Abstract
Field and soil column experiments were conducted to analyze the effects of N uptake and transpiration by corn on nitrate–nitrogen (NO3-N) leaching in a granitic regosol and to evaluate the contribution of plant growth to the reduction of NO3-N leaching. In the field experiment, NO3-N leaching, N uptake by corn and retained N (inorganic N and microbial biomass N) were monitored in conventional planting density (CPD), high planting density (HPD) and no planting (NP) treatments. Nitrogen (N) was applied as (NH4)2SO4 at the rate of 300 kg N ha−1 and corn (Zea mays L.) was sown. In the soil column experiment, 1,500 mg N per column was applied and corn was sown in four treatments: no plants (NP), 1-plant, 2-plants and 4-plants per column. NO3-N leaching, N uptake and transpiration by corn and retained NO3-N in the soil were measured. In the field experiment, cumulative NO3-N leaching in the NP treatment was 208 kg N ha−1 from 38 to 49 days after treatment. In the CPD and HPD treatments, NO3-N leaching was reduced to 148 kg N ha−1 and 73 kg N ha−1, respectively. Nitrogen uptake by corn and retained N in the soil increased with increasing plant density. Cumulative NO3-N leaching was negatively correlated with N uptake by corn (r = −0.940, P < 0.01, n = 10). NO3-N leaching decreased as N uptake by corn increased above 60 kg N ha−1. In the soil column experiment, cumulative NO3-N leaching decreased with increased planting density because of increased N uptake by corn and the amount of retained NO3-N in the soil. The amount of retained NO3-N in the soil was positively correlated with transpiration by corn (r = 0.943, P < 0.01, n = 12). We concluded that NO3-N leaching from a granitic regosol during the rainy season could be reduced by increasing the planting density because of the increase in N uptake by plants and the increase of retained N in the soil derived from the increase in transpiration by plants.
INTRODUCTION
To ensure crop yield or as a result of tradition more N fertilizer than necessary is applied to crops. Excess fertilized N is transformed into nitrate and is easily leached from arable soil to pollute rivers and marine and groundwater resources (CitationAndraski et al. 2000; CitationThomsen et al. 1993; Citationvan der Ploeg et al. 1997). This nitrate–nitrogen (NO3-N) leaching from arable soil is a worldwide concern. If rainfall is high in the period following harvesting of the main crop, as is the case in Europe, the use of cover crops is effective for reducing NO3-N leaching. Cover crops reduce both the residual N in the soil and the deep percolation of rainfall, consequently reducing NO3-N leaching (CitationBrandi-Dohrn et al. 1997; CitationLogsdon et al. 2002; CitationMacdonald et al. 2005). It is believed that a decrease in soil N content by plant N uptake and a decrease in deep percolation by transpiration of plants can reduce NO3-N leaching from arable soil.
The Chugoku district of Japan is characterized as having an Asian monsoon climate with heavy rains in the main cropping season. The heavy rain often occurs after the application of fertilizer for the main crop, which is in the early growing stage. Therefore, NO3-N leaching occurs easily in soils with high NO3-N content during cropping. NO3-N leaching from arable soil depends on soil texture and is greatest in sandy soils (CitationBergström and Johansson 1991; CitationGeleta et al. 1994; CitationSogbedji et al. 2000). Granitic regosols have a sandy texture with poor physical, chemical and biological characteristics and are widely distributed in the Chugoku district of Japan (CitationHerai et al. 2006). In addition, NO3-N leaching from arable soil depends on the rate of N fertilizer application and the soil N content (CitationDecau et al. 2004; CitationTrindade et al. 1997). Large amounts of NO3-N leaching occur with chemical fertilizer application. To reduce NO3-N leaching from granitic regosol during the June–July rainy season, two methods are generally considered. The reduction of the NO3-N level in soil by plant N uptake is one method. This may be enhanced by controlling the planting density and timing of sawing, or by the selection of crops that have a higher rate of N uptake. To reduce the NO3-N level in soil, it is also effective to use a controlled N release fertilizer (CitationQuiroga-Garza et al. 2001) or a nitrification inhibitor (CitationBall-Coelho and Roy 1999). Increasing the amount of retained N (inorganic N and microbial biomass N) temporarily in the soil during the rainy season is the other method. This may be controlled by microbial biomass N formation using organic manure or by a decrease in the deep percolation of NO3-N in the soil with transpiration by plants. The retained N in the soil is available for plants after the rainy season because NO3-N leaching hardly occurs after the rainy season. CitationHerai et al. (2006) reported that NO3-N leaching from granitic regosol was less with compost application than with chemical fertilizer application, and that the increase in microbial biomass N with compost application reduced soil inorganic N and consequently reduced NO3-N leaching.
Although NO3-N leaching is reduced with compost application in the short term, in the long term compost application increases inorganic N derived from the decomposition of organic N accumulated in the soil, and there is a concern that as much or more NO3-N leaching occurs as with chemical fertilizer application (CitationMaeda et al. 2003). In addition, farmers often apply chemical fertilizer because it is easier to use than compost. In these situations, it is considered that the effect of plant growth on NO3-N leaching becomes more important. In addition, the increases in N uptake and water-use efficiency with the development of root systems (CitationBenjamin et al. 1996; CitationKristensen and Thorup-Kristensen 2004), the increase in microbial biomass with plant growth, and the rate of immobilization of N (CitationJoergensen 2000) and denitrification with increasing microbial biomass (CitationQian et al. 1997) may contribute to reduce NO3-N leaching. Therefore, it is considered that plant growth, which results in N uptake, transpiration and development of the root system, is an important factor affecting NO3-N leaching from soil if heavy rain occurs in the main cropping season.
The objectives of this study were to analyze the effects of N uptake and transpiration by plants on NO3-N leaching from a granitic regosol, and to evaluate the contribution of plant growth to the reduction of NO3-N leaching. For this purpose, a field experiment was conducted using a N application of 300 kg N ha−1 and three levels of planting density of corn. In addition, to analyze the effect of transpiration by plants on NO3-N leaching, a soil column experiment was conducted with four levels of planting density.
MATERIALS AND METHODS
Field experiment
Experimental site
The field experiment was carried out at the Hiroshima University experimental field, Hiroshima Prefecture, Japan (34°23′N, 132°42′E). The experimental area falls within the Asian monsoon area and has a mean annual air temperature of 13°C and a mean annual precipitation of approximately 1,500 mm (25 year mean) with heavy rainfall in June–July. The weather data for the experimental site were obtained from a weather station located 2.6 km from the site. The soil was a granitic regosol with a loamy sand texture containing less than 10% clay content. Details of the soil chemical characteristics at initiation of the site were reported by CitationHerai et al. (2006).
Experimental design
The experimental plot of chemical fertilizer (CF) – conventional planting density treatment (CPD of 60,000 plants ha−1) with 80 cm row spacing and 20 cm plant spacing was initiated in 1996, and has been planted with corn as a summer crop. NO3-N leaching, shoot dry matter and N uptake by corn, soil inorganic N and microbial biomass N were monitored in the CF-CPD treatment from May 2003 to September 2005. In the field experiment of 2005, a CF-high planting density treatment (HPD of 110,000 plants ha−1) with 40 cm row spacing and 20 cm plant spacing, and a CF-no planting (NP) treatment were prepared in the same field block. The CF-NP and CF-HPD treatments have been kept free of vegetation since 1996, except for the CF-HPD treatment in 2002. Corn and oats were planted in the CF-HPD treatment in 2002. A plot measuring 5 m × 8 m was used for each treatment with two replicates.
Corn (Zea mays L. cv. snowdent127S) was sown on 21 May 2003, 18 May 2004 and 26 May 2005 in the CF-CPD and on 26 May 2005 in the CF-HPD treatment. Nitrogen was applied as (NH4)2SO4 at 300 kg N ha−1 in each treatment. An available P level of 100 kg P ha−1 was maintained with superphosphate and fused magnesium
Table 1 Chemical characteristics of the soil before fertilizer application in the field experiment (2005)
Two replicate capillary lysimeters (height × width × length of 500 mm × 300 mm × 600 mm: DAIKI, DIK-6900 COMH-9-30, Kounosu city, Saitama, Japan) were used for each treatment and drainage water was sampled from 80 cm below the soil surface. Drainage samples from the lysimeter were collected 24 h after rainfall (at 28, 36, 43, 48, 54, 62, 83 and 103 days after treatment [DAT] in 2003, at 7, 15, 34, 45, 72, 91, 98 and 107 DAT in 2004, and at 20, 38–41, 44–47, 49, 54, 67, 85, 89 and 109 DAT in 2005). After measuring the drainage volume, drainage samples were stored at 4°C until NO3-N analysis.
Soil was sampled before fertilizer application and subsequently at 0, 9, 15, 30, 60 and 90 DAT in each year. Soil samples were collected at a depth of 0–10 cm and sieved (< 2 mm) before being divided into two subsamples; one sample was stored at 4°C for soil NO3-N, NH4-N and microbial biomass N analysis and the other was air-dried for additional chemical analysis. Ten plants were collected from each of the two replicates of each treatment and the fresh weight of leaves, stems and grains was determined at 15, 30, 49, 60 and 90 DAT. Four plants in each treatment were dried at 80°C for at least 48 h before being weighed. The dried samples were finely ground and stored for chemical analysis.
Soil column experiment
The soil column experiment was conducted from 15 July to 11 August 2005 in a greenhouse. The four treatments used differed in planting density: zero (no plant), one, two and four plants per column (NP, 1-plant, 2-plant and 4-plant treatments). All treatments were replicated six times making a total of 24 soil columns and half of the soil columns were sampled before water application on 1 August. The columns were made of cylindrical polyvinyl chloride (PVC) tubes (inner diameter 20 cm and length 80 cm). The bottom of the PVC tube was capped with a PVC plate that had a hole covered with nylon mesh that was connected to a plug with a tube for collecting drainage samples. The soil column was filled with a sieved (< 2 mm) granitic regosol consisting of 60 cm of subsoil and 15 cm of topsoil. The bulk density of the soil used in the soil column experiment was similar to that of the field soil (1.3 g cm−3).
On 15 July, phosphorus and potassium were applied as superphosphate at 200 kg P ha−1 and as K2SO4 at 200 kg K ha−1, respectively, and dolomite was applied at 500 kg ha−1 for the 15 cm topsoil in each treatment to adjust the soil pH to 6.0. Nitrogen was applied to the soil surface using Ca(NO3)24H2O at 1,500 mg N per column (corresponding to 476 kg N ha−1) to avoid the influence by nitrification of ammonium sulfate. After N application, corn was sown in the 1-plant, 2-plant and 4-plant treatments. From 31 July to 9 August, deionized water corresponding to 40 mm of precipitation was applied every day to the soil surface in each soil column. The total amount of water applied to the soil column (400 mm) was equal to the average amount of precipitation received during the rainy season in this area. Evapotranspiration from the soil column was calculated by measuring the weight of the soil column every day before water was applied. Plant weight during the water application period was estimated using a growth curve and the plant weight at the beginning and end of the water application period, then subtracted from the weight of the soil column. The difference in evapotranspiration between the NP treatment and the 1-plant, 2-plant and 4-plant treatments was assumed to be transpiration by the plant. Drainage samples were collected 24 h after water was applied and stored at 4°C until NO3-N analysis. On 1 and 11 August, shoots were cut at the soil surface and roots and soils were sampled from the topsoil and every 10 cm of the subsoil in each soil column. Roots were sieved from the soil and washed with deionized water. The fresh weights of shoots and roots were measured and dried at 80°C for at least 48 h before being weighed. The dried samples were finely ground and stored for chemical analysis. Soil samples were stored at 4°C until NO3-N analysis.
Chemical analysis
Drainage samples from the lysimeter and soil column were filtered through a 0.2 µm membrane filter (Dismic-13cp, Toyo Roshi, Tokyo, Japan) and the NO3-N concentration of the samples was determined using ion chromatography. The system was composed of an electric conductivity detector (CM-8000, TOSOH Corporation, Tokyo, Japan) and a thermostatic box (CO-8020, TOSOH Corporation) for the separation column (TSKgel IC-Anion-PW, TOSOH Corporation). The amount of NO3-N leached was determined by multiplying the drainage volume by the NO3-N concentration in the drainage samples.
For soil NH4-N analysis, moist soil containing 10 g dry soil was extracted with 100 mL of 2 mol L−1 KCl solution for 1 h. The extracts were filtered using ADVANTEC No.5C filter paper (Toyo Roshi) and the ammonium concentration was determined using the indophenol blue method with a spectrophotometer (Shimadzu, UVmini-1240, Kyoto, Japan). For soil NO3-N analysis, moist soil containing 10 g dry soil was extracted with 100 mL of deionized water for 1 h. The extracts were filtered using ADVANTEC No.5C filter paper and the NO3-N concentration was determined using ion chromatography. The amounts of microbial biomass C and N in the soil were determined using the chloroform fumigation extraction method (CitationBrookes et al. 1985), and the amount of total organic C and total N dissolved in 0.5 mol L−1 K2SO4 extracts were determined using an organic C and total N analyzer (Shimadzu, TOC-V CSN TNM-1, Kyoto, Japan). Microbial biomass C (B C ) and N (B N ) were calculated from the relationships B C = 2.22 × E C and B N = 2.22 × E N , respectively (CitationChowdhury et al. 1999), where E C and E N correspond to the amount of organic C and total N extracted from the fumigated samples minus the amount in the non-fumigated samples, respectively. Microbial biomass C and N in 0–10 cm soil were determined by multiplying the bulk density (1.31 g cm−3). Total C and N in the plant and soil samples were determined using the combustion method with a CN analyzer (Yanaco, MT-700, Kyoto, Japan).
Statistical analysis
Statistical analyses were carried out using the statistical package SPSS for Windows (version 13.0) with the level of significance (P value) set at P < 0.01. Means and standard deviations for each treatment were calculated.
RESULTS
Field experiment
Drainage volume, NO3-N concentration and NO3-N leaching
Cumulative NO3-N leaching and shoot dry matter in the CF-CPD treatment from May 2003 to September 2005 is presented in . Cumulative NO3-N leaching in the CF-CPD treatment showed a similar trend each year and increased markedly during the period between 30 and 60 DAT. The total amount of rainfall during the corn cropping season was 842 mm (3-year average).
In 2005, heavy rainfall was received 36–48, 81 and 103 DAT (). The amount of rainfall 36–48 DAT was 308 mm and particularly heavy rainfall of 213 mm was received 36–39 DAT, including more than 70 mm day−1 37 and 38 DAT and more than 100 mm day−1 of rainfall was observed 81 and 103 DAT. The drainage volume markedly increased from 38 to 41 DAT and small amounts of drainage were observed 44–49, 85–89 and 109 DAT (). The drainage volume was a little lower in the CF-HPD treatment than the CF-NP and CF-CPD treatments, but the differences were not statistically significant.
The NO3-N concentration in the drainage water during the period of 38–41 DAT was lowest in the CF-HPD treatment, followed by the CF-CPD treatment and the CF-NP treatment (). The NO3-N concentration in the drainage water was a little higher in the CF-HPD treatment than the CF-CPD treatments 44–49 DAT, but the difference was not statistically significant. The NO3-N concentration in the drainage water remained above 50 mg N L−1 in the CF-NP treatment after 49 DAT, while remaining very low in the CF-CPD and CF-HPD treatments.
Figure 2 (A) Precipitation and (B) cumulative drainage volume from the capillary lysimeter in the field experiment (2005). CF-NP, chemical fertilizer-no planting; CF-CPD, chemical fertilizer-conventional planting density; CF-HPD, chemical fertilizer-high planting density. Error bars represent standard deviation.
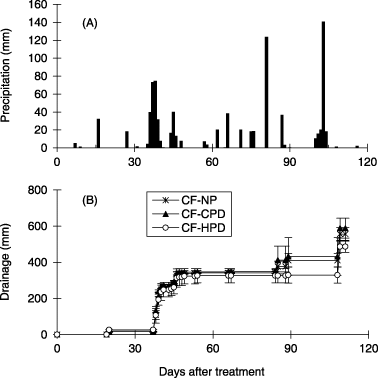
Figure 3 Effect of planting density on (A) nitrate–nitrogen (NO3-N) concentration in the drainage water and (B) cumulative NO3-N leaching from the capillary lysimeter in the field experiment (2005). CF-NP, chemical fertilizer-no planting; CF-CPD, chemical fertilizer-conventional planting density; CF-HPD, chemical fertilizer-high planting density. Error bars represent standard deviation.
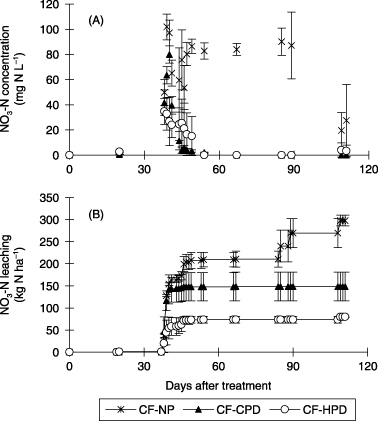
NO3-N leaching markedly increased from 38 to 41 DAT and very little leaching was observed 44–49, 85–89 and 109 DAT in all treatments (). Cumulative NO3-N leaching until 49 DAT in the CF-NP, CF-CPD and CF-HPD treatments was 208, 148 and 73 kg N ha−1, respectively, and NO3-N leaching decreased with increasing plant density. Total NO3-N leaching until 109 DAT in the CF-NP, CF-CPD and CF-HPD treatments was 298, 149 and 80 kg N ha−1, respectively. The ratio of cumulative NO3-N leaching at 49 DAT to the total amount at 109 DAT in the CF-NP, CF-CPD and CF-HPD treatments was 70, 99 and 91%, respectively.
Shoot dry matter and N uptake
Shoot dry matter was 2–4 Mg ha−1 49 DAT and reached 10–14 Mg ha−1 at harvest in the CF-CPD treatment from 2003 to 2005 () Shoot dry matter was lower in 2003 compared to 2004 and 2005. In 2005, shoot dry matter 30 DAT was 0.4 Mg ha−1 in the CF-CPD and 1.2 Mg ha−1 in the CF-HPD treatment (). Forty-nine DAT, shoot dry matter was 1.4–1.7-fold higher in the CF-HPD treatment than in the CF-CPD treatment. Shoot N uptake was 12 kg N ha−1 in the CF-CPD and 40 kg N ha−1 in the CF-HPD treatment 30 DAT and this increased to 55 kg N ha−1 and 103 kg N ha−1, respectively, 49 DAT (). Shoot N uptake was 1.2–3.3-fold higher in the CF-HPD treatment than in the CF-CPD treatment during the cropping season.
Inorganic N in the surface soil and soil microbial biomass N
Inorganic N in the surface soil (0–10 cm) was similar in all treatments () and was high (approximately 300 kg N ha−1) up to 15 DAT, and decreased to 100 kg N ha−1 at 30 DAT and became very low after 60 DAT.
Figure 4 Effect of planting density on inorganic N in the surface soil (0–10 cm) in the field experiment (2005). CF-NP, chemical fertilizer-no planting; CF-CPD, chemical fertilizer-conventional planting density; CF-HPD, chemical fertilizer-high planting density. Error bars represent standard deviation.
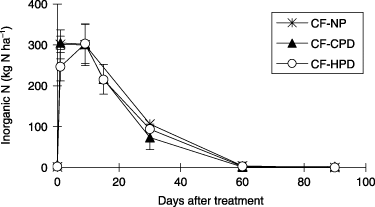
Table 2 Shoot dry matter and shoot N uptake by corn in the field experiment (2005)
Table 3 Microbial biomass N in the field experiment (2005)
Soil microbial biomass N in the CF-CPD treatment increased slightly up to 30 kg N ha−1 60 DAT and then remained constant (). Microbial biomass N in the CF-NP treatment was 14 kg N ha−1 at 15 DAT and slightly increased during the cropping period. The amount of microbial biomass N in the CF-CPD treatment was approximately 6–14 kg N ha−1 higher than in the CF-NP treatment.
Soil column experiment
NO3-N leaching
NO3-N leaching from the soil column increased from 3 to 7 DAT (timing of water application) and was then negligible after 7 DAT (). Total amounts of NO3-N leaching until the end of the water application period were 416, 427, 403 and 374 kg N ha−1 in the NP, 1-plant, 2-plant and 4-plant treatments with the 4-plant treatment being significantly less than the NP treatment.
Drainage and evapotranspiration
The drainage volume from the soil column decreased with increasing planting density. The total drainage volumes during the 10-day water application period were 331, 307, 281 and 266 mm in the NP, 1-plant, 2-plant and 4-plant treatments, respectively. The drainage volume decreased by 24, 50 and 65 mm in the 1-plant, 2-plant and 4-plant treatments compared to the NP treatment (). The cumulative evapotranspiration from the soil column during the water application period increased from 61 to 118 mm with increasing planting density (). Assuming that the differences in evapotranspiration between the NP treatment and
the 1-plant, 2-plant and 4-plant treatments resulted from transpiration by corn, transpiration by corn was estimated to be 19−57 mm for 10 days.NO3-N concentration in the drainage water
The NO3-N concentration in the drainage water was highest 4 DAT in all treatments and increased up to 600 mg N L−1 (). In the 1-plant, 2-plant and 4-plant treatments, NO3-N concentrations were lower 3 DAT and higher 5–6 DAT than in the NP treatment.
Dry matter and N uptake
Dry matter and N uptake of shoots and roots at the beginning and end of the water application period are
Figure 6 Effect of planting density on (A) cumulative drainage volume and (B) cumulative evapotranspiration in the soil column experiment. NP, no plant; 1-plant, one plant per soil column; 2-plant, two plants per soil column; 4-plant, four plants per soil column. Error bars represent standard deviation.
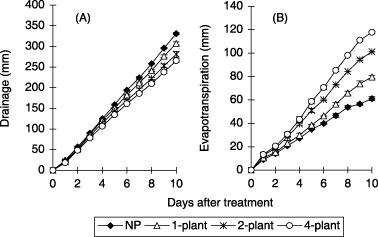
DISCUSSION
Relationships between NO3-N leaching, retained N in soil and N uptake by corn
In the field experiment, a large amount of NO3-N leaching was observed after more than 300 mm of heavy rainfall 36–48 DAT, including more than 70 mm day−1 for two consecutive days (,) in 2005. The drainage volume was not significantly different among the three treatments (), but NO3-N concentration in the drainage water significantly decreased in the CF-CPD and CF-HPD treatments compared to the CF-NP treatment 36–48 DAT (). In addition, the transpiration by corn would be low on a rainy day. The effect of the transpiration by corn on drainage volume was probably negligible under heavy rainfall conditions of more than 100 mm over 1–2 days. Therefore, the main reason for the decrease in NO3-N leaching in the CF-CPD and CF-HPD treatments was the decrease in NO3-N concentration in the drainage water. NO3-N concentration in the drainage water during the rainy season was closely linked to soil NO3-N contents in the 0–30 cm soil profile (CitationRoth and Fox 1990) and in the 0–120 cm soil profile (CitationJemison and Fox 1994) under field conditions. In our experiment, inorganic N in the surface
Table 4 Dry matter and N uptake at the beginning and the end of the water application period in the soil column experiment
Relationships between NO3-N leaching, retained NO3-N in soil and transpiration by corn
The effect of transpiration by corn on drainage volume was observed in the soil column experiment with the rate of water application set at 40 mm day−1 for 10 days. Cumulative NO3-N leaching, the amount of retained NO3-N
Figure 10 Effect of planting density on cumulative nitrate–nitrogen (NO3-N) leaching, plant N uptake and the amount of retained NO3-N in the soil at the end of water application in the soil column experiment. Error bars represent standard deviation.
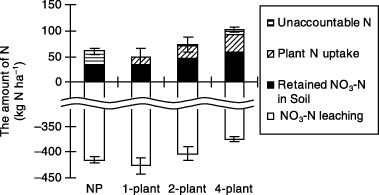
Figure 11 Relationship between the amount of retained nitrate–nitrogen (NO3-N) in the soil at the end of water application and cumulative transpiration by corn for 10 days of water application in the soil column experiment. **P < 0.01.
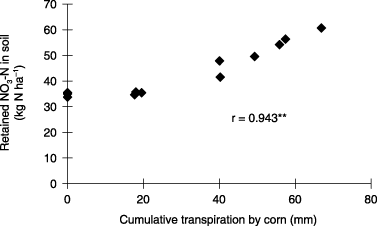
In the field experiment, transpiration by corn during the rainy season was probably negligible because of heavy rain. The retained N in the field soil could be increased if the transpiration rate of corn was high, as in the soil column experiment. NO3-N leaching hardly occurred after the rainy season under field conditions because of the little amount of rainfall and plant uptake of retained N in the soil. Therefore, it was considered that the increase of retained N in the soil derived from the increasing of transpiration by corn in the field was effective to reduce NO3-N leaching during the rainy season.
In this study, it can be concluded that NO3-N leaching from a granitic regosol during the rainy season can be reduced by increasing plant density because of an increase in N uptake by the plants and an increase in retained N in the soil derived from increasing transpiration by plants.
ACKNOWLEDGMENTS
The authors would like to acknowledge the Ministry of Agriculture, Forestry and Fisheries of Japan for financial support. We thank Professor Minoru Yamauchi (National Agricultural Research Center for Western Region) for his valuable advice and discussions.
REFERENCES
- Andraski , TW , Bundy , LG and Brye , KR . 2000 . Crop management and corn nitrogen rate effects on nitrate leaching . JEnvironQual , 29 : 1095 – 1103 .
- Thomsen , IK , Hansen , JF , Kjellerup , V and Christensen , BT . 1993 . Effects of cropping system and rates of nitrogen in animal slurry and mineral fertilizer on nitrate leaching from a sandy loam . Soil Use Manage. , 9 : 53 – 58 .
- van der Ploeg , RR , Ringe , H , Machulla , G and Hermsmeyer , D . 1997 . Postwar nitrogen use efficiency in West German agriculture and groundwater quality . JEnvironQual , 26 : 1203 – 1212 .
- Brandi-Dohrn , FM , Dick , RP , Hess , M , Kauffman , SM , Hemphill , DD and Selker , JS . 1997 . Nitrate leaching under a cereal rye cover crop . JEnvironQual , 26 : 181 – 188 .
- Logsdon , SD , Kaspar , TC , Meek , DW and Prueger , JH . 2002 . Nitrate leaching as influenced by cover crops in large soil monoliths . AgronJ , 94 : 807 – 814 .
- Macdonald , AJ , Poulton , PR , Howe , MT , Goulding , KWT and Powlson , DS . 2005 . The use of cover crops in cereal-based cropping systems to control nitrate leaching in SE England . Plant Soil , 273 : 355 – 373 .
- Bergström , L and Johansson , R . 1991 . Leaching of nitrate from monolith lysimeters of different types of agricultural soils . JEnvironQual , 20 : 801 – 807 .
- Geleta , S , Sabbagh , GJ Stone , JF . 1994 . Importance of soil and cropping systems in the development of regional water quality policies . JEnvironQual , 23 : 36 – 42 .
- Sogbedji , JM , van Es , HM , Yang , CL , Geohring , LD and Magdoff , FR . 2000 . Nitrate leaching and nitrogen budget as affected by maize nitrogen rate and soil type . JEnvironQual , 29 : 1813 – 1820 .
- Herai , Y , Kouno , K , Hashimoto , M and Nagaoka , T . 2006 . Relationships between microbial biomass nitrogen, nitrate leaching and nitrogen uptake by corn in a compost and chemical fertilizer-amended regosol . Soil SciPlant Nutr , 52 : 186 – 194 .
- Decau , ML , Simon , JC and Jacquet , A . 2004 . Nitrate leaching under grassland as affected by mineral nitrogen fertilization and cattle urine . JEnvironQual , 33 : 637 – 644 .
- Trindade , H , Coutinho , J , VanBeusichem , ML , Scholefield , D and Moreira , N . 1997 . Nitrate leaching from sandy loam soils under a double-cropping forage system estimated from suction-probe measurements . Plant Soil , 195 : 247 – 256 .
- Quiroga-Garza , HM , Picchioni , GA and Remmenga , MD . 2001 . Bermudagrass fertilized with slow-release nitrogen sources. I. Nitrogen uptake and potential leaching losses . JEnvironQual , 30 : 440 – 448 .
- Ball-Coelho , BR and Roy , RC . 1999 . Enhanced ammonium sources to reduce nitrate leaching . Nutrient CyclAgroecosyst , 54 : 73 – 80 .
- Maeda , M , Zhao , B , Ozaki , Y and Yoneyama , T . 2003 . Nitrate leaching in an Andisol treated with different types of fertilizers . EnvironPollut , 121 : 477 – 487 .
- Benjamin , JG , Ahuja , LR and Allmaras , RR . 1996 . Modelling corn rooting patterns and their effects on water uptake and nitrate leaching . Plant Soil , 179 : 223 – 232 .
- Kristensen , HL and Thorup-Kristensen , K . 2004 . Root growth and nitrate uptake of three different catch crops in deep soil layers . Soil SciSocAmJ , 68 : 529 – 537 .
- Joergensen , RG . 2000 . Ergosterol and microbial biomass in the rhizosphere of grassland soils . Soil BiolBiochem , 32 : 647 – 652 .
- Qian , JH , Doran , JW and Walters , DT . 1997 . Maize plant contributions to root zone available carbon and microbial transformations of nitrogen . Soil BiolBiochem , 29 : 1451 – 1462 .
- Brookes , PC , Landman , A , Pruden , G and Jenkinson , DS . 1985 . Chloroform fumigation and the release of soil nitrogen: A rapid direct extraction method to measure microbial biomass nitrogen in soil . Soil BiolBiochem , 17 : 837 – 842 .
- Chowdhury , MAH , Kouno , K and Ando , T . 1999 . Correlation among microbial biomass S, soil properties, and other biomass nutrients . Soil SciPlant Nutr , 45 : 175 – 186 .
- Roth , GW and Fox , RH . 1990 . Soil nitrate accumulations following nitrogen-fertilized corn in Pennsylvania . JEnvironQual , 19 : 243 – 248 .
- Jemison , JM and Fox , RH . 1994 . Nitrate leaching from nitrogen-fertilized and manured corn measured with zero-tension pan lysimeters . JEnvironQual , 23 : 337 – 343 .
- Nishio , T , Li , XH and Komada , M . 2002 . Comparison of fate of nitrogen applied to 4 different kinds of soils with particular reference to denitrification . Soil SciPlant Nutr , 48 : 307 – 313 .