Abstract
The electrospinning technique, which was invented about 100 years ago, has attracted more attention in recent years due to its possible biomedical applications. Electrospun fibers with high surface area to volume ratio and structures mimicking extracellular matrix (ECM) have shown great potential in tissue engineering and drug delivery. In order to develop electrospun fibers for these applications, different biocompatible materials have been used to fabricate fibers with different structures and morphologies, such as single fibers with different composition and structures (blending and core-shell composite fibers) and fiber assemblies (fiber bundles, membranes and scaffolds). This review summarizes the electrospinning techniques which control the composition and structures of the nanofibrous materials. It also outlines possible applications of these fibrous materials in skin, blood vessels, nervous system and bone tissue engineering, as well as in drug delivery.
Introduction
The process of electrospinning was first patented by Cooley [Citation1] and Morton [Citation2] in 1902, and its further developments toward commercialization were made by Formhals between 1934 and 1944 [Citation3, Citation4]. An electrospinning device essentially consists of a high-voltage power supply, which creates an electric field between a grounded collector and a positively charged capillary filled with polymer solution (figure ). When a sufficiently high voltage is applied to a liquid droplet, the body of the liquid becomes charged, and when the electrostatic charge becomes larger than the surface tension of the polymer solution at the capillary tip, a polymer jet is created. As the jet dries out in flight, it is elongated by a whipping process caused by electrostatic repulsion initiated at small bends in the fiber, until it is finally deposited on the grounded collector. The elongation and thinning of the fiber leads to the formation of a uniform fiber with micro- to nanometer scale diameters, which can be collected in various orientations to create unique structures with different composition and mechanical properties [Citation5].
Electrospinning has attracted much attention not only because of its versatility in spinning a wide variety of polymeric fibers but also because of its consistency in producing fibers with submicron thickness [Citation6]. Electrospun fibers with the diameter between 50 nm and 5 μm have shown many outstanding properties, such as large surface area, high length/diameter ratio, flexible surface functionality, tunable surface morphologies and superior mechanical performance [Citation7]. Among many possible applications of electrospun fibers, such as multifunctional membranes, filter media for submicron particles in separation industry, composite reinforcement and structures for nanoelectronic machines, biomedical applications, such as preparation of scaffolds used for tissue engineering and drug delivery, have become one of the most interesting areas in electrospinning field [Citation8–10].
Tissue engineering is an interdisciplinary field that applies the principles of chemistry, physics, materials science, engineering, cell biology and medicine to the development of biological substitutes that restore, maintain or improve tissue/organ functions [Citation11]. With the ability to form nano-fibrous structures, a drive has begun to mimic the extracellular matrix (ECM) and form scaffolds that are an artificial extracellular matrix suitable for tissue formation [Citation12]. Electrospun fibrous scaffolds have a high surface-to-volume ratio which is thought to be able to enhance cell adhesion [Citation12], migration, proliferation and differentiated function [Citation13], and the porosity of electrospun mats aids in nutrient transport. However, limitations due to fabrication technology exist when using electrospun fibers to design tissue engineering scaffolds to mimic specific tissue structure. In order to combat these limitations, many researchers have been paying more and more attention to the development of new electrospinning techniques in recent years. This review summarizes all the up-to-date techniques for preparation of different structures of electrospun fibers for tissue engineering applications.
Besides tissue engineering, electrospun fibers have also been attracting significant attention lately for topical drug delivery. Localized drug therapy of skin and wound treatment, using appropriate electrospun fibers as delivery vehicles, could significantly reduce the systemic absorption of the drugs, and also provide localized therapeutic effect at lower drug concentrations. Some of the unique features of electrospun fibers as drug delivery vehicles are the ability to incorporate a wide range of drugs in an amorphous or solid solution form within the fibers, the high surface area of the fibers leading to efficient drug release, the interconnecting porous structure with high permeability, and the ease of fabricating the delivery vehicle in the required architecture/form. Currently, polymeric nanofibers for drug delivery are under extensive investigations for a variety of other biomedical applications, such as post surgical adhesion prevention membranes where surgery is already indicated [Citation14]. In this review, drug delivery using electrospun fibers is also summarized.
In concern with the recent progress on the use of electrospun scaffolds for tissue engineering and drug delivery, this review lays emphasis on introducing the development of electrospinning techniques for fabrication of electrospun materials with different architecture and morphologies. This includes the structural development from single electrospun fibers to fiber bundles, membranes and three-dimensional (3D) scaffolds which can be practically applied in tissue engineering, as well as blend-electrospinning, core-shell structure and so on. Moreover, tissue engineering scaffolds and drug carriers can be combined to achieve double functions as drug releasing scaffolds. It is not difficult to foresee that scaffolds made of electrospun fibers will have a great potential for applications in tissue engineering field.
Preparation and characterization of electrospun nanofibers and fibrous assemblies
Randomly arranged electrospun nanofibers
Electrospun materials are generally prepared from ultrafine fibers with non-woven and randomly arranged structures, and the morphology and diameter of electrospun fibers are affected by a number of parameters. These parameters include
the type of polymers, polymer concentration, viscosity, elasticity, electrical conductivity and surface tension of the solvent;
the operational conditions such as the feeding rate for the polymer solution, tip to collector distance and the strength of applied electric field;
the structure of the collectors and the design of the specific electrospinning setup; and
the humidity and temperature of the environment [Citation15].
In the aspects of polymer-electrospun fibers, Cui et al designed an orthogonal experiment system to optimize the processing parameters of the electrospinning [Citation7]. The orthogonal table L18(3)7 was used in the experiment, and the fiber diameters and beads per cent of obtained fibers were set as the investigation target. Six influential factors were chosen, including electrical voltage, solution concentration, polymer molecular weight, solvent system, flow velocity and the needle size of syringe; each factor set at three different levels. Through the range analysis, variance analysis, interaction effects between factors and regression analysis, the results showed that both polymer molecular weight and solution concentration have significant influences on fiber diameters. Significant effects on fiber morphologies were also found caused by the polymer molecular weight, solution concentration and solvent system. Therefore, different morphologies (figure ) and diameters of poly(DL-lactide) (PDLLA) electrospun fibers could be controlled by different parameters. In the orthogonal table design experiment, Cui et al further fabricated PDLLA electrospun fibers with different diameters, fibrous pore sizes and roughness (figure ) [Citation16]. They found that the surface roughness degree of electrospun mats increased with the decrease in fiber diameters and the pore sizes between fibers, leading to higher air entrapment in the surface pores which resulted in a more hydrophobic surface.
Figure 2 SEM observations of PDLLA electrospun mat containing only fibers (A), small amount of beads (B), large amount of beads (C) and beads with few fibers (D).
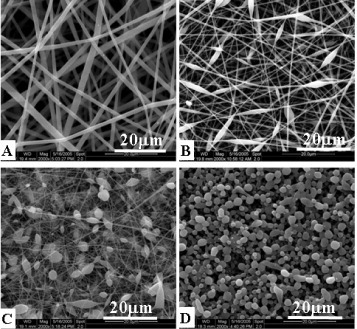
Figure 3 SEM morphologies and water contact angles images of different diameters (A), fibrous pore sizes (B) and roughness (C) PDLLA electrospun fibers.
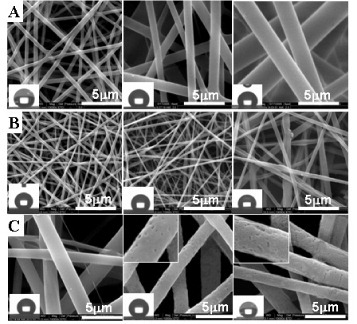
In the aspect of inorganic electrospun fibers, Chang et al investigated the effect of electrospinning parameters on the diameter and morphology of bioactive glass (BG) nanofibers [Citation17]. They found that the addition of poly(vinyl pyrrolidone) (PVP) resulted in sufficient chain entanglement and the formation of smooth BG nanofibers, and the addition of P123 led to a further decrease of the diameter with appropriate electric field strength, which held the balance between the electrostatic repulsive force and surface tension of the electrospinning solution.
Electrospun fibrous bundle
Electrospinning can yield continuous fibers with diameters ranging from nano- to micro-scale in a randomly oriented and non-woven form [Citation18]. Mechanical strength of electrospun fibers is often significantly lower than that of textile fibers made from the same polymer, and this may limit their applications in many fields. Obtaining aligned fibers with highly ordered structure is desirable for some specific applications such as suture development. Therefore, the attempt has been made to fabric ultrafine electrospun fibers with bundled structure which can significantly improve the mechanical strength. Wang et al fabricated macroscopically continuous, aligned electrospun fiber yarns with a higher value of tensile strength using a self-bundling electrospinning method [Citation19]. Bosworth et al reported a way to prepare uniaxially aligned fiber bundles by collecting fibers in a liquid reservoir, and scaffolds mimicking the hierarchical tendon structure could be fabricated using these bundles for tendon regeneration [Citation20]. He et al obtained relatively long and aligned fiber bundles for suture application by using a rotating round disc with high rotation speed as the collector [Citation21].
Electrospun fibrous membranes
Electrospun fibrous membranes are widely used for tissue engineering applications. These membranes are generally prepared using flat collecting plates and thus have a randomly distributed fibrous structure [Citation22, Citation23]. Native ECMs in different tissues or organs have specifically defined architectures which play important role in determine tissue functions. Previous studies have shown that specific topological architectures of the materials mimicking ECMs could promote favorable biological responses, such as enhanced protein adsorption, as well as enhanced cell adhesion, proliferation and migration [Citation24]. Therefore, efforts have been made to develop electrospun materials with ordered microstructures and patterns [Citation22].
To make membranes with aligned nanofibers or simple patterns, various electrospinning setups have been applied, such as rotating disk collectors, parallel electrodes, or rotating wire drum collectors. Matthews et al [Citation25] demonstrated the effect of the rotating speed of a mandrel on the alignment degree of electrospun collagen fiber. At a speed below 500 rpm, a random mix of collagen fibers was collected. However, when the rotating speed of the mandrel was increased to 4500 rpm (approximately 1.4 m s−1 at the surface of the mandrel), the collagen fibers showed significant alignment along the axis of rotation. Theron et al [Citation26] described an electrostatic field-assisted assembly technique using a tapered and grounded wheel-like bobbin to position and align individual nanofibers into parallel arrays. Katta et al [Citation27] employed a macroscopic copper wire framed rotating drum as the collector, and the electrospun fibers, collected on the drum as it rotated, were parallel to each other. Li et al [Citation28, Citation29] fabricated parallel arrays made of polymeric and ceramic nanofibers using a collector consisting of two pieces of electrically conductive substrate separated by a gap. Yang et al fabricated well-aligned arrays and multilayer grids by a magnetic electrospinning technique, where magnetized fibers are stretched into essentially parallel fibers over large areas (more than 5×5 cm2) in a magnetic field [Citation30].
Considering the delicate structure and complexity of tissue, it is challenging to fabricate fibrous membrane materials with accurately controlled microstructures or patterns. The architecture of an electrospun membrane is generally composed of two parts, the macroscopic organization of the nanofibers and the secondary structure of the individual fiber arrangement. Zhu et al demonstrated fabrication of electrospun fibrous mats with mesoscopically ordered structure using a slowly rotating frame cylinder, and different rotation speeds were applied to acquire electrospun poly(DL-lactide-glycolide) (PLGA) fibrous mats with varied porosities [Citation31]. More complicated architectures and patterns, as shown in figure , can be obtained by designing electrically conductive templates with different microstructure [Citation32]. The protrusions on the template and the diameter of the conductive wires in the collectors are key parameters; they can greatly affect the structures of the electrospun mats, and woven structures can be generated by a timely controlled arrangement of the conductive protrusions in the collector.
Electrospun fibrous scaffolds
Non-woven electrospun nanofibers membranes are architecturally similar to the structure of the ECM, and the loose bonding between fibers is beneficial for tissue ingrowth and cell migration, promoting good nutrition distribution throughout whole fibrous scaffold [Citation33]. However, many tissue engineering applications require specific 3D assemblies of fibrous materials. For example, electrospun fibrous tubes are of practical importance in vascular, neural and tendinous tissue engineering [Citation34].
A fibrous scaffold conduit tube can be fabricated by electrospinning over a rotating rod. Stitzel et al fabricated a 12 cm long fibrous conduit by electrospinning a polymer solution containing a mixture of collagen type I, elastin and PLGA on a circular mandrel of 4.75 mm diameter for vascular grafting. Compliance tests showed that the conduit had a diameter change of 12–14% within the physiologic pressure range, which is close to that of the native vessels [Citation35]. Moreover, considering the three-layer structure of the native artery (the intima, media and adventitia), Boland et al fabricated a biomimicking vascular graft with a three-layered construction. A solution of collagen type I and elastin (80/20 w/w) at a concentration of 0.083 g ml−1 in 1, 1, 1, 3, 3, 3-hexafluoro-2-propanol (HFP) was electrospun onto a tubular mandrel with 4 mm internal diameter (ID), and subsequently seeded with both fibroblasts and smooth muscle cells. Another tubular scaffold, this time 2 mm ID, was created with collagen type I and elastin solution (30/70 w/w) and inserted into the 4 mm ID scaffold. The lumen was seeded with smooth muscle cells and cultured for 3 days. Then, human umbilical vascular endothelial cells were injected into the lumen, and the construct was cultured for two more days to form a tissue-engineered vascular construct [Citation36]. Apart from the vascular grafts, electrospun tubes made of biodegradable polymers have also been used to regenerate nerve defects. Panseri et al fabricated an electrospun tube by deposition of PLGA/PCL (PCL, poly(ε-caprolactone) micro- and nanofibers on a 16-gauge copper wire 1.29 mm in diameter held near the grounded target, and used it to repair a 10-mm nerve gap in a rat sciatic nerve in vivo [Citation37].
Considering the variation in anatomic location and biological environment of tissue defects, it is also important to design and control microscopic and macroscopic 3D structures of tissue engineering tubes [Citation38]. Zhang and Chang developed a novel static method to fabricate 3D electrospun fibrous tubes with multiple micropatterns. In addition, multiple interconnected tubes, and many tubes with the same or different sizes, shapes, structures and patterns can be prepared synchronously (figure ) [Citation39]. This technique provides the possibility to design nanofibrous tubes with bimimetic structures for various tissue engineering applications.
Electrospun fibrous of composite scaffolds
The design and fabrication of scaffolds using appropriate biomaterials is a key but limiting step for the creation of functional engineered tissues and their clinical applications. Naturally occurring materials, such as collagen, elastin, silk protein, fibrinogen, chitosan, dextran, hyaluronic acid and their combinations, have been electrospun into nanofibers [Citation40, Citation41]. Although these fibrous scaffolds apparently mimic the molecular and structural properties of the native ECMs, there are still limitations preventing them from being applied in tissue engineering, such as poor mechanical properties of these natural materials and loss of their 3D structure in aqueous environment. This problem can be overcome by physically blending the synthetic and naturally occurring materials, which may result in composite materials with good biocompatibility and mechanical strength, thus combining the advantages of two types of materials. Moreover, the existence of polymers of natural origin on the surface and inside the composite nanofibers provides sustained cell recognition signals with polymer degradation, which is important for cell function development.
Cui et al found that electrospun PDLLA fibers were hydrophobic, and the surface erosion and dimensional shrinkage of the materials made it unfavorable to trigger the initial adhesion and further growth and population of the cells [Citation16]. Therefore, they fabricated PDLLA and poly(ethylene glycol) (PEG) composite electrospun fibers by blending different amount of PEG into PDLLA [Citation42]. The surface hydrophilicity was improved, and the degradation patterns of PDLLA/PEG mats changed from surface erosion to bulk degradation with the increase of the PEG contents in the composite. The dimensional shrinkage was alleviated through the formation of crystal regions of PEG in the fiber matrix. Human dermal fibroblasts (HDFs) interacted and integrated well with the surrounding fibers containing 30% PEG, which provided better environment for growth and metabolism of HDFs than pure PDLLA electrospun mats. Zhou et al successfully prepared electrospun spider silk fibroins/PDLLA composite fibrous non-woven mats (figure ) [Citation43]. This study demonstrated that the superhydrophobic property of PDLLA fibers could be turned into the superhydrophilic property by introducing spider silk fibroins, and the mechanical properties of PDLLA were also improved by blending with spider silk fibroins. The composite fibers showed excellent biocompatibility according to the cell cytotoxicity test. Yang et al prepared electrospun composite mats of PLGA and collagen with high porosities of 85–90% and extended pore sizes of 90–130 mm to mimic the ECM morphologically and chemically [Citation44]. The mechanical test for the blended fibrous mats indicated that they were sufficiently durable for dermal tissue engineering. The blending of collagen with PLGA enhanced the cell attachment and proliferation and extracellular matrix secretion, which were found to be dependent on the amount of collagen in the composite scaffold.
Figure 6 SEM (A) and TEM (B) micrograph of fibers electrospun from the spider silk/PDLLA emulsion with spider silk ratios of 30%.
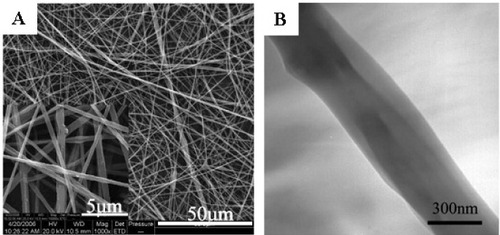
Optimal electrospun nanofibers composites should be exploited for more functional properties and high biocompatibility. The electrospun fibrous composites could be fabricated through blending polymer with nanoparticles or immobilization technologies to functionalize the electrospun nanofibers. For example, Wutticharoenmongkol et al [Citation45] prepared electrospun PCL/hydroxyapatite (HA) and PCL/CaCO3 composites using PCL solution containing nanoparticles of HA or calcium carbonate. Chang et al fabricated BG nanofibers by sol–gel electrospinning technology. These fibers had excellent bioactivity (figure (A)) and cell compatibility and are regarded as a promising next-generation biomaterial in the bone-regeneration field [Citation17]. A novel Poly(butylenes succinate) (PBSU)/wollastonite/apatite composite scaffold was also fabricated via electrospinning and biomimetic process by Zhang et al [Citation46]. After the biomimetic process, fibrous structure was formed with microspheres composed of worm-like apatite on composite fibers (figure (B)). These electrospun fibrous composites could also be fabricated with controllable microstructure and alternative materials which are applicable for bone tissue engineering.
Electrospun fibers with core-shell structure
Core-shell electrospun fibers have received great interest because of their potential in drug delivery applications. Using a coaxial dual-capillary spinneret, one component of the fiber can easily be encapsulated inside another component leading to what is known as a core-shell structured fiber. Apart from drugs, some biologically active molecules, such as enzymes and growth factors, can be shielded by the shell for specific delivery purpose [Citation47]. The shell could control the release kinetics and protect the core material as well.
A coaxial dual-capillary spinneret has been employed in electrospinning to fabricate core-shell nanofibers, which allows for the simultaneous coaxial electrospinning of two different polymer solutions [Citation48, Citation49]. Zhang et al investigated the feasibility of encapsulating a non-electrospinnable model protein along with a water-soluble polymer, PEG, into biodegradable PCL nanofibers via the coaxial electrospinning technique [Citation50]. The core-shell structured nanofibers could suppress the burst release because the drug was mixed into the inner fiber, thus its releasing rate was highly dependent on the degradation rate of the outer fiber polymer. However, it was also noted that the equipment design and electrohydrodynamic behaviors of the coaxial electrospinning were complex, and that the selection of the solvent for the inner solution and the control of the spinning parameters had a dramatic influence on the structure of the fibers, especially when a water-soluble polymer was incorporated in the inner core [Citation48]. Coating and vapor-phase deposition can also be used to fabricate core-shell fibers by immersing the inner fiber in coating materials. Selective removal of the core fiber by soaking in specific solvents or by thermal decomposition yields hollow fibers [Citation51]. Emulsion electrospinning is a novel process which prepares core-sheath fibers through stabile polymer emulsion. Xu et al have successfully encapsulated poly(ethylene oxide) (PEO) and doxorubicin hydrochloride in the shell material of poly(ethylene glycol)- poly(L-lactic acid) (PELA) [Citation52, Citation53]. Yang et al aimed at assessing the potential use of emulsion electrospinning to prepare core-shell structured ultrafine fibers as carriers for therapeutic proteins. In the case of bovine serum albumin (BSA) which was selected as a model protein, PDLLA ultrafine fibers, prepared by emulsion electrospinning using a lower volume ratio of aqueous to organic phase, showed higher structural integrity of core-shell fiber as assessed by laser confocal scanning microscopy (LCSM) (figure (A)) [Citation47]. Yang et al further investigated the structural integrity, bioactivity and release patterns of lysozyme, as a model protein, encapsulated within the core-shell structured ultrafine fibers prepared by emulsion electrospinning [Citation48]. Electron microscopy and LCSM images demonstrated that the fibrous mats were very porous with integrally core-shell structured, bead-free and randomly arranged fibers (figure (B)).
Figure 8 SEM images (A1) and LCSM images (A2) of PDLLA electrospun core-shell fiber containing 5% BSA; SEM images (A1) and LCSM images (A2) of PDLLA electrospun core-shell fiber containing 5% lysozyme.
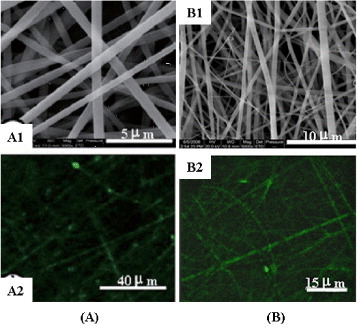
Of special interest for applications in tissue engineering are polymer nanofibers loaded with functional materials, such as inorganic, nature polymer, or other biocompatible and bio-inducement materials aiming at specific functional characteristics. Cui et al prepared PDLLA electrospun fibers with calcium nitrate entrapment and then incubated in phosphate solution to form in situ calcium phosphate on the polymer fibers matrix, and core-shell electrospun fibrous composite could be formed with a polymer core and a inorganic shell [Citation54]. The formation of calcium phosphate and good dispersion of calcium phosphate particles on the electrospun fibers were observed. The resulting nanocomposites may have potential applications as scaffolds for tissue engineering, or as fillers for preparation of fiber-enforced composites for other biomedical applications. Cui et al further grafted free amino groups on the PDLLA electrospun fibers through an optimized amino lysis process, and gelatin was further grafted on the aminolyzed fibers through glutaraldehyde coupling [Citation55]. The core-shell composites of HA and PDLLA could be further fabricated by incubating PDLLA-gelatin fibers in simulated body fluid (SBF), in which gelatin was functioning as the active site for HA nucleation and growth. Li et al also obtained electrospun core-shell fibrous composites of calcium phosphate and polymer by coating the polymer fibers with gelatin through layer-by-layer self-assembly, followed by functionalization with a uniform coating of bonelike calcium phosphate on the electrospun fibers surface by mineralization in the concentrated SBF for 2 h [Citation56, Citation57]. These core-shell system containing PCL, gelatin and calcium phosphate could serve as a new class of biomimetic scaffolds for bone tissue engineering applications.
Application of electrospun nanofibrous materials in tissue engineering and drug delivery
Application of electrospun fibrous materials in tissue engineering
Scaffold architectures and materials of tissue engineering are both important aspects for cell adhesion, migration, proliferation and differentiation. Among the various types of scaffolds in tissue engineering, electrospun fibrous scaffolds offer great advantages because they, to certain extent, mimic the structure of ECM as well the possibility of the wide range of chemicals that can be chosen from to meet different application requirements. Also, the simplicity of this technique for preparation of tissue engineering scaffolds is a plus. Many investigators have made contributions to fabricate various electrospun fibrous scaffolds for engineering human tissues such as skin, bone, blood vessel, nerve, cartilage and so on.
Skin trauma is one of the major problems in surgical field. An ideal skin tissue engineering scaffold should possess moderately hydrophilic surface, dimensional un-shrinkage, suitable microstructure, porosity, controllable biodegradability, suitable mechanical property and should be improving the initial adhesion and further growth and population of cells. To serve these purposes, different starting materials of the electrospinning have been explored, such as synthetic biodegradable polymers (PCL, PDLLA, PGA, PLGA and so on) and natural polymers (collagen, gelatin, chitosan, fibrin and so on) [Citation58, Citation59]. Cui et al found that the electrospun mats containing 30% PEG and 70% PDLLA exhibited the most balanced properties, including moderate hydrophilicity, minimal dimensional changes, adaptable bulk biodegradation pattern and enhancement of cell penetration and growth within fibrous mats (figure (A)) compared to other combinations [Citation42]. Yang et al also found that electrospun composite mats of PLGA mixed with collagen enhanced the cell attachment, proliferation and ECM secretion (figure (C)) [Citation44]. Meanwhile, high porosity and larger pore sizes of the mats allowed cells to migrate into the fibrous scaffolds and improved their proliferation [Citation44]. Zhu et al fabricated highly porous PLGA electrospun fibrous scaffolds and observed higher human dermal fibroblast viability, collagen deposition and cell migration on the scaffold (figure (B)) [Citation31].
Figure 9 SEM images of human dermal fibroblasts growth on the PDLLA/PEG fibrous mats containing 30% PEG (A), larger pore sizes and higher porosity (B), and CLSM images of cell-grown PLGA/collagen fibrous mats containing 30% collagen.
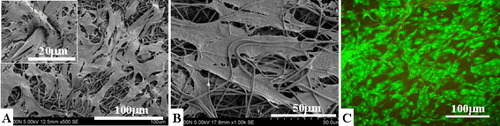
Blood vessels not only transport blood to and from the heart, they are also important places for the exchange of water and other chemicals between blood and the tissues. Presently, many researchers have successfully created, by electrospinning, various geometries and sizes of blood vessels with seamless, non-woven fibrous structures using natural and synthetic biodegradable polymers. A “mechano-active” small-diameter artificial vascular graft made of elastomeric poly(L-lactide-co-ecaprolactone) (PLCL) was designed by Inoguchi et al using electrospinning technique. The wall thicknesses of those tubular scaffolds were adjustable [Citation60]. Tillman et al evaluated the in vivo stability of the electrospun PCL-collagen scaffolds in vascular reconstruction in a rabbit aortoiliac bypass model [Citation61]. They found that the vascular scaffolds could maintain enough mechanical strength for cell growth and other physiologic conditions. Numerous studies suggest that tissue engineering scaffold, combined with both electrospun scaffolds and vascular cells, may become an alternative to prosthetic vascular grafts for vascular reconstruction. However, having the scaffold materials compatible to the cells is yet challenging. Stankus et al fabricated small-diameter conduits by electrospinning of a biodegradable, elastomeric poly(ester urethane) urea (PEUU). During that process, they concurrently electrosprayed smooth muscle cells to achieve cellular infiltration within the electrospun matrix [Citation62]. Other studies have demonstrated that the cells actually migrate and proliferate along the direction of the electrospun fiber alignment [Citation63, Citation64]. In another word, aligned fibers are favorable to the growth of the cells attached on them. Xu et al developed an aligned nanofibrous membrane by electrospinning of a PLCL (75:25) copolymer [Citation64]. They found that the adhesion and proliferation of the coronary artery smooth muscle cells were improved as compared to the plane polymer films, and cells were able to migrate along the axis of the aligned fibers. Furthermore, the proteins comprising the cytoskeleton of the smooth muscle cells were aligned parallel to the aligned fibers, demonstrating the cells proclivity to organize along oriented fibrous topography. These results suggested that a nano-fibrous membrane, containing aligned fibers with appropriate diameter and distance apart, is favorable in oriented cell growth.
The nervous system coordinates the actions of an animal and transmits signals between different parts of the body. However, the regeneration capability of the nervous system is quite limited. When the nerve stumps cannot be directly connected by surgery without producing tension, an artificial nerve conduit seems to be the only choice to bridge the gap without creating new injuries in the body [Citation65, Citation66]. Kim et al fabricated polyacrylonitrile-methacrylate (PAN-MA) thin fibrous scaffolds as guidance channels for nerve regeneration. These scaffolds could promote Schwann cell migration and reconnect critically sized nerve gaps without the aid of any exogenous ECM or trophic proteins [Citation67]. Nisbet et al implanted PCL scaffolds into the caudate putamen of the adult rat brain to investigate the extent of microglial and astrocytic response. They found that the implanted scaffolds were not encapsulated by a scar after 60 days of implantation. More interesting, they demonstrated that neuritis penetrated into the randomly orientated electrospun scaffolds, but could not penetrate the partially aligned scaffolds, suggesting the importance of the fiber orientation [Citation68]. In the peripheral nerve regeneration process, aligned nanofibrous scaffolds have the ability to direct axonal outgrowth and glial cell migration [Citation69], which is consistent with the observations in smooth muscle cells mentioned previously [Citation64]. Gerardo-Nava et al investigated the interaction between human neural cell and orientated electrospun nanofibers in vitro. They found that the orientation of the electrospun fibers had a great impact on the astrocytes growth rate, morphology and alignment. And fiber surface functionalized by collagen significantly improved neural cell adhesion and migration. In their paper, they proposed that the specific functionalization of nanofibers and controlled incorporation of such nanofibers into a 3D scaffold are both significant challenges in supporting axonal regeneration [Citation70].
The bone matrix is mineralized by calcium phosphate in the form of crystals, which is very similar to hydroxyapatite (HA). The current challenge in bone tissue engineering is to fabricate a bioactive bone graft which mimics the ECM with effective bone mineralization. Prabhakaran et al fabricated biocomposite polymeric nanofibers containing nano HA by electrospinning for bone tissue engineering. They suggested that the biocomposite PLLA/collagen/HA nanofibrous scaffold could improve the proliferation and mineralization of osteoblasts, resulting in the enhancement of bone regeneration [Citation71]. Recently, Wang et al successfully fabricated electrospun fibrous scaffold with well-tailored architectures and patterns from PCL using a stainless steel mesh as the template collector [Citation72]. The patterned electrospun PCL scaffold showed a much higher cell proliferation rate as compared with the PCL scaffold with random fibrous structure in a mouse osteoblastic cell MC3T3-E1. Moreover, the cells grew and elongated along the electrospun fiber axial orientation. The results suggested that the electrospun nanofiber scaffolds with tailored architectures and patterns have great potential for engineering functional tissues or organs, where an ordered of directional cellular organization is essential.
Application of electrospun fibers in drug delivery
Many therapeutic compounds can be conveniently incorporated into the electrospun polymers fibers through electrospinning process, which was unlike common encapsulation methods involving some complicated preparation process. At present, both degradable and non-degradable polymers are under investigation to be developed as drug carriers for local delivery of antibiotics and anticancer drugs, and electrospun fibrous materials with different structure are preferred selections [Citation73]. The advantages of the electrospinning technique are in maintaining the molecular structure and bioactivity of the incorporated drugs or bioactive molecules due to the mild process conditions, and in reducing the burst release of drugs in vitro. Ignatious et al defined that the release of pharmaceutical dosage from nanofibers can be designed as rapid, immediate, delayed or modified dissolution depending on the polymer carrier used [Citation74].
To design the proper release system, two electrospinning methods can be applied. One is the preparation of drug carrying fibers by blending drugs into the polymer materials. In this method, both drugs and polymers are mixed together and electrospun into drug-loaded ultrafine fibers. In this way, drugs could easily locate on the fiber surface because of the rapid evaporation of the solvent and high ionic strength in solution. Therefore, fibrous mats prepared by blending method will most probably result in a burst effect in the initial stage of drug release [Citation75, Citation76], where initial burst release is necessary to eliminate the intruding bacteria before they begin to proliferate in the case of antibiotics [Citation73]. For example, bio-absorbable nanofibers of PLA were used to load an antibiotic drug Mefoxin, and the efficiency of this nanofibrous membrane was superior to that of bulk film [Citation77]. Cui et al investigated the effect of fiber diameter and drug loading on the release behavior in an electrospun polymer drug delivery system prepared using blending method, and they found that electrospun fibers with larger diameter exhibited longer period of nearly zero order release, and higher drug encapsulation resulted in a more significant burst release [Citation78]. For potential use in topical drug administration, Brewster et al investigated poorly water-soluble drugs loaded in a water-insoluble, non-biodegradable polymer [Citation79]. The collected non-woven fabrics were shown to release the drugs at various rates and profiles based on the nanofiber morphology and drug content. Cui et al synthesized acid-labile polymers by introducing acetal groups and ortho ester groups into biodegradable backbone of PELA, respectively, and investigated the degradation and drug release behaviors responding to the local pathological pH environment [Citation80, Citation81]. In vitro release study showed that the total amount of drug released from acid-labile polymeric fibers was accelerated due to the pH-induced structural and morphological changes of fibrous mats and the degradation of matrix polymers, and the burst release was significantly higher for polymers with higher contents of acid-labile segments.
The second method to prepare fibrous drug delivery system is the fabrication of electrospun fibers with core-shell structures, in which two miscible or immiscible components can be conveniently electrospun into a composite fiber with a core layer encapsulated inside a shell. In this way, drugs or their mixture can be enclosed within the polymeric outer shell to form a core-shell type drug delivery device [Citation51, Citation82]. The core-shell electrospinning technique opens a new chapter to expand applications of electrospinning. Bioactive agents or drugs encapsulated into electrospun nanofibers show steady release characteristics. A model drug tetracycline hydrochloride (TCH) encapsulated into PLLA fibers have been used to fabricated core-shell fibers through coaxial electrospinning [Citation21]. In vitro release study indicated that threads made from the core-shell fibers could suppress the initial burst release and provide a sustained drug release useful for administering growth factor or other therapeutic drugs. Yang et al obtained core-shell structured ultrafine fibers of BSA/PDLLA through emulsion electrospinning [Citation45]. They found that emulsion electrospinning can provide a useful core-shell structure, which may serve as a promising scaffold for sustainable, controllable, and effective release of bioactive proteins for tissue engineering and other applications.
In summary, by controlling the mode of encapsulation and the architecture of electrospun fibers, drug-carriers can be prepared to achieve appropriate drug release patterns. Therefore, electrospun fibers have great potentials in a variety of therapeutic applications based on the present stage of development.
Electrospun fibers as drug releasing tissue engineering scaffolds
Tissue engineering and drug delivery are closely related areas in biomedical field, and the release of the therapeutic factors may enhance the efficacy of tissue engineering scaffolds. Goldberg et al reported various nano-structured materials for drug delivery combined with tissue engineering applications [Citation83].
Chew et al reported that the human β-nerve growth factor (NGF) was stabilized in electrospun copolymer of ε-caprolactone and ethyl ethylene phosphate (PCLEEP) with BSA as a carrier protein [Citation84]. NGF could sustain release for three months from the matrix, and PC12 neurite outgrowth assay confirmed that the bioactivity of electrospun NGF was retained throughout the period of sustained release. Nie and Wang [Citation85] have constructed an electrospun PLGA/HA composite scaffold for delivery of plasmid DNA. Cell culture experiments with human marrow stem cells (hMSCs) show that the scaffolds with encapsulated DNA/chitosan nanoparticles resulted in higher cell attachment, higher cell viability than the pure polymer scaffolds, and the composite scaffolds revealed desirable transfection efficiency of DNA. Nie et al further fabricated composite fibrous scaffold of PDLLA/HA containing bone morphogenetic protein-2 (rhBMP-2), and they found that BMP-2 release rate could be controlled by the amount of HA [Citation86]. Bolgen et al fabricated PCL electrospun fibers containing antibiotic of Biteral, which was used to prevent post surgery induced abdominal adhesions. They found that PCL electrospun fibers containing antibiotic could reduce extent, type and tenacity of adhesion of the materials after implanting on the abdominal wall of rats [Citation87]. Casper et al reported that the electrospun fibers, made from PEG functionalized with low molecular weight heparin, had displayed potentials for drug delivery and wound repair applications. They also proposed that the nanomaterials made by electrospinning may be ideal for growth factor localization [Citation88]. All these studies demonstrated the feasibility and advantages of nanofibrous materials for controlled drug delivery and tissue engineering applications by defining the appropriate combination of cells, materials, drugs or growth factors presented in the electrospun fibers.
Opportunities and challenges
Electrospinning technology can be used to fabricate fibrous materials with various chemical compositions, macro- and micro-structures and morphologies, which have been used for tissue engineering and drug delivery applications. The advantages of the electrospun materials for tissue engineering and drug delivery applications are that the nanofibrous structure mimics, to certain extend, the ECM of the living cells, and allows easy incorporation of drugs by blending or core-shell encapsulation into nanofibrous structures. However, challenges using electrospun fibers are generally associated with defining the appropriate cell populations and controlling the effects of matrix composition and signaling on the cells of interest. One of the key factors is to accurately control the fiber deposition, probably in nanometer scale, in order to achieve a more precise mimicking of ECM. Therefore, a further understanding is critical of the mechanisms and key parameters that determine the nanofiber formation and deposition. It will accelerate the development of electrospinning techniques and setups, and electrospun materials will then find more potential applications in the field of tissue engineering and drug delivery. In addition, it is also critical to further understand the cellular reaction to electrospun materials with different composition, fiber orientation and microstructures. Currently, although numerous studies have demonstrated the great potential of electrospun fibrous materials, fewer in vivo studies have been carried out. For drug delivery applications, more sophisticated electrospinning techniques need to be further developed according to the requirements of biomedical applications. The joint efforts from both material scientists and biologists are needed for more interdisciplinary research on the development of electrospun techniques.
Acknowledgment
This work was supported by the National Basic Science Research Program of China (973 Program) (Grant 2005CB522704) and the Natural Science Foundation of China (Grant 30730034).
References
- CooleyJ 1902 US Patent No. 692,31
- MortonW 1902 US Patent No. 705,691
- FormhalsA 1934 US Patent No. 1,975,504
- FormhalsA 1939 US Patent No. 2,349,950
- BarnesCSellSBolandESimpsonDBowlinG 2007 Adv. Drug Deliv. Rev. 59 1413 http://dx.doi.org/10.1016/j.addr.2007.04.022
- SubbiahTBhatGTockRParameswaranSRamkumarS 2005 J. Appl. Polym. Sci. 96 557 http://dx.doi.org/10.1002/app.21481
- CuiWLiXZhouSWengJ 2007 J. Appl. Polym. Sci. 103 3105 http://dx.doi.org/10.1002/app.25464
- LiMMondrinosMGandhiMKoFWeissALelkesP 2005 Biomaterials 26 5999 http://dx.doi.org/10.1016/j.biomaterials.2005.03.030
- FrenotAChronakisI 2003 Curr. Opin. Colloid Interface Sci. 8 64
- ZongXLiSChenEGarlickBKimKFangDChiuJZimmermanTBrathwaiteCHsiaoB 2004 Ann. Surg. 240 910 http://dx.doi.org/10.1097/01.sla.0000143302.48223.7e
- LangerRVacantiJ 1993 Science 260 920 http://dx.doi.org/10.1126/science.8493529
- FlemmingRMurphyCAbramsGGoodmanSNealeyP 1999 Biomaterials 20 573 http://dx.doi.org/10.1016/S0142-9612(98)00209-9
- KimTParkT 2006 Tissue Eng. 12 221 http://dx.doi.org/10.1089/ten.2006.12.221
- MaZKotakiMInaiRRamakrishnaS 2005 Tissue Eng. 11 101 http://dx.doi.org/10.1089/ten.2005.11.101
- LiDXiaY 2004 Adv. Mater. 16 1151 http://dx.doi.org/10.1002/adma.200400719
- CuiWLiXZhouSWengJ 2008 Polym. Degrad. Stab. 93 731 http://dx.doi.org/10.1016/j.polymdegradstab.2007.12.002
- XiaWZhangDChangJ 2007 Nanotechnology 18 135601 http://dx.doi.org/10.1088/0957-4484/18/13/135601
- HuangZHeCYangAZhangYHanXYinJWuQ 2006 J. Biomed. Mater. Res. A 77 169
- WangXZhangKZhuMHsiaoBChuB 2008 Macromol. Rapid Commun. 29 826 http://dx.doi.org/10.1002/marc.200700873
- BosworthLCleggPDownesS 2008 Int. J. Nano Biomater. 1 263 http://dx.doi.org/10.1504/IJNBM.2008.016875
- HeCHuangZHanX 2009 J. Biomed. Mater. Res. A 89 80
- TeoWRamakrishnaS 2006 Nanotechnology 17 R89 http://dx.doi.org/10.1088/0957-4484/17/14/R01
- HuangZZhangYKotakiMRamakrishnaS 2003 Compos. Sci. Technol. 63 2223 http://dx.doi.org/10.1016/S0266-3538(03)00178-7
- LiWTuliROkaforCDerfoulADanielsonKHallDTuanR 2005 Biomaterials 26 599 http://dx.doi.org/10.1016/j.biomaterials.2004.03.005
- MatthewsJWnekGSimpsonDBowlinG 2002 Biomacromolecules 3 232 http://dx.doi.org/10.1021/bm015533u
- TheronAZussmanEYarinA 2001 Nanotechnology 12 384 http://dx.doi.org/10.1088/0957-4484/12/3/329
- KattaPAlessandroMRamsierRChaseG 2004 Nano Lett. 4 2215 http://dx.doi.org/10.1021/nl0486158
- LiDWangYXiaY 2004 Adv. Mater. 16 361 http://dx.doi.org/10.1002/adma.200306226
- LiDWangYXiaY 2003 Nano Lett. 3 1167 http://dx.doi.org/10.1021/nl0344256
- YangDLuBZhaoYJiangX 2007 Adv. Mater. 19 3702 http://dx.doi.org/10.1002/adma.200700171
- ZhuXCuiWLiXJinY 2008 Biomacromolecules 9 1795 http://dx.doi.org/10.1021/bm800476u
- ZhangDChangJ 2007 Adv. Mater. 19 3664 http://dx.doi.org/10.1002/adma.200700896
- Heydarkhan-HagvallSSchenke-LaylandKDhanasoponARofailFSmithHWuBSheminRBeyguiRMacLellanW 2008 Biomaterials 29 2907 http://dx.doi.org/10.1016/j.biomaterials.2008.03.034
- SroujiSKizhnerTSuss-TobiELivneEZussmanE 2008 J. Mater. Sci. Mater. Med. 19 1249 http://dx.doi.org/10.1007/s10856-007-3218-z
- StitzelJLiuJLeeSKomuraMBerryJSokerSLimGVan DykeMCzerwRYooJ 2006 Biomaterials 27 1088 http://dx.doi.org/10.1016/j.biomaterials.2005.07.048
- BolandEMatthewsJPawlowskiKSimpsonDWnekGBowlinG 2004 Front. Biosci. 9 1422 http://dx.doi.org/10.2741/1313
- PanseriSCunhaCLoweryJDel CarroUTaraballiFAmadioSVescoviAGelainF 2008 BMC Biotechnol. 8 39 http://dx.doi.org/10.1186/1472-6750-8-39
- GadegaardNDalbyMRiehleMCurtisAAffrossmanS 2004 Adv. Mater. 16 1857 http://dx.doi.org/10.1002/adma.200400408
- ZhangDChangJ 2008 Nano Lett. 8 3283 http://dx.doi.org/10.1021/nl801667s
- JiangHFangDHsiaoBChuBChenW 2004 Biomacromolecules 5 326 http://dx.doi.org/10.1021/bm034345w
- UmIFangDHsiaoBOkamotoAChuB 2004 Biomacromolecules 5 1428 http://dx.doi.org/10.1021/bm034539b
- CuiWZhuXYangYLiXJinY 2009 Mater. Sci. Eng. C 29 1869 http://dx.doi.org/10.1016/j.msec.2009.02.013
- ZhouSPengHYuXZhengXCuiWZhangZLiXWangJWengJJiaW 2008 J. Phys. Chem. B 112 11209 http://dx.doi.org/10.1021/jp800913k
- YangYZhuXCuiWLiXJinY 2009 Macromol. Mater. Eng. 294 611 http://dx.doi.org/10.1002/mame.200900052
- WutticharoenmongkolPSanchavanakitNPavasantPSupapholP 2006 Macromol. Biosci. 6 70 http://dx.doi.org/10.1002/mabi.200500150
- ZhangDChangJZengY 2008 J. Mater. Sci. Mater. Med. 19 443 http://dx.doi.org/10.1007/s10856-006-0043-8
- YangYLiXCuiWZhouSTanRWangC 2008 J. Biomed. Mater. Res. A 86 374
- YangYLiXQiMZhouSWengJ 2008 Eur. J. Pharm. Biopharm. 69 106 http://dx.doi.org/10.1016/j.ejpb.2007.10.016
- JiangHHuYZhaoPLiYZhuK 2006 J. Biomed. Mater. Res. B 79 50
- ZhangYWangXFengYLiJLimCRamakrishnaS 2006 Biomacromolecules 7 1049 http://dx.doi.org/10.1021/bm050743i
- BognitzkiMHouHIshaqueMFreseTHellwigMSchwarteCSchaperAWendorffJGreinerA 2000 Adv. Mater. 12 637 http://dx.doi.org/10.1002/(SICI)1521-4095(200005)12:9<637::AID-ADMA637>3.0.CO;2-W
- XuXZhuangXChenXWangXYangLJingX 2006 Macromol. Rapid Commun. 27 1637 http://dx.doi.org/10.1002/marc.200600384
- XuXYangLWangXChenXLiangQZengJJingX 2005 J. Control. Release 108 33 http://dx.doi.org/10.1016/j.jconrel.2005.07.021
- CuiWLiXZhouSWengJ 2007 J. Biomed. Mater. Res. A 82 831
- CuiWLiXChenJZhouSWengJ 2008 Cryst. Growth Des. 8 4576 http://dx.doi.org/10.1021/cg800641s
- LiXXieJYuanXXiaY 2008 Langmuir 24 14145 http://dx.doi.org/10.1021/la802984a
- LiXXieJLipnerJYuanXThomopoulosSXiaY 2009 Nano Lett. 9 2763 http://dx.doi.org/10.1021/nl901582f
- HuangSFuX B 2010 J. Control. Release 142 149 http://dx.doi.org/10.1016/j.jconrel.2009.10.018
- SunTMaiSNortonDHaycockJ WRyanA JMacneilS 2005 Tissue Eng. 11 7
- InoguchiHKwonI KInoueETakamizawaKMaeharaYMatsudaT 2006 Biomaterials 27 1470 http://dx.doi.org/10.1016/j.biomaterials.2005.08.029
- TillmanB WYazdaniS KLeeS JGearyR LAtalaG AYooJ J 2009 Biomaterials 30 583 http://dx.doi.org/10.1016/j.biomaterials.2008.10.006
- StankusJ JSolettiLFujimotoKHongYVorpD AWagnerW R 2007 Biomaterials 28 2738 http://dx.doi.org/10.1016/j.biomaterials.2007.02.012
- LeeCShinHChoIKangYKimIParkKShinJ 2005 Biomaterials 26 1261 http://dx.doi.org/10.1016/j.biomaterials.2004.04.037
- XuCInaiRKotakiMRamakrishnaS 2004 Biomaterials 25 877 http://dx.doi.org/10.1016/S0142-9612(03)00593-3
- ChengH WJiangTBrownS APasinettiG MFinchC EMcNeillT H 1994 Neuroscience 62 425 http://dx.doi.org/10.1016/0306-4522(94)90377-8
- SchmidtC ELeachJ B 2003 Annu. Rev. Biomed.Eng. 5 293 http://dx.doi.org/10.1146/annurev.bioeng.5.011303.120731
- KimY THaftelV KKumarSBellamkondaR V 2008 Biomaterials 29 3117 http://dx.doi.org/10.1016/j.biomaterials.2008.03.042
- NisbetD RRoddaA EHorneM KForsytheJ SFinkelsteinD I 2009 Biomaterials 30 4573 http://dx.doi.org/10.1016/j.biomaterials.2009.05.011
- SchnellEKlinkhammerKBalzerSBrookGKleeDDaltonPMeyJ 2007 Biomaterials 28 3012 http://dx.doi.org/10.1016/j.biomaterials.2007.03.009
- Gerardo-NavaJFührmannTKlinkhammerKSeilerNMeyJKleeDMollerMDaltonP DBrookG A 2009 Nanomed 4 11 http://dx.doi.org/10.2217/17435889.4.1.11
- PrabhakaranM PRamakrishnaV S 2009 Acta Biomater. 5 2884 http://dx.doi.org/10.1016/j.actbio.2009.05.007
- WangYWangGChenLLiHYinTWangBLeeJYuQ 2009 Biofabrication 1 015001 http://dx.doi.org/10.1088/1758-5082/1/1/015001
- KimKLuuYChangCFangDHsiaoBChuBHadjiargyrouM 2004 J. Control. Release 98 47 http://dx.doi.org/10.1016/j.jconrel.2004.04.009
- IgnatiousFBaldoniJ 2002 US Patent Appl. No. 10/181,640
- KenawyEBowlinGMansfieldKLaymanJSimpsonDSandersEWnekG 2002 J. Control. Release 81 57 http://dx.doi.org/10.1016/S0168-3659(02)00041-X
- ZengJXuXChenXLiangQBianXYangLJingX 2003 J. Control. Release 92 227 http://dx.doi.org/10.1016/S0168-3659(03)00372-9
- ZhangYLimCRamakrishnaSHuangZ 2005 J. Mater. Sci. Mater. Med. 16 933 http://dx.doi.org/10.1007/s10856-005-4428-x
- CuiWLiXZhuXYuGZhouSWengJ 2006 Biomacromolecules 7 1623 http://dx.doi.org/10.1021/bm060057z
- VerreckGChunIRosenblattJPeetersJDijckAMenschJNoppeMBrewsterM 2003 J. Control. Release 92 349 http://dx.doi.org/10.1016/S0168-3659(03)00342-0
- CuiWQiMLiXHuangSZhouSWengJ 2008 Int. J. Pharm. 361 47 http://dx.doi.org/10.1016/j.ijpharm.2008.05.005
- QiMLiXYangYZhouS 2008 Eur. J. Pharm. Biopharm. 70 445 http://dx.doi.org/10.1016/j.ejpb.2008.05.003
- SunZZussmanEYarinAWendorffJGreinerA 2003 Adv. Mater. 15 1929 http://dx.doi.org/10.1002/adma.200305136
- GoldbergMLangerRJiaX 2007 J. Biomater. Sci. Polym. Ed. 18 241 http://dx.doi.org/10.1163/156856207779996931
- ChewSWenJYimELeongK 2005 Biomacromolecules 6 2017 http://dx.doi.org/10.1021/bm0501149
- NieHWangC 2007 J. Control. Release 120 111 http://dx.doi.org/10.1016/j.jconrel.2007.03.018
- NieHSohBFuYWangC 2008 Biotechnol. Bioeng. 99 223 http://dx.doi.org/10.1002/bit.21517
- BolgenNVargelIKorkusuzPMencelogluY ZPiskinE 2007 J. Biomed. Mater. Res. B 81 530
- CasperC LYamaguchiNKiickK LRaboltJ F 2005 Biomacromolecules 6 1998 http://dx.doi.org/10.1021/bm050007e