contributors
Ming Hu
Ming Hu is an Associate Dean for Research and Associate Professor at the School of Architecture and at the College of Engineering at the University of Notre Dame, USA. An architect and environmental engineer, Dr. Hu specializes in decarbonizing the built environment through net-zero impact and healthy building design, emphasizing life cycle impact and human health-centered decarbonization of the US building stock.
Jakub Świerzawski
Dr. Jakub Świerzawski is the Dean of the Faculty of Architecture, Civil Engineering, and Applied Arts at the Academy of Silesia (Katowice, Poland). His research focuses on contemporary architecture within its cultural and technological contexts, particularly in Poland and the Silesia region. Dr. Hu and Dr. Świerzawski co-founded a Polish-American research team “Zero-Emission Built Environments and Heritage Research Alliance” (zebra.academyofsilesia.com). ZEBRA integrates architecture, culture, history, and engineering to promote sustainable urban development, aiming to create resilient and culturally rich environments that balance innovation and sustainability..
References
- Adi, B. K., Joko, T., & Setiani, O. (2022). Life cycle assessment, is it beneficial for environmental sustainability? A literature review. Jurnal Serambi Engineering, 7(3). https://doi.org/10.32672/jse.v7i3.4349
- Aigwi, I. E., Egbelakin, T., & Ingham, J. (2018). Efficacy of adaptive reuse for the redevelopment of underutilised historical buildings: Towards the regeneration of New Zealand’s provincial town centres. International Journal of Building Pathology and Adaptation, 36(4), 385–17. https://doi.org/10.1108/IJBPA-01-2018-0007
- Alauddin, K., Najwa Mohd Nusa, F., Nurfaisal Baharuddin, M., Sabrizaa Abdul Rashid, M., & Remeli@ramli, R. (2022). The APPLICATION of GREEN ADAPTIVE REUSE of HISTORICAL BUILDINGS in UNESCO CITIES. Planning Malaysia, 20. https://doi.org/10.21837/pm.v20i22.1137
- André, H., & Björklund, A. (2023). A framework to open the black box of the use phase in circular economy life cycle assessments: The case of shell jacket reuse. Journal of Industrial Ecology, 27(4), 1137–1150. https://doi.org/10.1111/jiec.13408
- Ardente, F., Beccali, G., Cellura, M., & Lo Brano, V. (2005). Life cycle assessment of a solar thermal collector: Sensitivity analysis, energy and environmental balances. Renewable Energy, 30(2), 109–130. https://doi.org/10.1016/j.renene.2004.05.006
- Barthel-Bouchier, D. (2016). Cultural heritage and the challenge of sustainability. Routledge.
- Besen, P., Boarin, P., & Haarhoff, E. (2020). Energy and seismic retrofit of historic buildings in New Zealand: Reflections on current policies and practice. The Historic Environment: Policy & Practice, 11(1), 91–117. https://doi.org/10.1080/17567505.2020.1715597
- Bosone, M., De Toro, P., Fusco Girard, L., Gravagnuolo, A., & Iodice, S. (2021). Indicators for ex-post evaluation of cultural heritage adaptive reuse impacts in the perspective of the circular economy. Sustainability, 13(9), 4759. https://doi.org/10.3390/su13094759
- Broniewicz, E., Broniewicz, M., Skubiak, B., Bryliński, A., & Grabowska, P. (2023). Adaptive reuse of buildings. Ekonomia i Środowisko - Economics and Environment, 83(4), 338–357. https://doi.org/10.34659/eis.2022.83.4.575
- Bullen, P. A., & Love, P. E. D. (2011). Adaptive reuse of heritage buildings. Structural Survey, 29(5), 411–421. https://doi.org/10.1108/02630801111182439
- Burke, G. K. (2004). Jet grouting systems: Advantages and disadvantages. In GeoSupport 2004: Drilled Shafts, Micropiling, Deep Mixing, Remedial Methods, and Specialty Foundation Systems (pp. 875–886). https://doi.org/10.1061/40713(2004)75
- Carter, W. (2008). https://citeseerx.ist.psu.edu/document?repid=rep1&type=pdf&doi=f3b34d48c1a9c1c260105cffb743efd52f50d72f
- Çevik, S., Vural, S., Tavşan, F., & Aşık, Ö. (2008). An example to renovation–revitalization works in historical city centres: Kunduracılar Street/Trabzon-Turkey. Building and Environment, 43(5), 950–962. https://doi.org/10.1016/j.buildenv.2006.10.053
- Conley, D. J., Paerl, H. W., Howarth, R. W., Boesch, D. F., Seitzinger, S. P., Havens, K. E., Lancelot, C., & Likens, G. E. (2009). Controlling Eutrophication: Nitrogen and Phosphorus. Science, 323 (5917), 1014–15. https://doi.org/10.1126/science.1167755
- De, M., Stefania, P., De, T., & Nocca, F. (2019). Cultural heritage and sustainable development: Impact assessment of two adaptive reuse projects in Siracusa, sicily. Sustainability, 12(1), 311. https://doi.org/10.3390/su12010311
- De Silva, G. D. R., Perera, B. A. K. S., & Rodrigo, M. N. N. (2019). Adaptive reuse of buildings: The case of Sri Lanka. Journal of Financial Management of Property and Construction, 24(1), 79–96. https://doi.org/10.1108/JFMPC-11-2017-0044
- de Simone Souza, H. H., de Abreu Evangelista, P. P., Medeiros, D. L., Albertí, J., Fullana-I-Palmer, P., Boncz, M. Á., & Gonçalves, J. P. (2021). Functional unit influence on building life cycle assessment. The International Journal of Life Cycle Assessment, 26(3), 435–454. https://doi.org/10.1007/s11367-020-01854-1
- Djebbour, I., & Wided Biara, R. (2020). The challenge of adaptive reuse towards the sustainability of heritage buildings. International Journal of Conservation Science, 11(2), 519–530.
- Dorgham, M. M. (2014). Effects of Eutrophication. In Eutrophication: Causes, Consequences and Control: (Vol. 2, pp. 29–44). Springer.https://doi.org/10.1007/978-94-007-7814-6_3
- EN. (2012). BS EN 15978: 2011 Sustainability of construction works. assessment of environmental performance of buildings. calculation method (British standard. https://www.en-standard.eu/bs-en-15978-2011-sustainability-of-construction-works-assessment-of-environmental-performance-of-buildings-calculation-method/
- Farooq, D. S., & Khalid Qureshi, A. (2020). Sustainable viability for restoration and adaptive reuse of Sikh era havelis in Lahore, Pakistan: Sustainable viability for restoration. Sir Syed University Research Journal of Engineering & Technology, 10(2). https://doi.org/10.33317/ssurj.162
- Foster, G. (2020). Circular economy strategies for adaptive reuse of cultural heritage buildings to reduce environmental impacts. Resources, Conservation and Recycling, 152(January), 104507. https://doi.org/10.1016/j.resconrec.2019.104507
- Freeman, M. H., Shupe, T. F., Vlosky, R. P., & Barnes, H. M. (2003). Past, present, and future of the wood preservation industry. Forest Products Journal, 53(10), 8–15.
- Frossard, M., Lasvaux, S., Petetin, F., & Gross, L. (2023). Reuse practices in building construction: Proposition of a life cycle assessment methodology and application to a case study in Switzerland. Journal of Physics: Conference Series, 2600(15), 152007. https://doi.org/10.1088/1742-6596/2600/15/152007
- Galatioto, A., Ricciu, R., Salem, T., & Kinab, E. (2019). Energy and economic analysis on retrofit actions for Italian Public Historic Buildings. Energy, 176(June), 58–66. https://doi.org/10.1016/j.energy.2019.03.167
- Gaspar, P. L., & Lobato Santos, A. (2015). Embodied energy on refurbishment vs. Demolition: A southern Europe case study. Energy and Buildings, 87(January), 386–394. https://doi.org/10.1016/j.enbuild.2014.11.040
- Gosling, J., Sassi, P., Naim, M., & Lark, R. (2013). Adaptable buildings: A systems approach. Sustainable Cities and Society, 7(July), 44–51. https://doi.org/10.1016/j.scs.2012.11.002
- Günçe, K., & Mısırlısoy, D. (2019). Assessment of adaptive reuse practices through user experiences: traditional houses in the walled City of Nicosia. Sustainability, 11(2), 540. https://doi.org/10.3390/su11020540
- Gyurkovich, M., & Gyurkovich, J. (2021). New housing complexes in post-industrial areas in city centres in Poland versus cultural and natural heritage protection—with a particular focus on cracow. Sustainability, 13(1), 418. https://doi.org/10.3390/su13010418
- Haase, A., Bontje, M., Couch, C., Marcinczak, S., Rink, D., Rumpel, P., & Wolff, M. (2021). Factors driving the regrowth of European cities and the role of local and contextual impacts: a contrasting analysis of regrowing and shrinking cities. Cities, 108(January), 102942. https://doi.org/10.1016/j.cities.2020.102942
- Hamida, M. B., & Hassanain, M. A. (2020). Post occupancy evaluation of adaptively reused buildings: Case study of an office building in Saudi Arabia. Architecture, Civil Engineering, Environment, 13(1), 29–40. https://doi.org/10.21307/acee-2020-003
- Hao, L., Herrera-Avellanosa, D., Del Pero, C., & Troi, A. (2020). What are the implications of climate change for retrofitted historic buildings? A literature review. Sustainability, 12(18), 7557. https://doi.org/10.3390/su12187557
- Holyoake, K., & Watt, D. (2002). The sustainable re-use of historic urban industrial buildings: interim results and discussion. In, 1(2), 1–17.
- Hu, M. (2019). Life-Cycle environmental assessment of energy-retrofit strategies on a campus scale. Building Research & Information. Routledge. 48(6), 659–680. https://doi.org/10.1080/09613218.2019.1691486
- Hu, M., & Milner, D. (2020). Factors influencing existing medium-sized commercial building energy retrofits to achieve the net zero energy goal in the United States. Building Research & Information. Routledge. 49(5), 525–542. https://doi.org/10.1080/09613218.2020.1798208
- Ilgin, M. A., & Gupta, S. M. (2010). Environmentally conscious manufacturing and product recovery (ecmpro): A review of the state of the art. Journal of Environmental Management, 91(3), 563–591. https://doi.org/10.1016/j.jenvman.2009.09.037
- ISO. (2020). ISO 14044: 2006/Amd 2: 2020 environmental management — life cycle assessment — requirements and guidelines — amendment 2. iSO. https://www.iso.org/standard/76122.html
- Ivanica, R., Risse, M., Weber-Blaschke, G., & Richter, K. (2022, March). Development of a life cycle inventory database and life cycle impact assessment of the building demolition stage: A case study in Germany. Journal of Cleaner Production, 338, 130631. https://doi.org/10.1016/j.jclepro.2022.130631
- Jahed, N., Aktaş, Y. D., Rickaby, P., & Güliz Bilgin Altınöz, A. (2020). Policy Framework for Energy Retrofitting of Built Heritage: A Critical Comparison of UK and Turkey. Atmosphere, 11(6), 674. https://doi.org/10.3390/atmos11060674
- Kyaw, K. S., Fufa, S. M., & Kraniotis, D. (2023). Adaptive reuse of industrial heritage building – comparative life cycle assessment using a case study in Norway. IOP Conference Series: Earth and Environmental Science 1196 (Vol. 1, pp. 012107). https://doi.org/10.1088/1755-1315/1196/1/012107
- Kyrö, R., & Lundgren, R. (2022). Your vibe attracts your tribe – the adaptive reuse of buildings delivering aesthetic experience and social inclusion. IOP Conference Series: Earth and Environmental Science 1101 (Vol. 6. pp. 062014). https://doi.org/10.1088/1755-1315/1101/6/062014
- Langston, C., Francis, K. W., Wong, E., Hui, C. M., & Shen, L.-Y. (2008). Strategic assessment of building adaptive reuse opportunities in Hong Kong. Building & Environment, 43(10), 1709–1718. https://doi.org/10.1016/j.buildenv.2007.10.017
- Lidelöw, S., Örn, T., Luciani, A., & Rizzo, A. (2019). Energy-efficiency measures for heritage buildings: A literature review. Sustainable Cities and Society, 45(February), 231–242. https://doi.org/10.1016/j.scs.2018.09.029
- Marika, G., Beatrice, M., & Francesca, A. (2021). Adaptive reuse and sustainability protocols in Italy: Relationship with circular economy. Sustainability, 13(14), 8077. https://doi.org/10.3390/su13148077
- Marique, A.-F., & Rossi, B. (2018). Cradle-to-grave life-cycle assessment within the built environment: Comparison between the refurbishment and the complete reconstruction of an office building in Belgium. Journal of Environmental Management, 224(October), 396–405. https://doi.org/10.1016/j.jenvman.2018.02.055
- Mohamed, N., & Alauddin, K. (2016). The criteria for decision making in adaptive reuse towards sustainable development. In S. N. B. Kamaruzzaman, A. S. B. Ali, N. F. B. Azmi, & S. J. L. Chua (Eds.). Proceedings of the MATEC Web of Conferences (Vol. 66, pp. 00092). https://doi.org/10.1051/matecconf/20166600092
- Mora, T., Dalla, F. C., Peron, F., Romagnoni, P., & Bauman, F. (2015). Retrofit of an historical building toward NZEB. Energy Procedia, 78(November), 1359–1364. https://doi.org/10.1016/j.egypro.2015.11.154
- Mosha, Z., Mehra, S.-R., & Künzel, H. M. (2022). Energy-saving potential of deeply retrofitting building enclosures of traditional courtyard houses – a case study in the Chinese hot-summer-cold-winter zone. Building and Environment, 217(June), 109106. https://doi.org/10.1016/j.buildenv.2022.109106
- Mukhopadhyay, J., Ore, J., & Amende, K. (2019). Assessing housing retrofits in historic districts in Havre Montana. Energy Reports, 5(November), 489–500. https://doi.org/10.1016/j.egyr.2019.03.008
- Norris, G. A. (2002). Impact characterization in the tool for the reduction and assessment of chemical and other environmental impacts: Methods for acidification, eutrophication, and ozone formation. Journal of Industrial Ecology, 6. Wiley Online Library: 3–4), 79–101. https://doi.org/10.1162/108819802766269548
- Núñez, M., Pfister, S., Roux, P., & Antón, A. (2013). Estimating water consumption of potential natural vegetation on global dry lands: Building an LCA framework for green water flows. Environmental Science & Technology, 47(21), 12258–12265. https://doi.org/10.1021/es403159t
- Ortiz, O., Pasqualino, J. C., & Castells, F. (2010). Environmental performance of construction waste: Comparing three scenarios from a case study in Catalonia, Spain. Waste Management, 30(4), 646–654. https://doi.org/10.1016/j.wasman.2009.11.013
- Owojori, O., Okoro, C., & Chileshe, N. (2021). Current status and emerging trends on the adaptive reuse of buildings: a bibliometric analysis. Sustainability, 13(21), 11646. https://doi.org/10.3390/su132111646
- Pereira Roders, A., & Van Oers, R. (2014). Wedding cultural heritage and sustainable development: Three years after. Journal of Cultural Heritage Management and Sustainable Development, 4(1). Emerald Group Publishing Limited: 2–15. https://doi.org/10.1108/JCHMSD-04-2014-0015
- Pieczka, M., & Wowrzeczka, B. (2021). Art in post-industrial facilities—strategies of adaptive reuse for art exhibition function in Poland. Buildings, 11(10), 487. https://doi.org/10.3390/buildings11100487
- Potting, J., Schöpp, W., Blok, K., & Hauschild, M. (1998). Site-dependent life-cycle impact assessment of acidification. Journal of Industrial Ecology, 2(2), 63–87. https://doi.org/10.1162/jiec.1998.2.2.63
- Quéheille, E., Ventura, A., Saiyouri, N., & Taillandier, F. (2022). A life cycle assessment Model of end-of-life scenarios for building deconstruction and waste management. Journal of Cleaner Production, 339(March), 130694. https://doi.org/10.1016/j.jclepro.2022.130694
- Rada miasta Zabrze. (2024). Uchwała Nr LXXVII/1020/24 Rady Miasta Zabrze z dnia 15 stycznia 2024 r. w sprawie przyjęcia Gminnego Programu Rewitalizacji Miasta Zabrze do 2030 roku. Zabrze City Council.
- Remøy, H., & Van Der Voordt, T. (2014). Adaptive reuse of office buildings into housing: Opportunities and risks. Building Research & Information, 42(3), 381–390. https://doi.org/10.1080/09613218.2014.865922
- Roberti, F., Filippi Oberegger, U., Lucchi, E., & Troi, A. (2017). Energy retrofit and conservation of a historic building using multi-objective optimization and an analytic hierarchy process. Energy & Buildings, 138. Elsevier: 1–10. https://doi.org/10.1016/j.enbuild.2016.12.028
- Rodrigues, C., & Freire, F. (2017). Adaptive reuse of buildings: Eco-efficiency assessment of retrofit strategies for alternative uses of an historic building. Journal of Cleaner Production, 157(July), 94–105. https://doi.org/10.1016/j.jclepro.2017.04.104
- Shen, L., & Langston, C. (2010). Adaptive reuse potential: an examination of differences between urban and non‐urban projects. Facilities, 28(1/2), 6–16. https://doi.org/10.1108/02632771011011369
- Sowińska-Heim, J. (2020). Adaptive reuse of architectural heritage and its role in the post-disaster reconstruction of urban identity: Post-communist Łódź. Sustainability, 12(19), 8054. https://doi.org/10.3390/su12198054
- Świerzawski, J., Ming, H., Justyna, K., & Piotr, K. (2024). The transformation of post-industrial heritage: cultural, urban, energy and environmental benefit. Case study from Zabrze, Poland. AMPS PROCEEDINGS SERIES (Vol. 35. pp. 455–463). AMPS, Prague, Czechia. https://amps-research.com/wp-content/uploads/2024/04/Amps-Proceedings-Series-35.2_2024.pdf.” In, Ed. J. Cirklová.
- Świerzawski, J., Ming, H., Justyna, K., & Piotr, K. (n.d.). The potential of higher education institutions as catalysts for revitalization in urban planning: A case study of a medical simulation centre at the workers’ housing estate of donnersmark ironworks in Zabrze. Środowisko Mieszkaniowe/Housing Environment, 2, 92–99. https://doi.org/10.4467/25438700SM.23.023.18484
- Tomasz Bradecki Architekci, S. B. (2021). doi: “Budynek Wydziału Nauk Medycznych Im. Prof. Zbigniewa Religii w Zabrzu - Rewitalizacja Obiektu Byłej Szkoły w Zabrzu Ul. Ks. Józefa Londzina 2 z Nadaniem Nowych Funkcji Publicznych/Edukacyjnych w Ramach Wyższej Szkoły Technicznej w Katowicach Poprzez Utworzenie Nowych Laboratoriów i Centrum Symulacji Medycznej, Construction Project Authorized by Construction Permit Decision No, (1), 92–100. https://doi.org/10.4467/25438700SM.23.023.18484
- Tu, H.-M. (2020). The attractiveness of adaptive heritage reuse: a theoretical framework. Sustainability, 12(6), 2372. https://doi.org/10.3390/su12062372
- Vardopoulos, I. (2019). Critical sustainable development factors in the adaptive reuse of urban industrial buildings. A fuzzy DEMATEL approach. Sustainable Cities and Society, 50(October), 101684. https://doi.org/10.1016/j.scs.2019.101684
- Vardopoulos, I., & Theodoropoulou, E. (2018). Does the new‘fix’fit? adaptive building reuse affecting local sustainable development: preliminary results. Proceedings of the IAFOR Conference on Heritage & the City – New York 2018, New York(Vol. 1, pp. 997–114). IAFOR. https://d1wqtxts1xzle7.cloudfront.net/58210995/HCNY2018_43399-libre.pdf?1547834815=&response-content-disposition=inline%3B+filename%3DDoes_the_new_FIX_fit_Adaptive_building_r.pdf&Expires=1719973427&Signature=gtMp65c8Z4fB6P6Z6CJKc-cx2vZgiFmMlw~tPTqsUx-C1ptLIH2G3HJya~~rNw3v7QYFmZRWA9s-sCn-IfxSTsKLpbX~EUi~u~hBZbxZQrXaYTMDLF-45eb8~czGY6ICxhKrtiWjeTi192wXG7xHABK50pEGHtFDB8u~8PslZc-IeeSzXvHxtxNIT~oWyfo409i~xLdIBzFn2M1jUOa3nr77O8IHB1fmuwzzOvmodNVA2J6T5x25tdeO4omQNrNfDz-nSVLZ~uB76p7pVFz-cYvfy-I0cS44c6eMR6DxcYSCHmpnHiV-bExFStpMb86ZHoMi5hcezkY3WuqQ~Ib3xQ__&Key-Pair-Id=APKAJLOHF5GGSLRBV4ZA
- Webb, A. L. (2017). Energy retrofits in historic and traditional buildings: A review of problems and methods. Renewable and Sustainable Energy Reviews, 77(September), 748–759. https://doi.org/10.1016/j.rser.2017.01.145
- Wijesiri, W. M. M., Devapriya, K. A. K., Rathnasiri, P., & Wickremanayake Karunaratne, T. L. (2022). A framework to Implement green adaptive reuse for existing buildings in Sri Lanka. Intelligent Buildings International, 14(5), 581–605. https://doi.org/10.1080/17508975.2021.1906204
- Wilkinson, S., James, K., & Reed, R. (2009). Delivering Sustainability through the Adaptive Reuse of Commercial Buildings: The Melbourne CBD Challenge. Pacific Rim Real Estate Society (PPRES).
- Wong, L. (2016). Adaptive Reuse: Extending the Lives of Buildings. Birkhäuser.
- Yıldırım, M., & Turan, G. (2012). Sustainable development in historic areas: Adaptive Re-use challenges in traditional houses in sanliurfa, Turkey. Habitat International, 36(4). Elsevier: 493–503. https://doi.org/10.1016/j.habitatint.2012.05.005
- Zabrze. Raport za 2020 rok. (2021). Zabrze. Urząd Miasta w Zabrzu.
- Zakerhosseini, A., Ali Abdoli, M., Mohammadali Molayzahedi, S., & Kiani Salmi, F. (2023, August). Life cycle assessment of construction and demolition waste management: A case study of mashhad, Iran. Environment, Development and Sustainability. https://doi.org/10.1007/s10668-023-03703-1
- Zulkanain, M. I., Jaafar, M., & Aras Agus Salim, N. (2021). Exploring the adaptive reuse concept and benefits in managing public building quarters in Malaysia. Malaysian Construction Research Journal, 12(1), 258–270.