Abstract
This article reviews the properties and characterization of an intelligent thermoresponsive surface, which is a key technology for cell sheet-based tissue engineering. Intelligent thermoresponsive surfaces grafted with poly(N-isopropylacrylamide) exhibit hydrophilic/hydrophobic alteration in response to temperature change. Cultured cells are harvested on thermoresponsive cell culture dishes by decreasing the temperature without the use of digestive enzymes or chelating agents. Our group has developed cell sheet-based tissue engineering for therapeutic uses with single layer or multilayered cell sheets, which were recovered from the thermoresponsive cell culture dish. Using surface derivation techniques, we developed a new generation of thermoresponsive cell culture dishes to improve culture conditions. We also designed a new methodology for constructing well-defined organs using microfabrication techniques.
Introduction
Stimuli-responsive polymers can be used as switching sequences at the interface between artificial materials and biomolecules (peptides, proteins, nucleic acids and others), producing external, stimuli-induced changes in their structures and properties. ‘On–off’ switching of their respective functions can thus be achieved at the molecular level [Citation1–5]. Special attention is paid to thermoresponsive surfaces that have been prepared using a thermoresponsive polymer, poly(N-isopropylacrylamide) (PIPAAm). PIPAAm exhibits a reversible temperature-dependent phase transition in aqueous solutions at approximately 32 °C. The transition temperature is called the lower critical solution temperature (LCST) [Citation6, Citation7]. In biomedical applications, the thermoresponsive property of PIPAAm is widely used to switch between its functions as a temperature-dependent controlled release and gene delivery system [Citation8–11], a bioconjugated enzyme [Citation12, Citation13], and a microfluidic device [Citation14], among others.
Introduction of PIPAAm molecules onto a material changes the thermoresponsive soluble/insoluble properties of its surface. This alteration of the PIPAAm-modified intelligent surface can be used to modulate its interactions with solutes in the chromatographic separation [Citation15–20] and with cultured cells [Citation21–23]. PIPAAm-grafted chromatographic matrices allow us to regulate the retention of steroids [Citation15, Citation16], polypeptides and proteins [Citation17, Citation18], and nucleotides [Citation19, Citation20] by changing the temperature under the aqueous condition. This is an alternative to reverse-phase chromatography, where separation is controlled by regulating the interaction between octadecyl stationary phase surfaces and solute molecules through mobile phase composition and polarity. This system has the advantage of maintaining the biological activity of peptides as well as proteins and reducing pollution from the organic mobile phases commonly used in reverse-phase chromatography. In addition, PIPAAm-grafted cell culture substrates exhibit a thermoresponse in cell attachment/detachment. Cultured cells are harvested when temperature is decreased from 37 to 20 °C without the use of digestive enzymes or chelating agents. A confluent cultured cell monolayer, which is detached from the PIPAAm-grafted cell culture substrate by decreasing the temperature, is used in regenerative medicine [Citation24–26]. Thermoresponsive cell culture dishes, grafted by controlling the thickness of the thermoresponsive polymeric layer, allow recovery of confluent cell [Citation27, Citation28].
This paper focuses on the preparation and characterization of intelligent thermoresponsive surfaces for cell culture substrates. The application of harvested cell sheets from thermoresponsive cell culture dishes and the development of the next generation of thermoresponsive intelligent surfaces are also reviewed.
Preparation and characterization of PIPAAm-grafted surfaces
PIPAAm molecules grafted onto solid materials exhibit thermoresponsive soluble/insoluble changes due to the hydration/dehydration of PIPAAm side chains, and this changes physicochemical surface properties, such as wettability (figure ). We prepared free-end and multipoint attached PIPAAm surfaces using a coupling reaction between aminated glass coverslips and the carboxyl groups of semitelechelic PIPAAm or poly(IPAAm-co-acrylic acid) (poly(IPAAm-co-AAc)). We also investigated temperature-dependent effects on surface wettability using the Wilhelmy plate technique [Citation29]. A large contact angle change was observed on free-end PIPAAm-grafted surfaces at approximately 24 °C, while a small change in contact angle was observed on poly(IPAAm-co-AAc)-grafted surfaces over a wide temperature range. The transition temperatures of both surfaces were lower than that of soluble PIPAAm in water, probably due to the influence of the hydrophobicity of the basal polystyrene derivative and the density of the grafted PIPAAm chains. The small contact angle changes of the multi-point attached PIPAAm surfaces were likely caused by the restricted chain conformation of the grafted polymers.
Figure 1 Schematics of the temperature-dependent change in wettability on the PIPAAm-grafted surface at 10 and 37 °C.
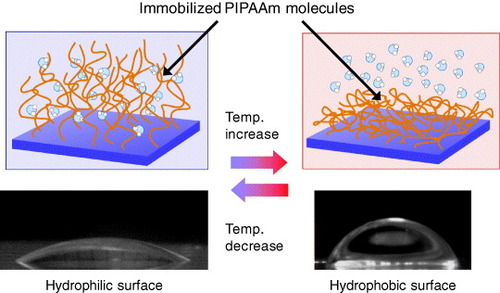
Our group has also reported temperature-dependent variations in the meniscus height within PIPAAm-grafted capillary lumen resulting from thermoresponsive hydration changes in surface-grafted PIPAAm [Citation30]. We observed a large change in meniscus heights within PIPAAm-grafted capillaries when the temperature was varied between 30 and 40 °C. The calculated contact angles of PIPAAm-grafted capillaries at specific temperatures remained nearly constant regardless of the inner diameter of the capillary. Scanning electron microscopy (SEM) revealed that the change in the diameter of the PIPAAm-grafted capillary lumen was ∼1% compared with that of an unmodified capillary lumen. Moreover, the changes in the wet thickness of the grafted PIPAAm during the phase transition were nanoscopic: the thicknesses were 143 nm at 25 °C and 68 nm at 35 °C, as confirmed by atomic force microscopy measurements in water. Therefore, temperature-dependent changes in the meniscus height within the PIPAAm-grafted capillary were due to the hydrophilic/hydrophobic alteration of PIPAAm-grafted surface transitions.
Using electron irradiation, uniformly spread IPAAm monomers were polymerized and covalently immobilized onto the surface of the tissue culture polystyrene dish (TCPS) [Citation21–23]. Although electron irradiation requires expensive equipment, this method facilitates large-scale production of thermoresponsive TCPS. Based on our technologies for thermoresponsive surfaces, a thermoresponsive TCPS dish UpCellTM has been marketed globally by NuncTM. Various types of cells adhere to and proliferate on the surface of thermoresponsive TCPS at 37 °C, but do not adhere to these surfaces at 20 °C. Cells cultured on hydrophobized thermoresponsive TCPS at 37 °C detach when the temperature is lowered to 20 °C. At 20 °C, the surface becomes hydrophilic, as the hydration/dehydration of the PIPAAm is altered at 32 °C. Applications of the thermoresponsive culture dish are discussed in sections 3 and 4.
To determine whether the immobilization of PIPAAm was successful, it is very important to assess PIPAAm-grafted surfaces using analytical techniques. Qualitative and quantitative analyses of grafted PIPAAm on inorganic substrates such as silicon, glass, and quartz can be performed relatively easily by x-ray photoelectron spectroscopy (XPS), atomic force microscopy (AFM), ellipsometry, surface plasmon resonance, time-of-flight secondary-ion mass spectroscopy (ToF-SIMS), x-ray reflectometry, or neutron reflectometry. By contrast, PIPAAm grafted on polymeric substrates requires sensitive and selective detection because signals of grafted PIPAAm are generally similar to those of the polymeric substrates.
XPS is the best means of characterizing surface-oriented PIPAAm on polymeric substrates qualitatively. Dense nanoparticle-like domains of PIPAAm can be observed on TCPS surfaces using AFM [Citation31]. However, a uniform coverage of PIPAAm on the surface can be characterized by XPS analyses, such as the angle-dependent intensity of the PIPAAm signal [Citation32] and by the absence of π-π∗ shake-up peaks derived from polystyrene [Citation31]. The atomic composition of carbon, nitrogen, and oxygen on the surface of PIPAAm-grafted TCPS is in agreement with the predicted values based on the stoichiometric ratio of the IPAAm monomer [Citation31, Citation32]. Moreover, the decomposed C1 s peaks (one sp2- and three sp3-hybridized carbon atoms) match the composition expected from the repeating unit of PIPAAm [Citation31]. These XPS analyses indicate that the molecular structure of repeating IPAAAm units is retained.
Attenuated total reflection Fourier transform infrared spectroscopy (ATR/FT-IR) can also be used for quantitative characterization. The amount of grafted PIPAAm on the surface of thermoresponsive TCPS is determined by ATR/FT-IR. For TCPS, absorption arising from monosubstituted aromatic rings is observed at 1600 cm−1. Absorption of the amide carbonyl derived from PIPAAm appears in the region of 1650 cm−1 [Citation31]. The ratio of peak intensities, (I1650)/(I1600), is used to determine the amount of grafted PIPAAm on the surface. The amounts of grafted PIPAAm on TCPS significantly influence the cell adhesion behavior [Citation33]. Bovine endothelial cells adhere to the PIPAAm-grafted TCPS when the density is less than 1.4 μg cm−2 at 37 °C, and the adhered cells can detach from the surfaces between 0.8 and 1.4 μg cm−2 at 15 °C. Cells cannot adhere to surfaces with high amounts of grafted PIPAAm chains for several reasons, as discussed in section 3.
Determining the thickness of PIPAAm on TCPS by optical measurements such as ellipsometry and surface plasmon resonance is very difficult because the refractive index of PIPAAm is similar to that of the polymeric substrates. Our group has estimated the thickness of PIPAAm on TCPS surfaces by AFM measurement after UV excimer ablation [Citation27]. To selectively remove the PIPAAm layer from the TCPS substrate, an ArF excimer laser (193 nm) was focused on a 30×25 μm region, and the ablation extent of PIPAAm was regulated by the number of excimer laser shots. The ablated region where the hydrophobic TCPS surfaces were exposed was stained with a hydrophobic fluorescent dye, namely 1,1′-dioctadecyl-3,3,3′,3′-tetramethylindocarbocyanine perchlorate (DiIC18). ToF-SIMS imaging indicated that the laser-ablated TCPS domains were detected only where the fluorescent DiIC18 was stained. After selective ablation of PIPAAm layers, the thickness was estimated from the gap between the top of the PIPAAm layer and the laser-ablated TCPS surface by AFM measurement. Therefore, a combination of the selective laser-ablation of PIPAAm and fluorescent imaging allowed us to estimate the thickness of grafted PIPAAm on TCPS.
Thermoresponsive cell culture dish
Cells initially stick to the surface of a material due to physicochemical interactions such as hydrophobic interactions, Coulomb forces, and van der Waals forces. This initial process is called ‘passive adhesion’. Then, the cells bind to a specific site on adhesive proteins, such as binding to fibronectin on the surface of materials by means of a receptor protein called integrin. Integrins also play a role in cell signaling; when integrins engage with molecules in the extracellular matrix, intracellular signaling cascades are activated through protein kinases that associate with the intracellular end of the integrin molecule. Such processes that require ATP metabolism are termed ‘active adhesion’. A conventional culture method is used to grow cells on the surface of a TCPS dish. Cells are then harvested by the enzymatic proteolysis of an extracellular matrix (ECM), such as trypsin, and by chelating Ca2+ ions to disrupt cell–cell junctions with a substance such as EDTA.
Since 1990, our group has been developing the thermoresponsive cell culture dish grafted with PIPAAm on the surface, allowing cultured cells to be recovered by simply lowering the temperature below LCST (figure (a)) [Citation21–23]. When the temperature is lowered, morphological changes to cells and the detachment of cells are suppressed by an ATP synthesis inhibitor, sodium azide, and a tyrosine kinase inhibitor, genistein [Citation23, Citation34]. An actin filament stabilizer, phalloidin, and its depolymerizer, cytochalasin D, also inhibit cell detachment [Citation34]. Cell detachment from the thermoresponsive cell culture dish is an active process induced by intracellular events, such as signaling and cytoskeleton reconstruction. The ‘deadhesion’ process is inherent on the surface of the thermoresponsive cell culture dish. The cells recovered from the thermoresponsive cell culture dish retain their cellular structure and functions, while trypsinization causes damage to the cell membrane and ECM [Citation21–23]. Thus, the cell culture method using the thermoresponsive cell culture dish is considered a powerful tool for investigating the molecular machinery involved in cell-surface detachment.
Figure 2 Diagrams illustrating the concept of cell sheet engineering. (a) Schematic diagrams for the interactions of the thermoresponsive surface with the cell. (b) A recovered cell sheet from the thermoresponsive cell culture dish retains the extracellular matrix beneath the cell sheet and cell–cell junctions.
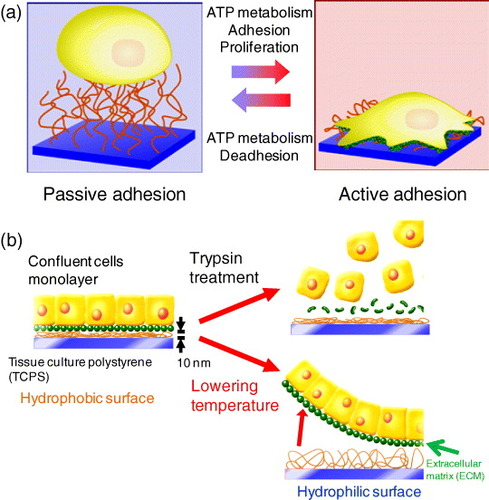
Cells cultured to confluence on the surface of hydrophobized PIPAAm-grafted TCPS at 37 °C were detached as a single cell sheet when the temperature was lowered to 20 °C because the surface became hydrophilic below the LCST of PIPAAm (figure (b)) [Citation35]. The membrane protein and ECM on the cell sheet were retained because the cell sheets were recovered simply by lowering the temperature, with no enzymatic proteolysis treatment (figure (b)). In fact, fluorescent-stained fibronectin located beneath cultured cells was removed from the surface of thermoresponsive TCPS dish after the temperature was reduced [Citation35].
Temperature-induced alteration of cell adhesion/detachment has been observed on ultrathin PIPAAm-grafted TCPS with a dry thickness of 15 nm, but cell adhesion dramatically decreases on the surface grafted with a thicker PIPAAm layer (>30 nm) [Citation27]. Within the nanometer-scale ultrathin layer, the motion of PIPAAm chains is strongly restricted by immobilization onto the TCPS surface. Moreover, the hydrophobic surface of the TCPS decreases the hydration of grafted PIPAAm chains in the vicinity of the surface. These factors are thought to enhance the dehydration and aggregation of the PIPAAm chains. In contrast, the hydrophobic environment of the TCPS surface has little influence on the grafted PIPAAm layer over a thickness of 30 nm, causing hydration and increasing the mobility of the grafted PIPAAm chains.
Cell sheet-based tissue engineering and its clinical applications
Tissue engineering using biodegradable scaffolds has been proposed by Langer and Vacanti [Citation36] as a promising methodology for the in vitro reconstruction of three-dimensional tissues. However, clinical applications using biodegradable scaffold-based tissue engineering have been limited to artificial skin [Citation37], blood vessels [Citation38] and bladder [Citation39]. This method is not suitable for regenerating cell-dense tissues, including cardiac muscle, liver and kidney tissues. In addition, seeded cells survive only on the periphery of the scaffolds due to the limited diffusion of nutrients, such as oxygen and glucose, and due to waste metabolites.
A new approach called ‘cell sheet engineering’ has been used to construct ideal transplantable tissues composed exclusively of cells. Our group has developed a novel means of constructing three-dimensional tissues from cell sheets using a thermoresponsive cell culture dish [Citation24–26]. The recovered cell sheets bear the ECM on the bottom side, and, as a result, it is possible to transfer the cell sheets onto other materials, such as a culture dish, another cell sheet, or a biological tissue (figure (b)). Intrinsically, sheet-like tissues located on the surface of the body, such as epidermal keratinocytes [Citation40] and corneal epithelial cell sheets [Citation41], are easily transplantable. Our group has applied cell sheet engineering to clinical settings in plastic reconstructive surgery [Citation40], ophthalmology [Citation41], dilated cardiomyopathy [Citation42–44], and esophagus ulceration [Citation45, Citation46].
Our first clinical investigation was the treatment of patients with skin defects. Epidermal keratinocytes were cultured on thermoresponsive cell culture dishes with mitomycin-C-treated 3T3 feeder layer cells and harvested as multilayered cell sheets [Citation40]. Autologous keratinocyte sheets were transplanted to patients with burn injuries or scars.
In collaboration with Nishida et al, patients were treated with unilateral or bilateral total corneal stem-cell deficiencies arising from alkali burns or Stevens–Johnson syndrome (figure ) [Citation41]. In patients with a unilateral limbal stem-cell deficiency, a corneal epithelial cell sheet was cultured from autologous limbal stem cells. An autologous oral mucosal epithelial cell sheet was also applied to bilateral total corneal stem-cell deficiencies. In France, CellSeed Inc., a bioventure developed from Tokyo Women's Medical University, has begun a clinical trial to treat patients suffering from bilateral limbal stem cell deficiency with transplanted autologous oral mucosal epithelial cell sheets.
Figure 3 Transplantation of a corneal epithelial cell sheet. From a small biopsy of oral mucosal tissue (3×3 mm) from a patient's cheek, isolated epithelial cells are seeded onto thermoresponsive cell culture inserts. After being cultured for two weeks at 37 °C in the presence of 3T3 feeder layers, the cells grow to form a multilayered epithelial cell sheet. By reducing the temperature to 20 °C for 30 min, the cell sheet is harvested from the thermoresponsive cell culture insert and is then transplanted directly onto the diseased eye without sutures. (Reprinted with permission from [Citation41] © 2004 Massachusetts Medical Society.)
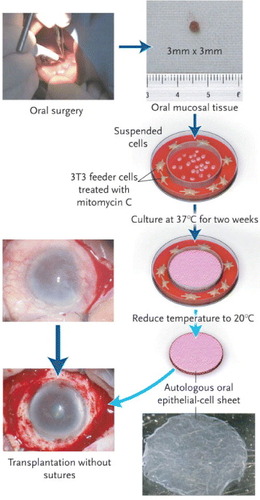
When a cardiac patch was fabricated by layering myocardial cell sheets harvested from the thermoresponsive cell culture dish, spontaneous pulsating of the patch was easily visible to the naked eye [Citation47]. Layered myocardial cell sheets transplanted to infarcted hearts demonstrated morphological communication with the host myocardium, and they improved the host heart function by increasing the ejection fraction and inhibiting dilation of the left ventricle [Citation42–44]. In collaboration with Sawa et al, layered autologous skeletal myoblast sheets have been used to treat dilated cardiomyopathy [Citation42], and clinical investigations of this treatment are being conducted.
Autologous mucosal epithelial cell sheets were transplanted for the treatment of esophageal ulcerations to prevent stenosis. A large animal model was examined by combining an endoscopic submucosal dissection and the endoscopic transplantation of autologous oral mucosal epithelial cell sheets. Complete wound healing without stenosis was confirmed 4 weeks after surgery (figure ) [Citation45]. The clinical investigation began in January 2008, and the treatment was found to promote wound healing, prevent inflammation and constriction, and improve the patients’ post-operative quality of life [Citation46].
Figure 4 Transplantation of oral mucosal epithelial cell sheets for the treatment of esophageal ulcerations to prevent stenosis. Left and right panels represent transplant and control groups, respectively. (a, c) Endoscopic photographs taken 4 weeks after operation. (b, d) Macroscopic images of the esophageal sites receiving endoscopic submucosal dissection after 4 weeks. (e, f) Hematoxylin and eosin (H&E) staining of the central portions of the ulcer sites. (g) H&E staining of the border region between the transplanted cell sheet and the outer portions of the ulcer site. (h) Comparison of the number of inflammatory cells present in the surgical sites between transplant and control groups. ∗p=0.01. (Reprinted with permission from [Citation45] © 2006 BMJ Publishing Group Ltd.)
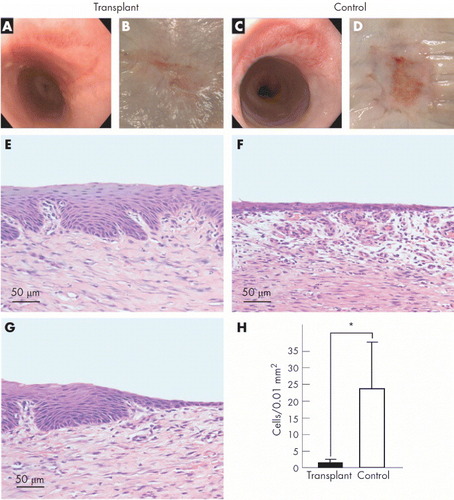
Periodontal treatment using periodontal ligament-derived cell sheets has been applied to defects in rats [Citation48] and beagles [Citation49] to regenerate damaged periodontal support. In both cases, periodontal regeneration with bone, periodontal ligament, and cementum formation was observed. In the clinical application, transplantable multi-layered periodontal ligament-derived cell sheets were successfully fabricated with woven poly(glycolic acid), and bone defects were filled with porous β-tricalcium phosphate (figure ) [Citation50]. Transplantation of periodontal ligament-derived cell sheets was found to induce true simultaneous periodontal regeneration, including alveolar bone, cementum, and well-oriented fibers. This transplantation was found to be useful for periodontal regeneration in clinical settings.
Figure 5 Periodontal treatment using a periodontal ligament-derived (PDL) cell sheet. (a) Schematic illustration of artificial periodontal defects and a three-layered cell sheet transplantation. After the three-wall bone defect was created, the root surface was curetted to remove all periodontal ligaments and cementum. Then, three-layered PDL cell sheets supported by woven poly(glycol acid) (PGA) were transplanted onto the root surface. After the cell sheets were transplanted, porous β-tricalcium phosphate (β-TCP) was filled in the infrabony defect. Photomicrographs show contralateral defect sites receiving β-TCP (b) and PDL cell sheet/β-TCP (C). Note that functionally well-oriented periodontal fibers with newly formed bone were only observed in the experimental group (c; bar, 1 mm; Azan staining). (Reprinted with permission from [Citation50] © 2009 Elsevier.)
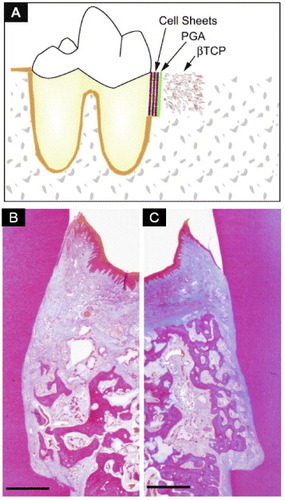
A novel lung air leak sealant has also been developed using autologous cell sheets derived from skin fibroblasts [Citation51]. In contrast to conventional materials such as fibrin glue, the cell sheets immediately and permanently seal air leaks in a dynamic manner. This allows for the extensive tissue contraction and expansion involved in respiration without any postoperative recurrences. In addition, host mesothelial cells migrate to cover the transplanted cell sheets, confirming excellent biocompatibility and integration with the host tissues. Recently, a novel device in endoscopic surgery was developed to transplant cell sheets with minimal invasiveness [Citation52]. With this device, square cell sheets are successfully transplanted onto wound sites of porcine lungs placed in a human body model. These studies have shown that an immediate and permanent lung air leak sealant combined with the transplantation device is suitable for future clinical applications.
Other applications using cell sheet engineering, such as liver [Citation53], pancreatic islet [Citation54], and bladder augmentation [Citation55], have been investigated and summarized in other reviews [Citation24–26]. Recent progress on the next generation of thermoresponsive culture dishes is discussed in the next section.
The next generation of thermoresponsive cell culture dishes
Biomolecule-immobilizing thermoresponsive cell culture dishes
To introduce bioactive molecules onto the surface of a thermoresponsive cell culture dish, an isopropylacrylamide analogue, 2-carboxyisopropylacrylamide (CIPAAm) with a carboxylate side chain, is copolymerized with IPAAm [Citation56, Citation57]. Covalent immobilization of the RGDS (Arg-Gly-Asp-Ser) peptide is performed through an amide bond onto a poly(IPAAm-co-CIPAAm)-grafted TCPS dish (figures (a) and (b)) [Citation58, Citation59]. Cells adhere and spread onto the RGDS-immobilized thermoresponsive TCPS in the absence of serum at 37 °C. When the temperature is reduced to 20 °C, the cells are detached from the surface. On surfaces in which an extensible poly(ethylene glycol) (PEG) spacer occurs between the RGD peptide and the grafted thermoresponsive polymer (figures (c) and (d)), the time required to release cells from the surface is longer than on surfaces immobilized directly with RGD [Citation60]. In another immobilization method, biotinylated biomolecules are coupled onto streptavidin-immobilized thermoresponsive TCPS via affinity binding [Citation61]. Biotinylated RGDS peptide serves as a model substrate with glycine as a spacer, and it is easily conjugated onto the surface without coupling reagents such as water-soluble carbodiimides and active esters. In addition, the co-immobilization of RGDS and PHSRN (Pro-His-Ser-Arg-Asn) sequences onto the poly(IPAAm-co-CIPAAm)-grafted TCPS enhance the synergistic binding to α5β1 integrin, resulting in stable adhesion and cell spreading. In particular, the immobilization of PHSRN-G6-RGDS dramatically delays the rate of cell detachment because the length of G6 roughly corresponds to the spatial distance between PHSRN and RGDS in native fibronectin [Citation62].
Figure 6 Schematic of cell adhesive peptide-bearing thermoresponsive surfaces showing thermally induced ‘on–off’ control of integrin-peptide binding. The grafted thermoresponsive polymers (orange) expose tethered peptides (red) to integrins (dark blue) at the ‘on’ state above the LCST, and they shield the peptides from integrin access at the ‘off’ state below the LCST (a, b). Employing extensible PEG spacers (light blue) between the peptides and the grafted polymer reduced the shielding effect of the protective polymer layer (c, d). (Reprinted with permission from [Citation60] © 2008 Elsevier.)
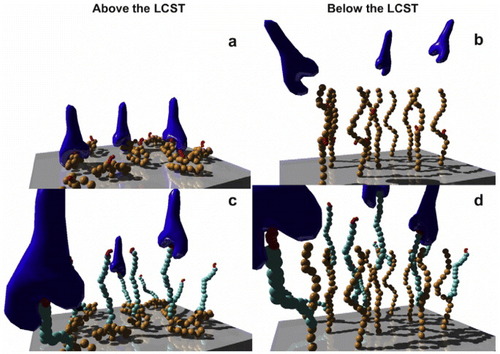
These surfaces facilitate the spreading of cells without serum via affinity interactions between the immobilized RGDS on poly(IPAAm-co-CIPAAm) chains and the integrin receptor at 37 °C (above the LCST). In contrast, the cells that spread at 37 °C detach spontaneously when the temperature is lowered below the LCST (20 °C). This occurs because the hydrated poly(IPAAm-co-CIPAAm) chains extend and spatially prevent the integrin from accessing the immobilized RGDS ligands. This system shows on/off affinity switching of integrin-RGDS binding only when the temperature is changed above/below the LCST. This method allows serum-free cell culturing and trypsin-free cell harvesting, which essentially eliminates components from mammalian sources from the culture process.
Micropatterned thermoresponsive cell culture dishes
Cellular micropatterning technology is a powerful tool for studying cellular behaviors such as cell–cell and cell–ECM interactions, as well as for mimicking heterotypic cellular architecture in vitro. Two-dimensional micropatterning of heterotypic cells has been investigated by photolithography [Citation63], soft lithography [Citation64, Citation65], and dielectrophoretic methods [Citation66]. In addition, three-dimensional cellular micropatterning has been developed using several different cellular printing systems [Citation67, Citation68]. However, the regeneration of complicated tissues and organs remains a challenging issue, mainly due to a lack of (i) three-dimensional tissues with microscopically arranged, high-density cells and (ii) vascularized, multi-functional tissues replicating the native structure.
To fabricate complicated three-dimensional tissues, our group has focused on developing micropatterned thermoresponsive surfaces exhibiting dual phase transition temperatures to enable both the culture and the recovery of patterned heterotypic cell sheets (figure ) [Citation69, Citation70]. Two types of thermoresponsive polymers exhibiting different LCSTs, namely PIPAAm and n-butyl methacrylate (BMA)-co-grafted PIPAAm, can be micropatterned using electron beam induced polymerization with stainless masks. The micropatterned surface exhibiting hydrophilic/hydrophobic property alterations allows the selective adhesion and growth of rat primary hepatocytes (HC) and bovine carotid endothelial cells (EC). At 27 °C, the seeded HCs adhere to the hydrophobized poly(IPAAm-BMA) co-grafted domains and not to the hydrophilic PIPAAm domains (figure (a)). When the temperature is increased to 37 °C, the secondary seeding of ECs allowed exclusive adhesion to the hydrophobized PIPAAm domains, resulting in patterned co-culture with HC (figure (b)). Co-cultured, patterned cell sheets are recovered by the hydration of both of the polymer-grafted domains when temperature decreases to 20 °C (figure (c)).
Figure 7 Schematic representation of the method of patterning cell co-culture and harvesting co-cultured cell sheets using a micropatterned thermoresponsive surface exhibiting a dual phase transition temperature. (a) First, HCs are seeded and cultured at 27 °C, causing the localization of hepatocytes onto P(IPAAm-BMA) co-grafted islands showing hydrophobic nature. (b) Second, ECs are seeded and cultured at 37 °C, resulting in the generation of patterned co-cultures. (c) Decreasing the temperature to 20 °C induced the detachment of a co-cultured cell sheet. Harvested patterned co-cultured cell sheets are shown on the right. The bar represents 1 cm. (Reprinted with permission from [Citation70] © 2006 Elsevier.)
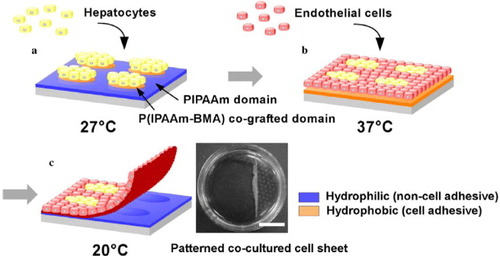
Recently, our group achieved precise, high-resolution micropatterning of cell sheets using a maskless photolithography method. An all-in-one device, which is equipped with a commercially available liquid crystal display projector and an objective lens for reduced projection, is used to expose patterned images onto a photoresist-coated substrate without the use of a mask [Citation71, Citation72]. The projection images are easily generated on a computer equipped with commercial software, so special software is not needed for lithographic pattern generation. With this device, poly(dimethylsiloxane) (PDMS) microstructure [Citation72–76] is prepared as a stamp and applied to printing fibronectin onto the surface of the thermoresponsive cell culture dish. Under serum-free conditions, HCs are attached selectively onto fibronectin-printed domains. Secondary seeding of ECs in the presence of serum forms a micropatterned co-culture with HCs. Finally, all of the cells are harvested as a single micropatterned cell sheet when temperature is decreased to 20 °C. The recovered co-cultured cell sheet is transferable, providing a novel technology to prepare tissue-mimicking multi-layer materials by overlaying co-cultured cell sheets.
The in vitro formation of capillary networks is considered a key factor in the creation of vascularized tissues replicating the native structure of the tissues. To construct three-dimensional tissues with capillary networks, our group has prepared micropatterned cell sheets using a micropatterned thermoresponsive surface fabricated by a maskless photolithography method [Citation77]. ECs selectively adhere to 20 μm-wide micropatterned PIPAAm domains separated by 60 μm from non-adhesive polyacrylamide (PAAm) domains at 37 °C. Stratified tissues are constructed by layering fibroblast sheets with the micropatterned ECs harvested from the thermoresponsive micropatterned surface. The fidelity of the micropatterned ECs within the multi-layered tissue is maintained after assembly, leading the ECs to self-organize into capillary-like networks after a 5-day culture period (figure ). Our previous work has shown that the networks of ECs formed within stratified myocardial cell sheets can promote the formation of tubules and can connect to the host in vivo after transplantation [Citation78]. This technique is promising for studying cell-cell communications and angiogenesis in reconstructed, three-dimensional environments, as well as for fabricating tissues with complex, multicellular architectures.
Figure 8 Fluorescently stained three-dimensional tissue constructs containing both micropatterned ECs and fibroblast cell sheets generated using the micropatterned thermoresponsive surfaces. Rhodamine-conjugated ulex europaeus agglutinin-I (red) for ECs and Alexa488-conjugated phalloidin (green) were used to stain F-actin in both ECs and fibroblasts. (a–c) Three-layer tissue after layering but before further culture. (d–f) Five-layer tissue after layering but before further culture. (g–i) Three-layer tissue after 5 days of cultivation at 37 °C after layering procedures; (a), (d) and (g) show fluorescent micrographs at a lower magnification. Scale bars represent a length of 5 μm. Panels (b), (e) and (h) show confocal laser micrographs, and (c), (f) and (i) show cross-sectional views (of (b), (e) and (h), respectively). Scale bars represent 100 μm. (Reprinted with permission from [Citation77] © 2007 Elsevier.)
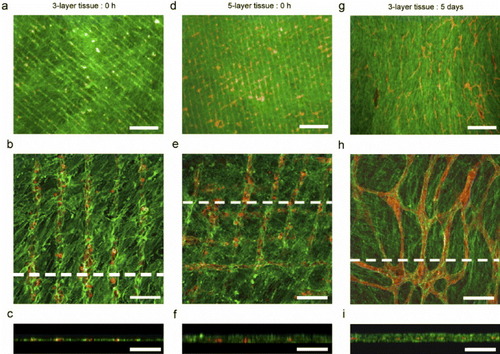
Conclusions
This review introduced the preparation and characterization of thermoresponsive cell culture dishes and their application in cell sheet-based tissue engineering. Thermoresponsive cell culture dishes, grafted with a thermoresponsive polymeric layer with a dry thickness of 15 nm, allow confluent cell sheets to be recovered. Development of the thermoresponsive cell culture dish facilitates successful cell sheet-based therapies. We have already applied cell sheet engineering to clinical settings in the treatment of skin defects, ophthalmology, dilated cardiomyopathy, and esophagus ulcerations. The next generation of thermoresponsive culture dishes has been developed using surface functionality and microfabrication. By tailoring the composition of thermoresponsive polymers and their derivatives, the interactions with biomolecules and cells on the surface are switched based on temperature triggers. Microfabricated thermoresponsive surfaces would allow the reconstruction of more complex tissues to potentially treat a wide range of diseases. Consequently, the coordination and/or fusion of medicine and engineering are essential for furthering biomedical engineering.
Acknowledgments
Part of this work was financially supported by the Formation of Innovation Center for the Fusion of Advanced Technologies in the Special Coordination Funds for Promoting Science and Technology, the High-Tech Research Center Program, and by Grant-in-Aid for Young Scientists (B) No. 20700398 from the Ministry of Education, Culture, Sports, Science and Technology (MEXT).
References
- OkanoTYoshidaR 1993 Biomedical Applications of Polymeric Materials TTsuruta THayashi KKataoka KIshihara YKimura Boca Raton, FL CRC Press p 407
- OkanoT 1993 Advances in Polymer Science, Responsive Gels; Volume Transition II KDusek Berlin Springer p 179
- OkanoTYuiNYokoyamaMYoshidaR 1994 Advances in Polymeric Systems for Drug Delivery Switzerland Gordon and Breach
- OkanoT 1998 Biorelated Polymers and Gels: Controlled Release and Applications in Biomedical Engineering Chestnut Hill Academic
- HoffmanA 1995 Artif. Organs 19 458 http://dx.doi.org/10.1111/j.1525-1594.1995.tb02359.x
- HeskinsMGuilletJ E 1968 J. Macromol. Sci. Chem. A 2 1441 http://dx.doi.org/10.1080/10601326808051910
- BaeY HOkanoTKimS W 1990 J. Polym. Sci. B: Polym. Phys. 28 923 http://dx.doi.org/10.1002/polb.1990.090280609
- CammasSSuzukiKSoneCSakuraiYKataokaKOkanoT 1997 J. Control. Release 48 157 http://dx.doi.org/10.1016/S0168-3659(97)00040-0
- ChungJ EYokoyamaMYamatoMAoyagiTSakuraiYOkanoT 1999 J. Control. Release 62 115 http://dx.doi.org/10.1016/S0168-3659(99)00029-2
- KurisawaMYokoyamaMOkanoT 2000 J. Control. Release 69 127 http://dx.doi.org/10.1016/S0168-3659(00)00297-2
- TakedaNNakamuraEYokoyamaMOkanoT 2000 J. Control. Release 95 343 http://dx.doi.org/10.1016/j.jconrel.2003.12.001
- ChilkotiAChenGStaytonP SHoffmanA S 1994 Bioconj. Chem. 5 504 http://dx.doi.org/10.1021/bc00030a004
- MatsukataMTakeiYAokiTSanuiKOgataNSakuraiYOkanoT 1994 J. Biochem. 116 682
- YuCMutluSSelvaganapathyPMastrangeloC HSvecFFréchetJ M J 2003 Anal. Chem. 75 1958 http://dx.doi.org/10.1021/ac026455j
- KanazawaHYamamotoKMatsushimaYTakaiNKikuchiASakuraiYOkanoT 1996 Anal. Chem. 68 100 http://dx.doi.org/10.1021/ac950359j
- YakushijiTSakaiKKikuchiAAoyagiTSakuraiYOkanoT 1999 Anal. Chem. 71 1125 http://dx.doi.org/10.1021/ac980677t
- KanazawaHKashiwaseYYamamotoKMatsushimaYKikuchiASakuraiYOkanoT 1997 Anal. Chem. 69 823 http://dx.doi.org/10.1021/ac961024k
- KanazawaHYamamotoKKashiwaseYMatsushimaYTakaiNKikuchiASakuraiYOkanoT 1997 J. Pharm. Biomed. Anal. 15 1545 http://dx.doi.org/10.1016/S0731-7085(96)02004-3
- AyanoESakamotoCKanazawaHKikuchiAOkanoT 2006 Anal. Sci. 12 539 http://dx.doi.org/10.2116/analsci.22.539
- KikuchiAKobayashiJOkanoTIwasaTSakaiK 2007 J. Bioact. Compat. Polym. 22 575 http://dx.doi.org/10.1177/0883911507084294
- YamadaNOkanoTSakaiHKarikusaFSawasakiYSakuraiY 1990 Macromol. Chem., Rapid Commun. 11 571 http://dx.doi.org/10.1002/marc.1990.030111109
- OkanoTYamadaNSakaiHSakuraiY 1993 J. Biomed. Mater. Res. 27 1243 http://dx.doi.org/10.1002/jbm.820271005
- OkanoTYamadaNOkuharaMSakaiHSakuraiY 1995 Biomaterials 16 297 http://dx.doi.org/10.1016/0142-9612(95)93257-E
- YamatoMOkanoT 2004 Mater. Today 7 42 http://dx.doi.org/10.1016/S1369-7021(04)00234-2
- YangJYamatoMShimizuTSekineHOhashiKKanzakiMOhkiTNishidaKOkanoT 2007 Biomaterials 28 5033 http://dx.doi.org/10.1016/j.biomaterials.2007.07.052
- YamatoMAkiyamaYKobayashiJJosephYKikuchiAOkanoT 2007 Prog. Polym. Sci. 32 1123 http://dx.doi.org/10.1016/j.progpolymsci.2007.06.002
- AkiyamaYKikuchiAYamatoMOkanoT 2004 Langmuir 20 5506 http://dx.doi.org/10.1021/la036139f
- FukumoriKAkiyamaYYamatoMKobayashiJSakaiKOkanoT 2009 Acta Biomater. 5 470 http://dx.doi.org/10.1016/j.actbio.2008.06.018
- TakeiY GAokiTSanuiKOgataNOkanoTSakuraiY 1994 Macromolecules 27 6163 http://dx.doi.org/10.1021/ma00099a035
- IdotaNKikuchiAKobayashiJSakaiKOkanoT 2005 Adv. Mater. 17 2723 http://dx.doi.org/10.1002/adma.200402068
- AkiyamaYKushidaAYamatoMKikuchiAOkanoT 2007 J. Nanosci. Nanotechnol. 7 796 http://dx.doi.org/10.1166/jnn.2007.509
- UchidaKSakaiKItoEKwonO HKikuchiAYamatoMOkanoT 2000 Biomaterials 21 923 http://dx.doi.org/10.1016/S0142-9612(99)00260-4
- SakaiHDoiYOkanoTYamadaNSakuraiY 1996 Advanced Biomaterials in Biomedical Engineering and Drug Delivery Systems NOgata S WKim JFeijen TOkano Tokyo Springer p 229
- YamatoMOkuharaMKarikusaFKikuchiASakuraiYOkanoT 1999 J. Biomed. Mater. Res. 44 44 http://dx.doi.org/10.1002/(SICI)1097-4636(199901)44:1<44::AID-JBM5>3.0.CO;2-X
- KushidaAYamatoMKonnoCKikuchiASakuraiYOkanoT 1999 J. Biomed. Mater. Res. 45 355 http://dx.doi.org/10.1002/(SICI)1097-4636(19990615)45:4<355::AID-JBM10>3.0.CO;2-7
- LangerRVacantiJ P 1993 Science 260 920 http://dx.doi.org/10.1126/science.8493529
- HansbroughJ FMozingoD WKealeyG PDavisMGidnerAGentzkowG D 1997 J. Burn Care Rehabil. 18 43 http://dx.doi.org/10.1097/00004630-199701000-00008
- Shin'okaTImaiYIkadaY 2001 N. Engl. J. Med. 344 532 http://dx.doi.org/10.1056/NEJM200102153440717
- AtalaABauerS BSokerSYooJ JRetikA B 2006 Lancet 367 1241 http://dx.doi.org/10.1016/S0140-6736(06)68438-9
- YamatoMUtumiMKushidaAKonnoCKikuchiAOkanoT 2001 Tissue Eng. 7 473 http://dx.doi.org/10.1089/10763270152436517
- NishidaK et al 2004 N. Engl. J. Med. 351 1187 http://dx.doi.org/10.1056/NEJMoa040455
- MemonI A et al 2005 J. Thorac. Cardiovasc. Surg. 130 1333 http://dx.doi.org/10.1016/j.jtcvs.2005.07.023
- MiyagawaS et al 2005 Transplantation 80 1586 http://dx.doi.org/10.1097/01.tp.0000181163.69108.dd
- SekineHShimizuTKosakaSKobayashiEOkanoT 2006 J. Heart Lung Transplant. 25 324 http://dx.doi.org/10.1016/j.healun.2005.09.017
- OhkiTYamatoMMurakamiDTakagiRYangJNamikiHOkanoTTakasakiK 2006 Gut 55 1704 http://dx.doi.org/10.1136/gut.2005.088518
- OhkiTYamatoMOtaMMurakamiDTakagiRKondoMNakamuraTOkanoTYamamotoM 2009 Gastrointest. Endosc. 69 AB253 http://dx.doi.org/10.1016/j.gie.2009.03.651
- ShimizuTYamatoMIsoiYAkutsuTSetomaruTAbeKKikuchiAUmezuMOkanoT 2002 Circ. Res. 90 e40 http://dx.doi.org/10.1161/hh0302.105722
- HasegawaMYamatoMKikuchiAOkanoTIshikawaI 2005 Tissue Eng. 11 469 http://dx.doi.org/10.1089/ten.2005.11.469
- AkizukiTOdaSKomakiMTsuchiokaHKawakatsuNKikuchiAYamatoMOkanoTIshikawaI 2005 J. Periodont. Res. 40 245 http://dx.doi.org/10.1111/j.1600-0765.2005.00799.x
- IwataTYamatoMTsuchiokaHTakagiRMukobataSWashioKOkanoTIshikawaI 2009 Biomaterials 30 2716 http://dx.doi.org/10.1016/j.biomaterials.2009.01.032
- KanzakiM et al 2007 Biomaterials 28 4294 http://dx.doi.org/10.1016/j.biomaterials.2007.06.009
- MaedaMYamatoMKanzakiMIsekiHOkanoT 2009 J. Tissue Eng. Regenerative Med. 3 255 http://dx.doi.org/10.1002/term.161
- OhashiK et al 2007 Nat. Med. 13 880 http://dx.doi.org/10.1038/nm1576
- ShimizuHOhashiKUtohRIseKGotohMYamatoMOkanoT 2009 Biomaterials 30 5943 http://dx.doi.org/10.1016/j.biomaterials.2009.07.042
- ShiroyanagiYYamatoMYamazakiYTomaTOkanoT 2004 BJU Int. 93 1069 http://dx.doi.org/10.1111/j.1464-410X.2004.04783.x
- AoyagiTEbaraMSakaiKSakuraiYOkanoT 2000 J. Biomater. Sci., Polym. Ed. 11 101 http://dx.doi.org/10.1163/156856200743526
- EbaraMYamatoMHiroseMAoyagiTKikuchiASakaiKOkanoT 2003 Biomacromolecules 4 344 http://dx.doi.org/10.1021/bm025692t
- EbaraMYamatoMAoyagiTKikuchiASakaiKOkanoT 2004 Biomacromolecules 5 505 http://dx.doi.org/10.1021/bm0343601
- EbaraMYamatoMAoyagiTKikuchiASakaiKOkanoT 2004 Tissue Eng. 10 1125 http://dx.doi.org/10.1089/ten.2004.10.1125
- EbaraMYamatoMAoyagiTKikuchiASakaiKOkanoT 2008 Biomaterials 29 3650 http://dx.doi.org/10.1016/j.biomaterials.2008.05.030
- NishiM et al 2007 Biomaterials 28 5471 http://dx.doi.org/10.1016/j.biomaterials.2007.08.027
- EbaraMYamatoMAoyagiTKikuchiASakaiKOkanoT 2008 Adv. Mater. 10 3034 http://dx.doi.org/10.1002/adma.200702308
- BhatiaSYarmushMTonerM 1997 J. Biomed. Mater. Res. 34 189 http://dx.doi.org/10.1002/(SICI)1097-4636(199702)34:2<189::AID-JBM8>3.0.CO;2-M
- YousafM NHousemanB TMrksichM 2001 Proc. Natl Acad. Sci. USA 98 5992 http://dx.doi.org/10.1073/pnas.101112898
- KhetaniS RBhatiaS N 2008 Nat. Biotechnol. 26 120 http://dx.doi.org/10.1038/nbt1361
- AlbrechtD RUnderhillG HWassermannT BSahR LBhatiaS N 2006 Nat. Method. 3 369 http://dx.doi.org/10.1038/nmeth873
- TsangV LBhatiaS N 2004 Adv. Drug Deliv. Rev. 56 1635 http://dx.doi.org/10.1016/j.addr.2004.05.001
- CalvertP 2007 Science 318 208 http://dx.doi.org/10.1126/science.1144212
- TsudaYKikuchiAYamatoMNakaoASakuraiYUmezuMOkanoT 2005 Biomaterials 26 1885 http://dx.doi.org/10.1016/j.biomaterials.2004.06.005
- TsudaYKikuchiAYamatoMChenGOkanoT 2006 Biochem. Biophys. Res. Commun. 348 937 http://dx.doi.org/10.1016/j.bbrc.2006.07.138
- ItogaKYamatoMKobayashiJKikuchiAOkanoT 2004 Biomaterials 25 2047 http://dx.doi.org/10.1016/j.biomaterials.2003.08.052
- ItogaKKobayashiJTsudaYYamatoMOkanoT 2008 Anal. Chem. 80 1323 http://dx.doi.org/10.1021/ac702208d
- ItogaKYamatoMKobayashiJKikuchiAOkanoT 2004 J. Biomed. Mater. Res. A 69 391 http://dx.doi.org/10.1002/jbm.a.30010
- KobayashiJYamatoMItogaK KOkanoT 2004 Adv. Mater. 16 1997 http://dx.doi.org/10.1002/adma.200400312
- NieF-QYamadaMKobayashiJYamatoMKikuchiAOkanoT 2007 Biomaterials 28 4017 http://dx.doi.org/10.1016/j.biomaterials.2007.05.037
- WadaKTaniguchiAKobayashiJYamatoMOkanoT 2008 Biotechnol. Bioeng. 99 1513 http://dx.doi.org/10.1002/bit.21718
- TsudaYShimizuTYamatoMKikuchiASasagawaTSekiyaSKobayashiJChenGOkanoT 2007 Biomaterials 28 4939 http://dx.doi.org/10.1016/j.biomaterials.2007.08.002
- SekiyaSShimizuTYamatoMKikuchiAOkanoT 2006 Biochem. Biophys. Res. Commun. 341 573 http://dx.doi.org/10.1016/j.bbrc.2005.12.217