Abstract
Luminescent metal nanoclusters that consist of only several, to tens of, metal atoms and which possess sizes comparable to the Fermi wavelength of electrons have recently attracted significant attention. This new class of luminescent materials not only provides the missing link between atomic and nanoparticle behaviors in metals but also they present abundant novel information for the development of new applicable material systems to meet urgent needs in many areas (such as ultrasensitive sensors for heavy metals, bioimaging, as well as information technology) mainly because of their attractive characteristics, including ultra-small size, good dispersibility, excellent biocompatibility and photostability. In this review, we summarize recent advances in the controlled synthesis and application of luminescent metal nanoclusters, with a particular emphasis on Pt, Mo, Bi and alloy clusters. We also speculate on their future and discuss potential developments for their use in sensors, bioimaging and energy harvesting and conversion.
Introduction
Current research that tends to shrink the dimensions of materials are driven by the desire to access the unique material properties and performance advantages that occur in the transition to nanometer length scales [Citation1]. Among nanomaterials, the study of metal nanoparticles has a long history in terms of preparation, characterization and applications. Understanding the properties of noble metal nanoparticles and exploring their application potential are major driving forces behind the synthesis of a large variety of nanomaterials [Citation2]. Nanoscale metals are roughly classified into three size domains: large nanoparticles, small nanoparticles and nanoclusters (NCs), corresponding to their characteristic length scales [Citation3–Citation5]. When the particle size is comparable to, or even larger than, the wavelength of photons that interact with it, the optical responses of these large metal nanoparticles to external electromagnetic fields are simply dependent on their sizes, free-electron density and their nearly bulk-like dielectric function relative to that of the surrounding medium; therefore, they can be quantitatively described with the Mie theory [Citation3, Citation5]. When the particle size approaches the electron mean free path (∼ 50 nm for Au) [Citation3, Citation5], the dielectric function and refractive indices become strongly size-dependent [Citation2, Citation3, Citation5]. Generally, these two classes of metal nanostructures possess diameters larger than 2 nm. The third kind of particles have sizes comparable with the Fermi wavelength of an electron. The optical, electronic and chemical properties demonstrate large differences with respect to those in the other two size regimes. Nowadays, it is widely accepted that the particles with diameters larger than 2 nm are called nanoparticles, particles smaller than 2 and 1 nm are generally called NCs and sub-NCs, respectively [Citation6, Citation7]. In view of the similarity between NCs and sub-NCs in many regards (such as preparation approaches, structural features and luminescent behaviors), hereafter we will refer to clusters with sizes smaller than 2 nm as NCs. What is particularly attractive is that such molecule-like metal NCs, composed of a few to roughly a hundred atoms, usually show high-yield photoluminescence (PL), good photostability, large Stokes shift and high emission rates. In the early stage of the study of luminescent noble metal clusters, the photophysical behaviors were roughly predicted by the free-electron model, suggesting that the emission originates from intraband transitions of free electrons. In recent years, employing state-of-the-art quantum chemistry codes, relatively accurate prediction and explanation of the optical properties of a broad range of NCs have been realized [Citation8–Citation12]. A broad range of luminescent metal NCs composed of gold (Au) [Citation5–Citation7, Citation13–Citation27], silver (Ag) [Citation12, Citation25–Citation36], copper (Cu) [Citation25, Citation35, Citation37, Citation38], platinum (Pt) [Citation25, Citation39, Citation40], bismuth (Bi) [Citation11, Citation41–Citation45], molybdenum (Mo) [Citation46–Citation48] or mixed metals [Citation49–Citation51] have been successfully fabricated using diverse top-down or bottom-up approaches. Compared with other counterparts, Au and Ag NCs have attracted more attention and have been intensively studied over the past decade. In addition, functional applications in the areas of sensors and bioimaging have been demonstrated. For specialized information on the properties and applications of Au and Ag NCs, the reader is referred to several recent excellent reviews [Citation5–Citation7, Citation9, Citation31, Citation52, Citation53].
In this review, we focus on luminescent NCs composed of Pt, Mo, Bi and even more than one metal element and will compare their respective advantages and disadvantages. Given that the preparation approaches are strongly dependent on the cluster's type (i.e. one synthesis method for one kind of NCs may not be suitable for others), we summarize the preparation methods and properties of typical NCs according to their compositions. We will then describe the representative achievements regarding their prospective applications in sensors and bioimaging. Finally, we will discuss some of the key scientific challenges that researchers currently face and present our perspectives on future research trends in this exciting field of science.
Synthesis approaches for Pt, Mo, Bi and alloy NCs
Pt NCs
Pt is one of the most important metals in catalysis applications. Recent work has shown that Pt8–10 NCs stabilized on high-surface-area solid supports are 40–100 times more active for the oxidative dehydrogenation of propane than the previously studied Pt catalysts, which is believed to result from the surprisingly high surface reactivity of Pt NCs [Citation54]. In addition to the peculiar catalysis properties of Pt NCs, Kawasaki et al [Citation39] were the first to report that Pt NCs synthesized in N,N-dimethylformamide (DMF) solution display bright luminescence. Inspired by a DMF reduction method for Au clusters [Citation15], Kawasaki et al adopted this technique for Pt NCs by strictly controlling the temperature and stirring speed. In brief, a solution of 150 ml of 0.1 M aqueous H2PtCl6 was added to 15 ml of DMF that had been preheated to 140 °C. The DMF solution was then refluxed in a 140 °C oil bath with vigorous stirring for 8 h in air [Citation39]. As the reaction proceeded, the solution slowly changed in color, from light yellow to colorless over 0–1 h and, finally, to yellow by 2–6 h. The color changes can be easily monitored by eye or by UV–visible (UV–Vis) absorption spectroscopy (figure (a)). The reaction was nearly complete at about 8 h, and the resulting DMF solution of Pt NCs were found to be stable for at least 6 months when stored in the dark, neither precipitating nor changing in spectral properties. The photophysical properties of the as-synthesized NCs are particularly interesting. As shown in figure (b), their emission maximum depends on the excitation wavelength (figure (b)). Under UV excitation at 350 nm, the maximum emission wavelength is 484 nm. With visible excitation at 500 nm, the maximum emission wavelength is 544 nm. The PL of metal clusters generally shifts to short wavelengths as the cluster size decreases. The PL results of Pt NCs suggest that there should be more than one emitter in the sample or the emission originates from the electronic transitions from multiple excited states to the ground state. X-ray photoelectron spectroscopy (XPS) analysis of the dried Pt NCs revealed a peak indicative of Pt 4f7/2 at 72.8 eV, suggesting the presence of Pt NCs in a more reduced state rather than Pt compounds in a high valence, such as Pt(II)Cl42− (74.4 eV). Given the size of the NCs, the medium binding energy of Pt 4f7/2 originates from the Pt at the surface and in the core. Owing to the limited information available [Citation39], much effort is required to further elucidate the luminescent mechanism from these Pt NCs. This approach provides a novel route for preparing highly fluorescent Pt NCs. In particular, this approach combines the advantages of a facile, surfactant-free synthesis that has high variability in the introduction of functionalized ligands and good control over the cluster stability and physical properties [Citation15, Citation39]. Obviously, the dilute solution of H2PtCl6 in DMF can be progressively reduced at high temperatures to form Pt atomic clusters, where no further stabilizing agent (such as surfactant, polymer or thiolate-organic compounds) is needed. Thus, DMF is expected to be a weak reducing agent as well as stabilizing ligand for Pt NCs. Furthermore, matrix-assisted laser desorption/ionization mass spectrometry (MALDI-MS) was taken to gather more information of such luminescent Pt clusters after undergoing ligand exchange with 2-mercapto-benzothiazole (MBT). The MALDI-MS spectrum demonstrates the existence of sub-nanometer-sized Pt NCs of 4–6 Pt atoms, using MBT as a novel matrix (figure ). The mass of the dominant molecular ion observed in negative ion mode was consistent with Pt5(MBT)7 NCs, the simulated mass obtained in isotropic pattern analysis. This result indicates that the excitation-wavelength dependent emission stems from more than one Pt NC.
Figure 1 (a) UV–Vis spectra at different reaction times of t = 0 (H2PtCl6), 5 min, 1, 2, 3, 4, 5 and 6 h. (b) PL spectra of DMF-protected Pt NCs. Emission spectra for excitation at 300, 350, 400, 450 and 500 nm are shown. The inset photographs show Pt NCs under: (a) room light; and (b) 365 nm UV light. (Reprinted with permission from [Citation39], The Royal Society of Chemistry © 2010.)
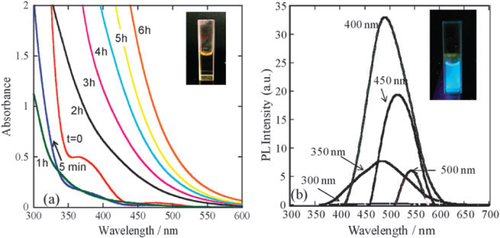
Figure 2 MALDI mass spectrum of MBT-protected Pt NCs in the negative ion mode, obtained for DMF-protected Pt NCs by ligand exchange with MBT. (Reprinted with permission from [Citation39], The Royal Society of Chemistry © 2010.)
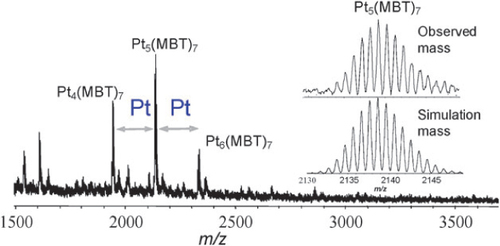
Generally, the reduction of metal ions in aqueous solutions results in large nanoparticles instead of small NCs due to their tendency to aggregate. In the case of DMF reduction approach, the stabilizing agent is DMF, which can easily be exchanged with other ligands for functionalization. Additionally, it has been found that the nature of the ligands used for capping the particle surface can markedly affect their emission properties [Citation55]. Therefore, choosing suitable agents capable of stabilizing clusters from aggregating and enhancing their fluorescence is of vital importance for obtaining small, highly fluorescent metal NCs [Citation7]. Because of the capability of sequestering metal ions from solution, dendrimers have been utilized as templates to prepare luminescent Pt NCs, as first reported by Tanaka et al [Citation40]. In brief, Pt NCs were prepared by reducing H2PtCl6 (6.0 ml, 0.5 m) with NaBH4 (3.0 mmol) in the presence of the fourth-generation polyamidoamine dendrimer (PAMAM (G4-OH)) (0.5 mmol, 71.4 mg). NaBH4was slowly added to the mixture under continuous stirring. To complete the reaction, the mixture was stirred for 2 weeks. Large Pt colloidal nanoparticles were removed by ultracentrifugation for 30 min at 4 °C. The supernatant emitted a strong blue fluorescence under UV light (365 nm) irradiation, indicating the formation of Pt NCs.
Recently, Yuan and co-workers reported a simple and scalable method for the synthesis of highly fluorescent Pt NCs based on a mild etching environment that was made possible by phase transfer through electrostatic interactions [Citation25]. A simple and fast phase transfer cycle has been developed to process originally polydisperse, nonfluorescent and unstable NCs into monodisperse, highly fluorescent and extremely stable NCs in the same phase (aqueous) and protected by the same thiol ligand. Basically, this method consists of three steps. First, original metal NCs in the aqueous phase were synthesized in the aqueous stock solutions of metal precursors with concentrations of 20 mM and aqueous solutions of thiol ligands. The second step is the transfer of the glutathione-protected metal NCs to an organic phase through the electrostatic interaction ((CTA)+(COO)−) between negatively charged carboxyl groups on the metal NC surface and the positively charged cation of a hydrophobic salt, cetyltrimethylammonium bromide (figure ) [Citation25]. This phase transfer takes place quickly upon mixing of the reagents. One of the most attractive features of this method is that the fluorescent metal NCs in the organic phase can easily be shuttled back to the aqueous phase. In the third step, upon the addition of hydrophobic salt in chloroform, tetramethylammonium decanoate, to the fluorescent metal NCs in toluene, the hydrophobic decanoate anion D− quickly forms a hydrophobic salt (CTA)+D− with the hydrophobic cation CTA+ on the glutathione-protected metal NCs. The removal of the hydrophobic cation CTA+ from the glutathione-protected metal NCs restores the negative charge on the metal NCs, enabling them to return back to the aqueous phase [Citation25]. As a consequence of the smart design of the chemical reaction, a fast and complete transfer of the fluorescent metal NCs from the organic to the aqueous phase was made possible. Note that the resulting NCs are different from those after the first step in many regards, such as dispersibility, stability and optical properties. This synthetic protocol can be applicable for the synthesis of other classes of highly fluorescent NCs, such as Au, Ag and Cu NCs.
Figure 3 A schematic illustration of the process to generate highly fluorescent metal NCs by a phase transfer cycle. (Reprinted with permission from [Citation25], The American Chemical Society © 2011.)
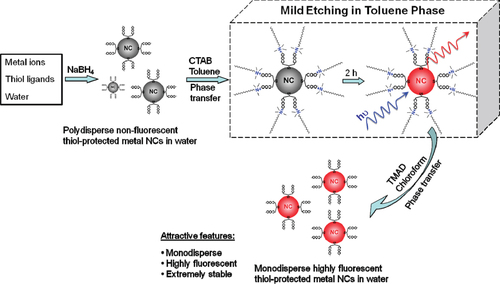
Mo NCs
A Mo element can form fascinating polynuclear structural units. Among these, compounds known as the Chevrel phases have been actively studied because they are type II superconductors with relatively high critical fields [Citation56]. Such materials are generally synthesized by high temperature (1100 °C) reactions of the chalcogen and Mo metal. Most of these materials can be described by the formula MMo6X8, where M = Pb, Sn, Ba, Au, Cu, Li, etc and X is usually S, Se or Te [Citation56]. Structurally related, soluble analogues have been prepared; for example, Mo6S8(PEt3)6 [Citation57]. The fundamental structural unit in the Chevrel phase is the cluster Mo6X8, which is shown in three different ways in figure . In figure (a), an octahedron of Mo atoms (Mo–Mo = 2.7 Å) is encased in a cube of chalcogens (Mo–S 2.45 or Mo–Se 2.6 Å). Figure (b) exhibits the same cluster as consisting of an octahedron with its triangular faces capped by chalcogens, this view emphasizes the connectivity within the cluster. In figure (c) the cluster has been reoriented so that a three-fold axis is vertical.
Figure 4 The structure of Mo6X8 (X is S, Se or Te). (Reprinted with permission from [Citation56], The American Chemical Society © 1983.)
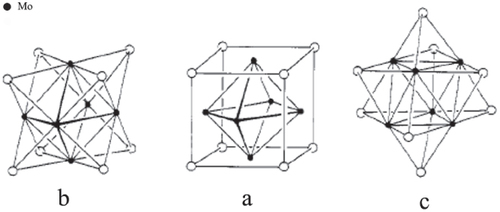
In addition to the stabilization of Mo subnanometer NCs by S, Se or Te in the Chevrel phase, it has well documented that Mo clusters can be well stabilized by halides. Although various reports of the chemistry of the compounds containing Mo have suggested that they were polynuclear, the first definite structural characterization was a crystallographic study of [Mo6Cl8](OH)4·14H2O [Citation58]. The Mo atoms are at the corners of a nearly regular octahedron of edge 2.63 Å while eight chlorine atoms are at the corners of a symmetrically circumscribed cube, such that the shortest Mo–Cl distance is 2.56 Å. Subsequently, Vaughan found that a more complex unit, [Mo6Cl14]2− ion, exists in (NH4)2Mo6Cl14·H2O [Citation59]. In idealized cubic symmetry, the [Mo6Cl14]2− consists of an octahedron of metal atoms surrounded by eight face-bridging and six axial halides. In 1981, Maverick and Gray [Citation60] first investigated the optical properties of [Mo6Cl14]2− ions and found that they are intensely luminescent. The emission demonstrates a large Stokes shift, the corrected peak wavelength is approximately 760 nm (figure ). Interestingly, the absorption and emission spectra for [Mo6Cl14]2− in acetonitrile and aqueous HCl solutions at room temperature do not show a large difference. The emission spectra are essentially identical for the two solutions, but they differ in intensity: estimated lower limits for emission quantum yields are 0.04 and 0.005 for the acetonitrile and hydrochloric acids solutions, respectively. Absorption spectra recorded for single crystals of (Bu4N)2Mo6Cl14 reveal weak shoulders at 530 and 590 nm. Maverick and Gray suggested that the 590 nm absorption is associated with the luminescent excited state.
Figure 5 Electronic spectra of [Mo6Cl14]2−. Absorption, (A) (NH4)2-Mo6Cl14 in 6 M aqueous HCl; (B) (Bu4N)2Mo6Cl14 in CH3CN. Emission, (C) (arbitrary units). The emission spectrum shown is uncorrected. In the corrected spectrum peak wavelength is at 760 nm. Similar absorption and emission spectra were observed for solid (Bu4N)2Mo6Cl14. (Reprinted with permission from [Citation60], The American Chemical Society © 1981.)
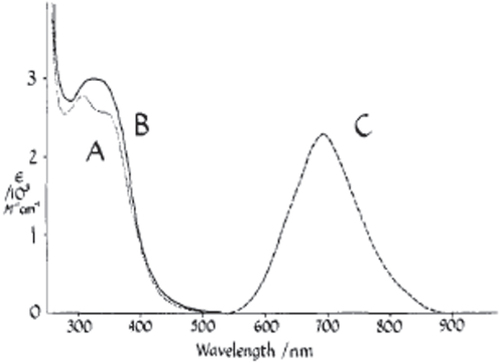
Stimulated by the peculiar electronic properties of Mo clusters, Maverick et al further synthesized a series of crystalline compounds containing Mo6 clusters. The cluster ions Mo6Cl142− and Mo6Br142− are luminescent, with emission maxima, lifetimes and quantum yields of 805, 180 μs, and 0.19 and 825, 130 μs, 0.23, respectively, in acetonitrile at room temperature [Citation61]. Since the energies of these bands are similar for Mo6Cl142− and Mo6Br142−, it is believed that both highest occupied and lowest unoccupied molecular orbitals are largely metal centered [Citation61]. Other researchers have revealed that Mo6 NCs can be integrated with other material systems, such as semiconducting quantum dots [Citation46], silica [Citation47] and copolymer [Citation48]. In an earlier effort, Grasset et al [Citation46] presented the preparation and characterization of a novel organosol and solid state nanocomposite, namely ((n-C4H9)4N)2Mo6Br14@ZnO, by using an original and simple bottom-up process. The hydrodynamic diameter of these particles is about 12 nm. PL spectroscopy was employed to determine whether the luminescence properties of either the Mo6 cluster or the ZnO were affected when the Mo6Br14 cluster core was mixed with the ZnO matrix (figure ). The steady-state and time-resolved PL results suggest that the emission between 500 and 600 nm stems from the trap state of ZnO, while that over 600 nm are mainly from Mo6Br14 cluster core.
Figure 6 (a) Excitation spectrum when monitored at 550 nm. (b) Emission spectra when the excitation wavelengths are at: (i) 380 nm; (ii) 390 nm; (iii) 400 nm; (iv) 405 nm; (v) 410 nm; and (vi) 440 nm. (Reprinted with permission from [Citation46], Wiley-VCH © 2008.)
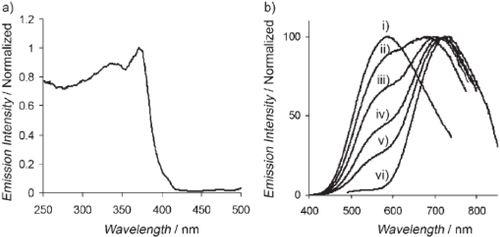
Recently, a simple method to obtain Mo6–PMMA copolymer samples within which Mo6 clusters keep their intrinsic luminescence properties has been reported [Citation48]. These emissive properties have been used successfully to sensitize Er3+ complex emission in the infrared region and within the polymer matrix. Aubert et al [Citation47] successfully stabilized the ZnO nanocrystals in the presence of water using polyvinylpyrrolidone for their further coencapsulation along with [Mo6Br14]2 cluster units in silica to form ZnO–Cs2[Mo6Br14]@SiO2 nanoparticles using a modified Stöber process. These nanoparticles exhibit tunable emission properties with a broad emission spectrum covering almost the entire visible range for an excitation wavelength of 365 nm (figure ). Importantly, the luminescence properties remain intact when these nanoparticles are dispersed in water. This advance paves the way for their application as biolabels.
Figure 7 Left: normalized emission spectra of ZnO–Cs2[Mo6Br14]@SiO2 nanoparticles depending on the excitation wavelength. Right: emission of ZnO–Cs2[Mo6Br14]@SiO2 nanoparticles dispersed in water under 365 nm excitation. (Reprinted with permission from [Citation47], Wiley-VCH © 2013.)
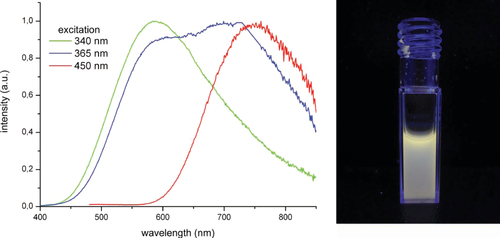
Bi NCs
Bi is one of the most thoroughly investigated main group elements and it exists in a wide array of functional materials such as magnets, superconductors, thermoelectric and spintronic materials [Citation44]. Basically, depending on the material systems, Bi displays two kinds of structural features. The first case is that Bi exists in functional materials in which it coordinates with other elements. In these compounds, Bi usually demonstrates diverse oxidation states such as +3 and +2. Interestingly, in some compounds such as molecular crystals containing Bi polycations and polyanions Bi can form molecule-like clusters (i.e. Bi coordinates with Bi element itself). The charges that such clusters possess can be balanced by other structural units such as [AlCl4]− or [K-crypt]+ (Crypt = 4,7,13,16,21,24-hexaoxa-1,10-diazabicyclo[8.8.8] hexacosane) [Citation41–Citation45]. Indeed, the study of such peculiar subnanometer Bi NCs dates back to half a century ago. In the 1960s, Smith and co-workers systematically studied the evolution of Bi oxidation states in molten salt systems [Citation62–Citation67]. Based on the thorough examination of spectroscopic properties of Bi-containing liquids, Smith and co-workers first proposed that the absorption bands in UV to near-infrared (NIR) spectral ranges stem from the electronic transitions of subvalent Bi (e.g. Bi+, Bi53+ or Bi82+) [Citation62–Citation67], although the detailed structural information of these subvalent species were not provided. Such pioneering work lead to more concentrated research on the synthesis and characterization of materials containing subvalent Bi in the following decades, and much clearer pictures on the oxidation states of Bi and new synthetic approaches have been reported by Ruck, Kloo and their co-workers [Citation68–Citation74]. These systematic studies suggest that a highly Lewis-acidic environment is a necessary prerequisite for the stabilization of Bi cationic clusters. Ruck et al studied the viability of Lewis-acid ionic liquids for the synthesis of low-valent Bi compounds. At room temperature, elemental Bi and Bi (III) cations synproportionate in the ionic liquid [BMIM]Cl/AlCl3 ([BMIM]+: 1-n-butyl-3-methylimidazolium) within minutes. The existence of Bi polycations in the dark colored solution was proven by Raman spectroscopy. Dark-red crystals of Bi5(AlCl4)3 were isolated from the ionic liquid [Citation75]. In subsequent studies, Sun et al [Citation42] found that Bi53+ and Bi+ emitters stabilized by the Lewis acidic liquid show ultrabroad PL with a lifetime of approximately 1 μs. Furthermore, it was found that Bi5(AlCl4)3 synthesized by this method exhibits extremely broad NIR PL with a full width at half maximum (FWHM) of >510 nm and an effective PL lifetime of 4.1 μs [Citation41]. This study greatly extends the understanding of photophysical properties of materials containing subvalent Bi, because of the establishment of structure–emitter–property relationships. Indeed, the infrared emission behaviors of material systems containing Bi are rather complicated, and in many cases there is no sound and definitely convincing explanation of emission mechanisms [Citation44]. This work, which uses the molecular crystals as model materials for the study of Bi-related emission mechanisms, represents a new and simple route to gaining deeper understanding of the photophysical behaviors of Bi doped systems. Subsequently, it was found that high-quality Bi5(AlCl4)3 crystal prepared by a modified approach displays a broadband emission band from 1 to 2.7 μm [Citation41, Citation44] (figure ). Interestingly, Bi53+ in Bi5(GaCl4)3 demonstrates a similar emission band under the excitation of 808 nm, although the exact structures of Bi53+ in both crystals are different (figure ).
Figure 8 PL spectra of Bi5(AlCl4)3 (a) and Bi5(GaCl4)3 (b) samples under 808 nm excitation. (Reproduced with permission from [Citation44], The Royal Society of Chemistry © 2012.)
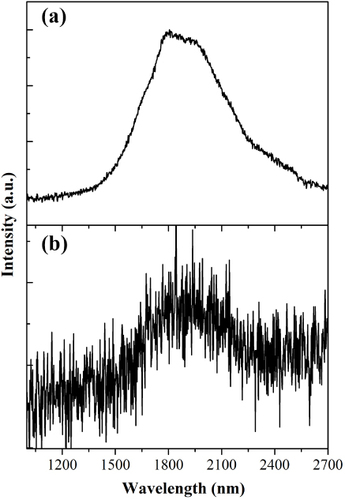
In addition to Bi53+, Sun et al [Citation43] experimentally and theoretically studied the photophysical properties of Bi82+ polycation stabilized by [AlCl4]−. As shown in figure (a), the final product, synthesized by a molten salt route, consists of Bi8(AlCl4)2 and Bi5(AlCl4)3 phases, as evidenced by powder x-ray diffraction (PXRD). It was reported that amorphous red phase, Bi5(AlCl4)3, usually forms when synthesizing Bi8(AlCl4)2 crystal [Citation76], which is in good agreement with the PXRD result. However, further detailed microscopic observation revealed that the product is dark black, and lacks a red phase, which suggests that the ratio of Bi5(AlCl4)3 to Bi8(AlCl4)2 is rather low [Citation43]. The Bi8(AlCl4)2 phase consists of Bi82+ polycations and tetrahedral [AlCl4]− anions (figure (b)). The [AlCl4]− ions render the compounds air- and moisture-sensitive because of the hydrolytic instability of Al–Cl bond.
Figure 9 (a) PXRD of the synthesized product after removal of the background. The vertical red and blue lines represent the diffraction peaks of Bi8(AlCl4)2 (CSD no: 405159) and Bi5(AlCl4)3 (CSD no: 420082) phases, respectively. (b) A general view of the structure of Bi8(AlCl4)2. (Reproduced with permission from [Citation43], The Royal Society of Chemistry © 2012.)
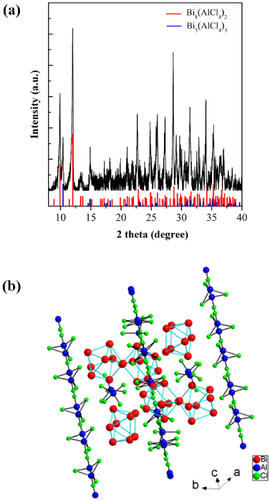
As displayed in figure , four peaks appear at the excitation/emission wavelengths of 290/1180, 490/1180, 636/1187 and 830/1192 nm, among which the intensity of the fourth peak is the strongest [Citation43]. It is obvious that the PL peak does not display significant excitation-wavelength-dependent shift, which situates it in the range of 1180–1200 nm under different excitation wavelengths. Further detailed analyses suggest that Bi82+ polycations contribute to emission peaking at about 1180 nm, while the emission tails at longer wavelengths results from Bi53+ [Citation43]. This work further evidences that Bi polycations can be smart emitters, whose emission can cover important telecommunication wavelengths.
Figure 10 Contour excitation-emission matrix plot of the obtained sample. Note that the jump at around 1400 nm results from measurement artifacts. (Reproduced with permission from [Citation43], The Royal Society of Chemistry © 2012.)
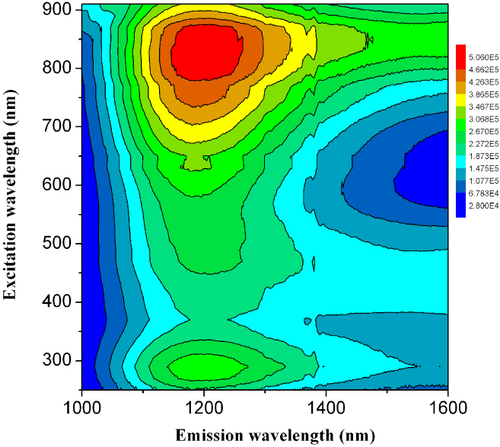
In addition to molecular crystals containing Bi polycations, it is noteworthy that many compounds with Bi polyanions absorb light in the visible range of the electromagnetic spectrum since they are colored, thus giving promise for unique optical properties [Citation45]. In 2012, Sun and coworkers first reported that a single crystal of (K-crypt)2Bi2 which contains [Bi2]2− polyanions displays ultrabroad NIR emission at around 1190 nm (figure ). As demonstrated in figure (b), the emission spectrum under 641 nm excitation has a very broad emission range, spanning from 975 to 1400 nm [Citation45]. The full width at half maximum (FWHM) of the spectrum is 212 nm and the emission peak is around 1190 nm. The emission spectrum by Gaussian fitting yields three components peaking at 1047, 1190 and 1331 nm with FWHMs of 99, 146 and 56 nm, respectively (figure (b)), whose emission energies are lower than the corresponding absorption energies at 990, 1090 and 1273 nm, respectively [Citation45]. A qualitative explanation of the observed photophysical behavior is proposed [Citation45]. The [Bi2]2− anion absorbs photons with energies in the NIR and visible ranges. After irradiation with high-energy photons, the electrons in the upper excited levels tend to nonradiatively relax to the first three excited levels from where the NIR emissions take place. That is, NIR PL results from the radiative electronic transitions from the first three excited levels to the ground level based on a one-photon process. The overlap of the three emission bands leads to the observed ultrabroad PL band ranging from 975 to 1400 nm. This result greatly deepens the understanding of Bi-related NIR emission behavior and leads to a reconsideration of the fundamentally important issue on Bi-related PL mechanisms in some material systems, such as bulk glasses, fibers and conventional optical crystals [Citation45].
Figure 11 (a) Part of the structure of (K-crypt)2Bi2. (b) The PL spectrum of (K-crypt)2Bi2 crystals under 641 nm excitation light. The black, red and green curves are experimental, fitted and three decomposed Gaussian peaks, respectively. (Reproduced with permission from [Citation45], The Royal Society of Chemistry © 2012.)
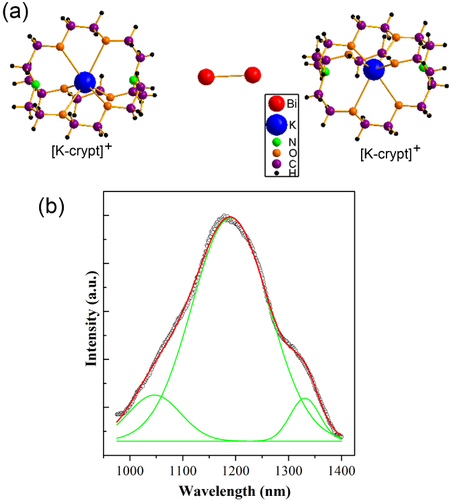
Additionally, the analysis of high-resolution synchrotron PXRD data coupled with steady-state and time-resolved PL spectra and time-dependent density functional theory (TDDFT) has found that the substructures of Bi+ residing in the sodalite cages of zeolites Y result in ultrabroad and tunable NIR PL [Citation11]. Subsequently, the structural and photophysical features of the resulting zeolite Y have been thoroughly characterized by using extensive experimental techniques, including nuclear magnetic resonance, electron paramagnetic resonance, two-dimensional excitation-emission and absorption spectra [Citation77]. A thorough analysis of luminescence and absorption spectra, coupled with TDDFT calculations, suggests that all Bi+ substructures (i.e. Bi44+, Bi33+, Bi22+ and Bi+) are optically active in the NIR spectral range. It is found that Bi+, Bi22+, Bi33+ and Bi44+ units result in NIR emissions peaking at about 1050, 1135, 1145, 1240/1285 nm, respectively [Citation77]. This finding represents an important contribution to the understanding of the processes involved in the formation of Bi+ and of the luminescence mechanisms of Bi+ substructures.
The dominant advantage of Bi NCs lies in their attractive infrared emission feature, which might lead to a broad range of applications in bioimaging, telecommunications and lasers. However, such NCs can only be stabilized by air-sensitive units or templates that can absorb water, resulting in the air instability of the final product. Furthermore, these NCs generally exist in crystalline matrices. It is greatly expected that monodisperse Bi NCs can be obtained for their practical applications. With enough effort in these directions, it is hopeful that air-stable, monodisperse and highly luminescent Bi NCs can be synthesized.
Alloy metal NCs
Atomically precise molecules of noble metals, such as Au, Ag, Pt and Cu with quantum confinement phenomena, have been some of the most fascinating materials in molecular cluster science during recent years [Citation5, Citation7, Citation9]. However, because PL from such NCs generally occurs only at some specific spectral ranges, the ability to tune these optoelectronic behaviors has been limited. Since the photophysical properties of NCs are closely correlated with their compositions and structures, there remains the possibility of manipulating their properties through precise variations in composition [Citation50]. This has stimulated the exploration of luminescent alloy NCs. A recent example of manipulation in alloy compositions is the creation of stable 13-atom AuAg alloy clusters [Citation49] (figure ). These alloy NCs are prepared from the Ag7,8 cluster (a mixture containing Ag7 and Ag8 NCs). Basically, synthesis of AuAg alloy NCs involves three steps. The first step is the synthesis of polydisperse Ag@H2MSA nanoparticles followed by the synthesis of Ag7,8 clusters by interfacial etching in the second step. In the third step, addition of an appropriate amount of 10 mm HAuCl4 to the as-synthesized Ag7,8 cluster yields the alloy cluster.
Figure 12 Changes observed during synthesis. (A,B) Solutions of Ag7,8 (A) and the alloy NC (B) after synthesis under visible light. Insets: the same samples under UV light. (C) UV–Vis profile of (a) Ag7,8 and (b) Alloy NC measured in water; arrows indicate the well-defined optical features of the cluster. (D) Luminescence spectra of (a) Ag7,8 and (b) alloy NC in water at 300 K. (E) Photographs of (a, a1) alloy NC in water and (b, b1) in the solid state under visible and UV light. (F) Comparison of the polyacrylamide gel electrophoresis results of (a, a1) Ag8 and (b, b1) alloy NC. Photographs of gel in visible (a, b) and UV (a1, b1) light. Band positions are marked with circles on the gels. (Reproduced with permission from [Citation49], Wiley-VCH © 2012.).
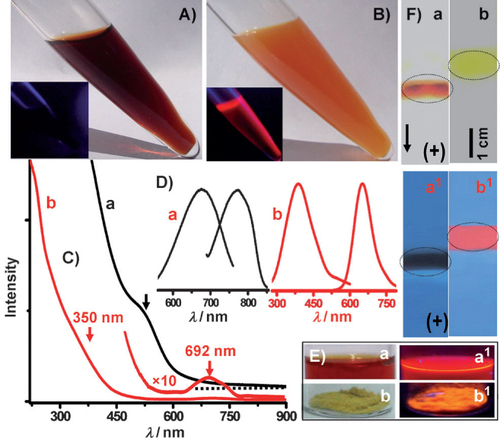
In a subsequent work, Mohanty et al [Citation50] further synthesized a series of alloy NCs with tunable compositions in a bovine serum albumin (BSA) template starting from Au and Ag clusters, either through NC–NC interaction or galvanic exchange reaction. The existence of the alloy is obviously confirmed using elemental analysis by XPS and scanning electron microscopy and energy dispersive spectroscopy (SEM-EDS). The mass spectra of the mixture of clusters show well-defined compositions with complete tenability (figure ), suggesting the formation of Au1−xAgx clusters across the entire compositional window.
Figure 13 MALDI MS data shows the tunability of the composition of the Au–Ag alloy clusters in protein templates. MS of native BSA (violet) and BSA at pH 12 (black). The products of 900 ml AgNC@BSA + 100 μl AuNC@BSA(90:10) peak at m/z 71 100 (orange trace), 500 ml AgNC@BSA + 500 μl AuNC@BSA(50:50) peak at m/z 72 300 (blue trace) and 100 ml AgNC@BSA + 900 ml AuNC@BSA(10:90) peak at m/z 72 800 (olive trace) lie between AgNC (red trace) and AuNC@BSA (magenta trace), suggesting compositional variation in the alloys. Inset photographs: (a) AgNC@BSA; (b) AuNC:AgNC@BSA(10:90); (c) 50:50AuNC: AgNC; (d) AuNC: AgNC(90:10) and (e) AuNC@BSA under visible light (left) and UV light (right). The ratio mentioned here is v/v, which can also be considered as a molar ratio since Au and Ag solutions of the same molarity were used. (Reproduced with permission from [Citation50], The Royal Society of Chemistry © 2012.)
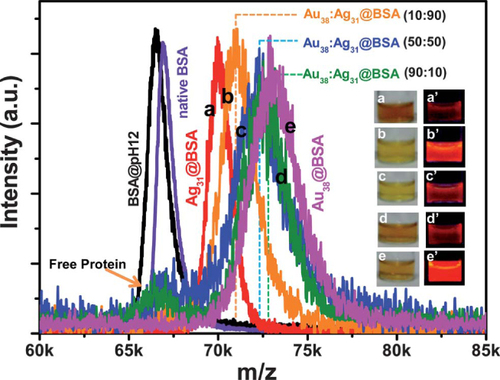
In addition to alloy NCs with noble metals, synthesis of luminescent NCs consisting of cheap metals not only presents a route for low-cost applicable materials but also might give rise to new opportunities for the understanding of the formation mechanisms of alloy NCs. In this regard, Andolina et al [Citation51] reported the synthesis of discrete, bimetallic Au–Cu NC alloys with diameters of 2–3 nm which display PL that can be tuned by changing the alloy composition. Upon varying the composition of the NCs from 0 to 100% molar ratio Cu, the PL maxima shift from 947 to 1067 nm under the excitation of 360 nm (figure ). The resulting particles exhibit brightness values that are more than an order of magnitude larger than the brightest NIR-emitting lanthanide complexes and small-molecule probes evaluated under similar conditions. The PL from these alloy NCs is very intriguing, given that the emission can extend to the NIR spectral range. These novel NCs may become a promising class of stable and tunable NIR nanoprobes that can be readily translated into biological settings if they possess excellent photostability and low cytotoxicity.
Figure 14 (A) Normalized and offset emission spectra of AuxCuy NCs, excitation at 360 nm. (B) Average emission wavelength as a function of the initial molar ratio of Cu. (Reproduced with permission from [Citation51], The American Chemical Society © 2013.)
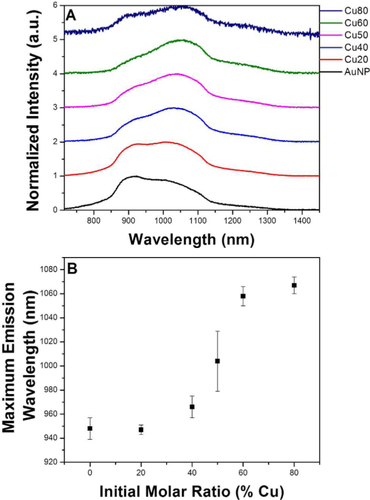
Although alloy NCs possess attractive photophysical properties and can be synthesized by well-controlled approaches, there are still a number of scientific issues that require to be answered (such as the detailed coordination environments of metal species in alloys, the long-term physiochemical stability and the exact PL mechanisms of such alloy NCs). These issues will hopefully be solved when using other powerful analytic techniques, such as x-ray crystallography and time-resolved x-ray absorption spectroscopy.
Functional applications
Bioimaging
Fluorescence imaging offers unique advantages over other imaging methods in regard to sensitivity, multiplex detection capabilities and equipment cost [Citation7]. Until now, a wide array of novel nanomaterials including quantum dots, organic dyes and dye-doped or undoped inorganic NPs have been developed for bioimaging [Citation78, Citation79]. For example, quantum dots have been successfully used as in vivo cancer-targeted imaging agents of living animals [Citation80]. However, most of quantum dots contain toxic elements (such as cadmium and lead) and organic dyes are easy to be photobleached, which makes them rather difficult for in vivo imaging in humans [Citation79]. In contrast, fluorescent metal NCs are smaller and exhibit bright and stable emission, and good biocompatibility, making them attractive alternatives as fluorescent probes for bioimaging. Interestingly, the emission from various metal NCs covers not only the visible spectral range but also the NIR biological window, which implies that NCs are suitable for both cell and in vivo imaging.
A number of papers have reported biological imaging applications based on fluorescent metal NCs. Tanaka et al [Citation40] investigated the capability of Pt5 NCs to act as fluorescent probes for live cell imaging. First, the Pt5(MAA)8 (MAA = mercaptoacetic acid) is conjugated with protein A using a 1-[(3-dimethylamino)-propyl]-3-ethylcarbodiimide hydrochloride/N-hydroxysulfosuccinimide coupling reaction. Next, Pt5(MAA)8–(protein A) is bound to an antichemokine receptor antibody through the Fc moiety of the antibody (figure ) [Citation40]. After the introduction of Pt5(MAA)8–(protein A)-(anti-CXCR4-Ab) into HeLa cells, the HeLa cells were incubated for 5 min prior to cell imaging. Figure (a) shows a confocal fluorescence image of HeLa cells labeled with Pt5(MAA)8 NCs. A blue fluorescence signal was clearly observed on cell membranes and no fluorescence signal was detected in a control sample labeled without Pt5(MAA)8 (figure (b)). Interestingly, the resulting NCs demonstrate the specific binding to the chemokine receptor [Citation40]. As shown in figure (c), no fluorescence signal was observed, indicating that CHOK1 cells were not stained by Pt5(MAA)8. Furthermore, the Pt5 NCs display low cytotoxicity [Citation40]. Figure (d) shows the viability of cells labeled with three different concentrations of Pt5 NCs. After 12 h incubation, more than 89% of the cells were alive and even after 48 h incubation cell viability was still more than 85%. These viabilities were comparable to controls [Citation40]. This suggests that Pt5 NCs are harmless fluorescent probes that are applicable for the long-term imaging of living cells.
Figure 15 Preparation of Pt5(MAA)8–(protein A)-(anti-CXCR4-Ab). (Reproduced with permission from [Citation40], Wiley-VCH © 2011.)
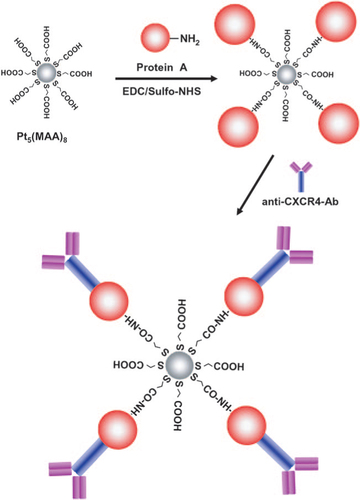
Figure 16 Confocal fluorescence microscopic images merged with differential interference contrast (DIC) images of living HeLa cells labeled with (a) and without (b) Pt5(MAA)8–(protein A)-(anti-CXCR4-Ab). (c) Confocal fluorescence microscopic images merged with DIC images of living CHO-K1 cells in the presence of Pt5(MAA)8–(protein A)-(anti-CXCR4-Ab). The scale bars are 20 μm. (d) Cell viability of HeLa cells. Cells were incubated with 1, 10 and 100 nm Pt5 NCs at 37 °C. The black bars show the cell viability of control samples in the absence of Pt5 NCs. (Reproduced with permission from [Citation40], Wiley-VCH © 2011.)
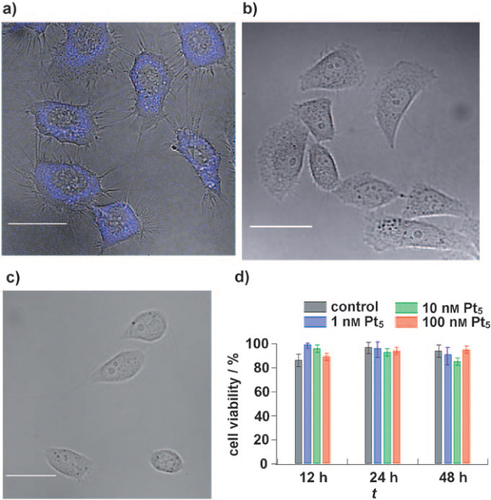
Recently, Zheng et al [Citation22] found that the renal clearance of 2 nm glutathione-coated luminescent Au NCs was more than 10–100 times better than that of similarly sized non-luminescent Au NPs. The small size in combination with surface ligands not only enables the majority of the luminescent Au NCs to be cleared from the body through kidney filtration but also stabilizes the luminescent Au NCs during blood circulation [Citation7, Citation22]. It is believed that the approach demonstrated by Zheng et al might be suitable for the improvement of the biological compatibility of other classes of NCs consisting of the element of Pt, Mo or Au/Ag, rendering them promising contrast imaging agents for cell and in vivo fluorescence imaging.
Sensors
The ions of heavy metals (such as mercury, lead and cadmium) are highly toxic pollutants to the environment. The accumulation of heavy metal ions in the body through food chains can ultimately induce various diseases [Citation6]. For example, Hg2+ is a highly toxic and widespread pollutant ion, and its damaging effects to the brain, nervous system and the kidney even at very low concentrations are well known [Citation81]. Ying et al [Citation82] demonstrated that BSA-stabilized Au NCs is rather sensitive for the detection of Hg2+. The sensing mechanism was based on the quenching of luminescence of Au NCs because of high-affinity metallophilic Hg2+–Au+ interactions (figure (a)). Upon adding Hg2+ ions (50 μM) to an aqueous Au NCs solution (20 μM), the red fluorescence of Au NCs was completely quenched within seconds (figure (b)). The red fluorescence of Au NCs could be partially recovered by adding a strong reductant (e.g. sodium borohydride) to Au NCs solution in the presence of Hg2+ ions [Citation82]. Furthermore, BSA-stabilized Au NCs display high specificity toward the detection of Hg2+ over other environmentally relevant metal ions owing to the high specificity of Hg2+–Au+ interactions. Figure (c) shows that the fluorescence of Au NCs was not quenched by 50 mM of Ag+, Cu2+, Zn2+, Mg2+, K+, Na+, Ni2+, Mn2+, Fe3+, Cd2+, Pt4+, Pd2+, Co2+, Pb2+ and Ca2+ ions [Citation82]. Only Hg2+ ions led to almost 100% quenching of Au NC fluorescence (figure (d)). The estimated detection limit for Hg2+ ions is 0.5 nM, which is much lower than the maximum level of mercury in drinking water (10 nM) permitted by the US Environmental Protection Agency (EPA).
Figure 17 (a) Schematic representation of Hg2+ sensing based on the fluorescence quenching of Au NCs resulting from high-affinity metallophilic Hg2+–Au+ bonds. (b) Photoemission spectra (ex. wavelength is 470 nm) and (inset) photographs under UV light (354 nm) of Au NCs (20 mM) in the (1) absence and (2) presence of Hg2+ ions (50 mM). (c) Photographs under UV light and (d) relative fluorescence (I/I0) under 470 nm excitation of aqueous Au NC solutions (20 mM) in the presence of 50 mM of various metal ions. (Reproduced with permission from [Citation82], The Royal Society of Chemistry © 2010.)
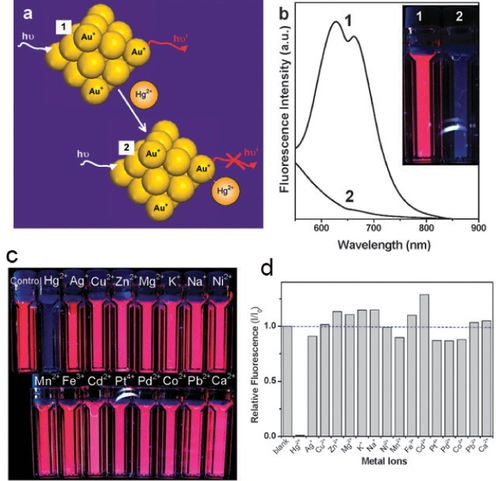
Lead ions (Pb2+) are a highly toxic environmental pollutant that can cause damage to the kidney, the liver and the nervous system and pose severe effects on human health [Citation83, Citation84]. The US EPA sets the maximum contamination level for lead in drinking water at 15 μg l−1 or 75 nM. Recently, Yuan et al [Citation85] developed a novel strategy to prepare functionalized fluorescent Au NCs based on a modified ligand exchange reaction and demonstrated the capability of glutathione functionalized Au NCs for ultrasensitive and selective Pb2+ sensing. The relative intensity reduction shows a nice linear relationship with Pb2+ concentration ranging from 5 nM to 5 mM. The limit of detection is determined to be 2 nM with a signal-to-noise ratio of 3.
The work by Ying et al [Citation82] and Yuan et al [Citation85] has clearly revealed that luminescent NCs can be exploited as sensors for toxic ions in the environment. It is noted that the functionality of such NCs is closely correlated with the ligands used for their stabilization. Given that the study of luminescent NCs is at the research stage and the more novel systems (e.g. alloy clusters) will be found in the near future, the employment of NCs as sensors for toxic ions has a bright future. By using environmentally friendly NCs with higher emission yields it is possible to develop more sensitive sensors for various toxic ions.
Conclusions and perspective
In this review, we have summarized the recent progress in the controlled synthesis and application of luminescent metal NCs, with a particular emphasis on Pt, Mo, Bi and alloy clusters. Compared with widely studied noble metal NCs, Pt, Mo, Bi and alloy NCs have received relatively less attention, and many works are still at the research stage. The abundant photophysical and physiochemical properties of NCs help researchers in this domain to deepen their understanding of the rationality of synthesis approaches, as well as the inherent interaction mechanisms with other species (e.g. when it is used as a sensor for toxic elements). However, a number of challenges remain in this exciting field of science.
Firstly, more effective synthesis protocols are greatly needed for the synthesis of highly luminescent and well-dispersed Pt, Mo, Bi and alloy clusters. In particular, most of the above-mentioned metal NCs possess a relatively weak brightness in comparison to semiconducting quantum dots and many organic dyes. Furthermore, it is noteworthy that some NCs (such as Mo and Bi NCs) are stable only in suitable matrices. Facing this, it is believed that developing revolutionary approaches for their synthesis is important for their broader application.
Secondly, more work should be done in the near future to establish relationships between the structure and emission properties. It is found that in many cases the resulting NCs are poly-disperse and display broad emission bands. Basically, there are two possibilities for this phenomenon: the emission is either from emitters with one kind of size or from those with diverse sizes. Furthermore, the photophysical properties of NCs are dependent on the protection ligands. To convincingly explain the observed luminescence from NCs, structural analyses of the obtained systems by x-ray crystallography are required, although in many cases it is difficult to culture high-quality single crystals of tiny NCs.
Thirdly, the emission and excitation ranges should be optimized. Most NCs emit in the spectral range <1000 nm. It is anticipated that the spectral range can be further extended into NIR or even mid-infrared, given them potential for both telecommunications and bioimaging. Furthermore, much effort is needed to build optical devices based on such NCs, which might find some commercial applications. Furthermore, most reported NCs have a UV excitation band and large-Stokes shifts occur, resulting in visible or NIR emissions. Indeed, this is not favorable for bioimaging, especially for in vivo imaging, owing to the weak penetration of UV light in living tissues. To solve this difficulty, one should develop NCs that exhibit NIR-excitable NIR emission.
In summary, given their low-cost, ready scalability, excellent chemical stability, colloidal stability and photostability, fluorescent metal NCs have shown primary potential in optical imaging and related disciplines. With further systematic experimental and theoretical studies, it is believed that these NC materials will find broad applications in addressing important issues related to the environment, medicine, diagnosis, telecommunications, and laser science.
Acknowledgments
HTS thanks the funding support from Soochow University, China and a project funded by the Priority Academic Program Development of Jiangsu Higher Education Institutions. The authors thank corresponding publishers for the kind permissions to reproduce their materials, especially figures, used in this review.
References
- GraingerD WCastnerD G 2008 Adv. Mater. 20 867 10.1002/adma.200701760
- SauT KRogachA LJäckelFKlarT AFeldmannC 2010 Adv. Mater. 22 1805 10.1002/adma.200902557
- KreibigUVollmerM 1995 Optical Properties of Metal Clusters Berlin Springer
- AshcroftN WMerminN D 1976 Solid State Physics New York Holt, Rinehart and Winston
- ZhengJNicovichP RDicksonR M 2007 Annu. Rev. Phys. Chem. 58 409 10.1146/annurev.physchem.58.032806.104546
- LuY ZChenW 2012 Chem. Soc. Rev. 41 3594 10.1039/c2cs15325d
- ShangLDongS JNienhausG U 2011 Nano Today 6 401 10.1016/j.nantod.2011.06.004
- ZhuM ZAikensC MHollanderF JSchatzG CJinR C 2008 J. Am. Chem. Soc. 130 5883 10.1021/ja801173r
- JinR C 2010 Nanoscale 2 343 10.1039/b9nr00160c
- PeiYPalRLiuC YGaoYZhangZ HZengX C 2012 J. Am. Chem. Soc. 134 3015 10.1021/ja208559y
- SunH TMatsushitaYSakkaYShirahataYTanakaMKatsuyaYGaoHKobayashiK 2012 J. Am. Chem. Soc. 134 2918 10.1021/ja211426b
- BakrO MAmendolaVAikensC MWenseleersWLiRNegroL DSchatzG CStellacciF 2009 Angew. Chem. Int. Edn Engl. 48 5921 10.1002/anie.200900298
- ZhengJPettyP TDicksonR M 2003 J. Am. Chem. Soc. 125 7780 10.1021/ja035473v
- ZhengJZhangC WDicksonR M 2004 Phys. Rev. Lett. 93 077402 10.1103/PhysRevLett.93.077402
- LiuX FLiC HXuJ LLvJZhuMGuoY BCuiSLiuH BWangSLiY L 2008 J. Phys. Chem. C 112 10778 10.1021/jp8028227
- XieJ PZhengY GYingJ Y 2009 J. Am. Chem. Soc. 131 888 10.1021/ja806804u
- YangXShiM MZhouR JChenX QChenH Z 2011 Nanoscale 3 2596 10.1039/c1nr10287g
- SunC J 2011 J. Am. Chem. Soc. 133 8617 10.1021/ja200746p
- HuangXLiB YLiLZhangHMajeedIHussainITanB N 2012 J. Phys. Chem. C 114 448 10.1021/jp209662n
- ShangLDorlichR MBrandholtSSchneiderRTrouilletVBrunsMGerthsenDNienhausG U 2011 Nanoscale 3 2009 10.1039/c0nr00947d
- WangH H 2011 ACS Nano 5 4337 10.1021/nn102752a
- ZhouCLongMQinY PSunX KZhengJ 2011 Angew. Chem. Int. Edn Engl. 50 3168 10.1002/anie.201007321
- KawasakiHHamaguchiKOsakaIArakawaR 2011 Adv. Funct. Mater. 21 3508 10.1002/adfm.201100886
- ShangLYangLStockmarFPopescuRTrouilletVBrunsMGerthsenDNienhausG 2012 Nanoscale 4 4155 10.1039/c2nr30219e
- YuanXLuoZZhangQZhangXZhengYLeeJXieJ 2011 ACS Nano 5 8800 10.1021/nn202860s
- WangZ JWuL NCaiWJiangZ H 2012 J. Mater. Chem. 22 3632 10.1039/c2jm15103k
- WangZ JCaiWSuiJ H 2009 ChemPhysChem 10 2012 10.1002/cphc.200900067
- KimYSeffK 1977 J. Am. Chem. Soc. 99 7055 10.1021/ja00463a047
- ZhengJPettyP TDicksonR M 2002 J. Am. Chem. Soc. 124 13982 10.1021/ja028282l
- ShangLDongS J 2008 Chem. Commun. 2008 1088
- ChoiSDicksonR MYuJ H 2012 Chem. Soc. Rev. 41 1867 10.1039/c1cs15226b
- PettyJ TFanC YStoryS PSenguptaBIyerA S JPrudowskyZDicksonR M 2010 J. Phys. Chem. Lett. 1 2524 10.1021/jz100817z
- O'NeillP RYoungKSchiffelsDFygensonD K 2012 Nano Lett. 12 5464 10.1021/nl3017797
- MuhammedM A HAldeekFPaluiGTrapiella-AlfonsoLMattoussiH 2012 ACS Nano 6 8950 10.1021/nn302954n
- ZhangHHuangXLiLZhangG WHussainILiZTanB N 2012 Chem. Commun. 48 567 10.1039/c1cc16088e
- YangXGanL FHanLWangE KWangJ 2013 Angew. Chem. Int. Edn Engl. 52 2022 10.1002/anie.201205929
- KawasakiHKosakaYMyoujinYNarushimaTYonezawaTArakawaR 2011 Chem. Commun. 47 7740 10.1039/c1cc12346g
- ChenJ HLiuJFangZ YZengL W 2012 Chem. Commun. 48 1057 10.1039/c2cc16668b
- KawasakiHYamamotoHFujimoriHArakawaRInadaMIwasakiY 2010 Chem. Commun. 46 3759 10.1039/b925117k
- TanakaS IMiyazakiJTiwariD KJinTInouyeY 2011 Angew. Chem. Int. Edn Engl. 50 431 10.1002/anie.201004907
- SunHSakkaYGaoHMiwaYFujiiMShirahataNBaiZLiJ 2011 J. Mater. Chem. 21 4060 10.1039/c1jm10164a
- SunHSakkaYFujiiMShirahataNGaoH 2011 Opt. Lett. 36 100 10.1364/OL.36.000100
- SunHSakkaYShirahataNGaoHYonezawaT 2012 J. Mater. Chem. 22 12837 10.1039/c2jm30251a
- SunHXuBYonezawaTSakkaYShirahataNFujiiMQiuJGaoH 2012 Dalton Trans. 41 11055 10.1039/c2dt31167d
- SunHYonezawaTGillett-KunnathM MSakkaYShirahataNGuiSFujiiMSevovS C 2012 J. Mater. Chem. 22 20175 10.1039/c2jm34101h
- GrassetFMolardYCordierSDorsonFMortierMPerrinCGuilloux-ViryMSasakiTHanedaH 2008 Adv. Mater. 20 1710 10.1002/adma.200701845
- AubertTNerambourgNSaitoNHanedaHOhashiNMortierMCordierSGrassetF 2013 Part. Syst. Charact. 30 90 10.1002/ppsc.201200047
- MolardYLabbéCCardinJCordierS 2013 Adv. Funct. Mater. 23 4821 10.1002/adfm.201300417
- UdayabhaskararaoTSunYGoswamiNPalS KBalasubramanianKPradeepT 2012 Angew. Chem. Int. Edn Engl. 51 2155 10.1002/anie.201107696
- MohantyJ SXavierP LChaudhariKBootharajuM SGoswamiNPalS KPradeepT 2012 Nanoscale 4 4255 10.1039/c2nr30729d
- AndolinaC MDewarA CSmithA MMarbellaL EHartmannM JMillstoneJ E 2013 J. Am. Chem. Soc. 135 5266 10.1021/ja400569u
- YuYYaoQ FLuoZ TYuanXLeeJ YXieJ P 2013 Nanoscale 5 4606 10.1039/c3nr00464c
- YuanXLuoZ TYuYYaoQ FXieJ P 2013 Chem. Asian J 8 858 10.1002/asia.201201236
- VajdaS 2009 Nature Mater. 8 213 10.1038/nmat2384
- WuZ KJinR C 2010 Nano Lett. 10 2568 10.1021/nl101225f
- HughbanksTHoffmannR 1983 J. Am. Chem. Soc. 105 1150 10.1021/ja00343a014
- SaitoTImotoH 1996 Bull. Chem. Soc. Japan 69 2403 10.1246/bcsj.69.2403
- BrossetC 1945 Ark. Kemi. Mineral. Geol. 20A 1
- VaughanP A 1950 Proc. Natl Acad. Sci. USA 36 461 10.1073/pnas.36.9.461
- MaverickA WGrayH B 1981 J. Am. Chem. Soc. 103 1298 10.1021/ja00395a088
- MaverickA WNajdzionekJ SMacKenzieDNoceraD GGrayH B 1983 J. Am. Chem. Soc. 105 1878 10.1021/ja00345a034
- DavisH LBjerrumN JSmithJ P 1967 Inorg. Chem. 6 1172 10.1021/ic50052a023
- BjerrumN JSmithJ P 1967 Inorg. Chem. 6 1968 10.1021/ic50057a005
- BjerrumN JDavisH LSmithJ P 1967 Inorg. Chem. 6 1603 10.1021/ic50054a045
- BjerrumN JBostonC RSmithJ P 1967 Inorg. Chem. 6 1162 10.1021/ic50052a022
- BostonC RSmithG P 1962 J. Phys. Chem. 66 1178 10.1021/j100812a047
- BostonC RSmithG PHowickL C 1963 J. Phys. Chem. 67 1849 10.1021/j100803a026
- RuckM 2001 Angew. Chem. Int. Edn Engl. 40 1182 10.1002/1521-3773(20010401)40:7%3C1182::AID-ANIE1182%3E3.0.CO;2-B
- RuckM 1997 Angew. Chem. Int. Edn Engl. 36 1971 10.1002/anie.199719711
- RuckM 1998 Z. Anorg. Allg. Chem. 624 521 10.1002/(SICI)1521-3749(199803)624:3%3C521::AID-ZAAC521%3E3.0.CO;2-M
- RuckMHampelS 2002 Polyhedron 21 651 10.1016/S0277-5387(01)01025-7
- RuckMDubenskyyVSöhnelT 2003 Angew. Chem. Int. Edn Engl. 42 2978 10.1002/anie.200250801
- DubenskyyVRuckM 2004 Z. Anorg. Allg. Chem. 630 2458 10.1002/zaac.200400206
- WahlBKlooLRuckM 2008 Angew. Chem. Int. Edn Engl. 47 3932 10.1002/anie.200800142
- AhmedEKöhlerDRuckM 2009 Z. Anorg. Allg. Chem. 635 297 10.1002/zaac.200800302
- BeckJBrendelCBengtsson-KlooLKrebsBMnmmertMStankowskiAUlvenlundS 1996 Chem. Ber. 129 1219 10.1002/cber.19961291013
- SunH TSakkaYShirahataNMatsushitaYDeguchiKShimizuT 2013 J. Phys. Chem. C 117 6399 10.1021/jp401861c
- FrangioniJ V 2003 Curr. Opin. Chem. Biol. 7 626 10.1016/j.cbpa.2003.08.007
- SunH 2011 Small 7 199 10.1002/smll.201001011
- GaoX HCuiYLevensonR MChungLNieS M 2004 Nature Biotechnol. 22 969 10.1038/nbt994
- HolmesPJamesK A FLevyL S 2009 Sci. Total Environ. 408 171 10.1016/j.scitotenv.2009.09.043
- XieJ PZhengY GYingJ Y 2010 Chem. Commun. 46 961 10.1039/b920748a
- ArakiSSatoHYokoyamaKMurataK 2000 Am. J. Ind. Med. 37 193 10.1002/(SICI)1097-0274(200002)37:2%3C193::AID-AJIM5%3E3.0.CO;2-J
- NeedlemanH 2004 Annu. Rev. Med. 55 209 10.1146/annurev.med.55.091902.103653
- YuanZ QPengM HHeYYeungE S 2011 Chem. Commun. 47 11981 10.1039/c1cc14872a