Abstract
Background
During the last three decades hematopoietic stem cells (HSC) have become a standard protocol for the treatment of many hematologic malignancies and non-malignant disorders. Umbilical cord blood (UCB), as a source of HSCs, has many advantages compared with other sources. One major drawback in using this source in treatment of adult patients is the low HSC dose available. Ex vivo expansion of HSCs is a solution to overcome this limitation. In this study we used TEPA, as a Cu chelator, and human bone marrow (BM) mesenchymal stem cells (MSCs) to investigate expansion rate of UCB-HSCs.
Materials and methods
CB-HSCs were isolated using miniMACS magnetic separation system. We cultured the enriched CD34+cells in various conditions: culture condition A, supplemented only with recombinant cytokines; culture condition B, supplemented with BM-MSCs as a cell feeder layer and recombinant cytokines; culture condition C, supplemented with recombinant cytokines and TEPA; culture condition D, supplemented with recombinant cytokines, BM-MSCs as a cell feeder layer and TEPA. In order to evaluate the HSC expansion, we performed cell count, analysis of CD34+ expression by flow cytometry, and colony-forming cell assay on Day 10 after culture.
Results
The most fold increase in CD34+ cell, total cell, and total colony numbers was observed in culture condition D (110.11 ± 15.3, 118.5 ± 21, and 172.9 ± 44.7, respectively) compared to other conditions.
Conclusion
The results showed that co-culture of HSCs with BM-MSCs in the presence of copper chelating agent (TEPA) could dramatically increase expansion rate of UCB-HSCs. Therefore, this strategy could be useful for HSC expansion.
Introduction
The hematopoietic stem cells (HSCs) have become a tool for the treatment of many hematologic malignancies and non-malignant disorders.Citation1–Citation3 HSCs are characterized by their capacity for self-renewal and differentiation into the various types of mature blood cells.Citation4,Citation5 Umbilical cord blood (UCB), collected from the postpartum placenta and cord, is a promising alternative to bone marrow (BM) as a source of HSCs for HSC transplantation, because of its large potential donor pool, rapid availability, and lower incidence of graft-versus-host disease (GVHD) despite human leucocyte antigen disparity.Citation6 One major drawback of UCB currently limiting the efficacy of umbilical cord is the low cell dose available for transplantation in an adult patient (the optimal dose for an adult is ≥2.5 × 106 CD34+ cells/kg), which can lead to delayed reconstitution of adult BM function and higher rates of graft failure.Citation7–Citation12
Ex vivo expansion of HSCs was suggested as a potential approach in obtaining larger cell numbers. It is well known that HSCs can be expanded in ex vivo culture if they are treated with cytokines. ‘Unfortunately, this kind of expansion leads to cell differentiation and loss of ‘‘stemness’’.’Citation13,Citation14 This is presumably due to a lack of appropriate cues that are provided in vivo by the microenvironment.
The recent data suggest that direct cell-to-cell contact between stromal cells and HSCs is necessary for stem cell survival, cell-cycle regulation, migration, and engraftment. In recent years, many studies reported a synergistic effect using recombinant cytokines and mesenchymal stem cells (MSCs)-derived cell feeder layer in proliferation and differentiation of HSCs.Citation15–Citation21
Interestingly, recent studies showed that the high-affinity copper (Cu) chelator TEPA, together with early acting cytokines (thrombopoietin (TPO), stem cell factor (SCF), interleukin-6 (IL-6), and Flt3 ligand (FL)) can promote the expansion of both human cord blood-derived hematopoietic progenitor cells and HSCs in culture.Citation22–Citation26 In vitro studies showed that addition of Cu salts augmented the retinoic acid-induced differentiation of HL-60 human myeloid leukemia cell line.Citation27 Lowering of the cellular Cu level by the Cu chelator tetraethylenepentamine (TEPA) blocked 1,25-dihydroxy vitamin D or phorbol 12-myristate 13-acetate-induced U937 cell differentiation, and this block was reversed by adding Cu.Citation28 In the present study, we investigated the effect of TEPA on expansion of CD34+ cells derived from UCB in co-culture with BM-derived MSCs. For this purpose we used cultures of CB-derived purified CD34+ cells grown in cytokine-supplemented liquid medium with or without feeder in high and low levels of cellular Cu.
Materials and methods
Collection and purification of CB-derived CD34+ cells
The cells were separated from UCB after obtaining informed consent from normal full-term pregnancies. The samples were processed within 4 hours postpartum. Blood was mixed with hydroxyethyl starch (HES, stem Cell Technologies, Copenhagen, Denmark) to remove the majority of RBCs in 30-minute intervals. For isolation of mononuclear cells (MNCs), the leukocyte-rich fraction was harvested, layered on Ficoll-Hypaque (1.077 ± 0.001 kg/l, Sigma, St. Louis, MO, USA) and centrifuged at 400 g for 30 minutes. Then, the MNCs were incubated with anti-human CD34 antibody conjugated with Micro Beads (Miltenyi Biotec, Auburn, CA, USA) for 30 minutes at 4°C. CD34+-enriched cells were isolated using two cycles of magnetic-activated cell separation (MACS) system (Miltenyi Biotec, Auburn, CA, USA). The averaged percentage of CD34+ cells among the CD34+-enriched cells was about 62% by flow cytometric analysis (BD Bioscience, San Diego, CA, USA).Citation29
Collection and purification of BM-derived MSCs
MSCs were obtained from fresh BM of healthy donors in heparin anticoagulant (informed consent was obtained). The samples were gently laid on ficoll-hypaque gradient. The BM MNCs were harvested and washed with Dulbecco's modified Eagle's medium (DMEM) (Sigma, St. Louis, MO, USA). The cells were plated in culture flasks in DMEM\low Glu\10% fetal bovine serum (FBS) and incubated at 37°C in a humidified atmosphere of 5% CO2. Non-adherent and loosely attached cells were removed by replacing the mediumCitation30 and adherent cells were left to expand. Confluent cultures were detached by 0.25% trypsin-ethylenediaminetetra-acetate (Stem Cell Technologies, Copenhagen, Denmark) and replated at 1 × 104 cells in six-well plates (Nunc, Roskilde, Denmark) to reach 80% confluence. The phenotype of all human MSCs was tested by flow cytometry: CD markers (Dako, Copenhagen, Denmark) including CD90, CD105, CD166, and CD73 (Dako, Copenhagen, Denmark) had to be present whereas CD34 and CD45 had to be absent.Citation19
Differentiation of BM-derived MSCs
MSCs had been tested for their tential to differentiate along the osteogenic lineage using differentiation media containing dexamethasone solution (Chemicon, Billerica, MA, USA), ascorbic acid-2phosphate, DMEM-low glucose (Sigma, St. Louis, MO, USA), and glycerol 2-phosphate solution (Chemicon, Billerica, MA, USA). After differentiation under proper stimuli, the differentiation potential along the osteoblastic series was evaluated by the expression of alkaline phosphatase (ALP) (Sigma, St. Louis, MO, USA) and alizarin red staining (Sigma, St. Louis, MO, USA).Citation30
MTT assay
The MTT (3-[4,5-dimethylthiazol-2-yl]-2,5-diphenyl tetrazoliumbromide) assay is a means of measuring the activity of living cells via mitochondrial dehydrogenases. To investigate TEPA effects on MSCs, it is necessary to make some measures of cellular activity as an indicator of cell damage or cytotoxicity. Procedure was performed according to company instructions.Citation31 After culture of MSCs in presence of TEPA (10 µM), culture media were removed and MTT (5 mg/ml) was added to cells. By dissolving MTT-formazan crystals in dimethyl sulfoxide, the absorbance was recorded at 570 nm.
Cu determination
Overall cellular Cu content was determined as previously described. Briefly, the cells were harvested and washed using phosphate-buffered saline (PBS). Aliquots, containing 2 × 106 cells, were then transferred to metal-free Eppendorf tubes and pelleted. The pellets were brought to a concentration of 1 × 1010/l cells using 0.03 molar ultra pure nitric acid (Mallinckrodt Baker B.V, Deventer, and the Netherlands). The samples were sonicated and then analyzed using a furnace atomic absorption spectro photometry (model 670 G, Shimadzu, Japan) in wavelength of 324.7 and 0.7 nm slit width.Citation23
Cell culture experiments
CD34+-enriched cells were plated in various culture conditions at 1 × 104 cells/ml for 10 days. The culture conditions for the present ex vivo expansion study were classified into four conditions:
•. | Culture condition A, supplemented only with recombinant cytokines consisting of 100 ng/ml each of flt3-ligand (FL), SCF and TPO both without a cell feeder layer and TEPA 10 Mμ. | ||||
•. | Culture condition B, supplemented with the recombinant cytokines (the same concentration above) and BM-derived MSCs as a cell feeder layer without TEPA. | ||||
•. | Culture condition C, supplemented with the recombinant cytokines (the same concentration above) and TEPA 10 Mμ without BM-derived MSCs as a cell feeder layer. | ||||
•. | Culture condition D, supplemented with the recombinant cytokines (the same concentration above), BM-derived MSCs as a cell feeder layer and TEPA 10 Mμ. |
Twice a week, half the volume of fresh medium and cytokines with or without TEPA was exchanged in all cultures. Before culture initiation and after 10 days, the cells were carefully counted for total cell number and were analyzed for CD34+ by flow cytometry.
Colony-forming cell assay
For various culture conditions assayed, duplicate colony-forming cell (CFU) assays were performed. 1 × 103 CD34+-enriched cells were taken before culture initiation or after 10 days in various culture conditions. The cells were plated in methylcellulose culture medium containing 30% FBS, 1% bovine serum albumin, 10−4 M 2-mercapto ethanol, 2 mM l-glutamine, 50 ng/ml rh SCF, 10 ng/ml rh granulocyte, macrophage-colony stimulating factor (GM-CSF), 10 ng/ml rh IL-3, and 3 units/ml rh erythropoietin (Stem Cell Technologies, Vancouver, Canada)Citation32 for 14 days at 37°C under 5% CO2 in a humidified incubator. Colonies consisting of 40 cells or more were known as colony forming cells (CFCs) and were counted using an inverted light microscope. The expansion folds were calculated by comparing the CFCs obtained after 10 days with the CFCs before culture initiation.Citation33
Flow cytometric analysis
Ex vivo expanded HSCs in culture conditions A, B, C, and D at Day 10 of culture were collected and stained with flourescein isothiocyanate (FITC)-conjugated CD34 antibody and PE-conjugated CD38 antibody (BD Bioscience, San Diego, CA, USA) at 4°C for 30 minutes. After washing with PBS, the cells were fixed with 1% paraformaldehyde (Sigma, St. Louis, MO, USA). Appropriate antibodies served as a negative isotype control.Citation34
Statistical analysis
Means of different experimental groups were compared using Paired t test. A 95% confidence interval was chosen and P < 0.05 was considered statistically significant by analysis of variance analysis.
Results
Characterization of BM-derived MSCs
Immunophenotype of BM-derived MSCs was analyzed with flow cytometry. CD90, CD105, CD166, and CD73 expression was 96.8, 93, 98.8 and 94%, respectively, whereas CD34 and CD45 expression was 2.8 and 3.8%, respectively. Osteogenic differentiation potential of BM-derived MSCs was tested after stimulation by dexamethasone, beta-glycerol phosphate, ascorbic acid, and 10% FBS. Cultured MSCs showed an osteogenic differentiation with increased ALP expression and forming extracellular calcium matrix on Day 17 of differentiation.
MTT assay
The 10 µM concentration of TEPA had no obvious effect on cell proliferation and viability of BM-derived MSCs ().
Effect of TEPA on Cu
The effect of TEPA on cellular Cu content was determined. Intracellular concentration of Cu was 7 ng/107 cells measured by atomic absorption. When the Cu chelator, TEPA, was included in the culture medium at 10 mM concentration, intracellular Cu content dropped to 4 ng/107 cells. These results indicate that intracellular Cu content can be modulated by the addition of TEPA to the culture medium.
Assessment of expansion in various conditions
To determine whether MSCs and reduced Cu were capable of supporting ex vivo expansion of UCB-derived hematopoietic cells, four culture systems were established in parallel as indicated in ‘Materials and methods.’ Total number of total nucleated cells (TNCs), CD34+ cells, and CFU-C were evaluated on Day 10 ( and ).
Figure 2. Co-culture of HSCs with MSCs after 10 days expansion in serum-free media supplemented with cytokine cocktail (40×).
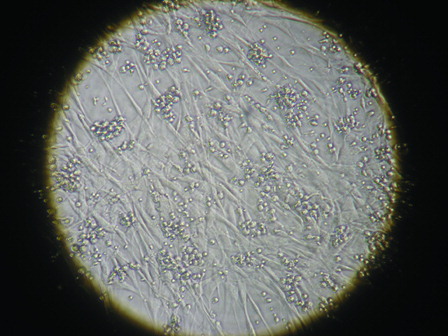
Figure 3. Expansion folds after 10 days in various culture conditions. (A), Expansion fold of TNCs; (B), Expansion fold of CD34+ cells; (C), Expansion fold of CD34+ CD38− cells; and (D), Expansion fold of CFCs. Condition A, supplemented only with the cytokine cocktail; Condition B, supplemented with the cytokine cocktail and BM-derived MSCs; Condition C, supplemented with cytokine cocktail and TEPA; Condition D, supplemented with the cytokine cocktail, BM-derived MSCs as a cell feeder layer and TEPA. Data are expressed as mean ± SD of mean (n = 5); significant differences observed between culture conditions (*P < 0.05).
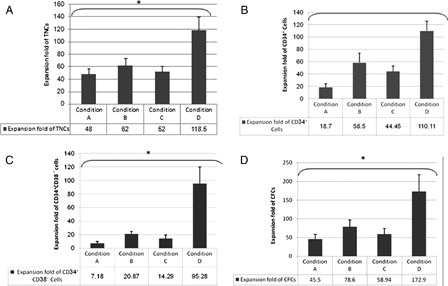
Culture condition A
The average fold expansion of TNCs, CD34+ cells, and CFU-C in this condition was 48 ± 8.7, 18.7 ± 5.6, and 45.5 ± 12.7, respectively.
Culture condition B
Effect of MSCs on ex vivo expansion of TNCs, CD34+ cells, and CFU-C were studied in synergy with cytokines. The expansion magnitude of CD34+ cells by co-culture system was 58.5 ± 15.4-fold. Fold expansion of TNCs and CFU-C in this culture were 62 ± 11.2 and 78.6 ± 18.6, respectively.
Culture condition C
In order to evaluate the effect of Cu modulation on expansion, CD34+ cultures were maintained continuously in the presence of TEPA. The expansion magnitude of CD34+ cells in this culture condition was 44.45 ± 8.7-fold. The average fold expansion of TNCs and CFU-C in this condition increased to 52 ± 8.8 and 58.94 ± 15.4, respectively.
Culture condition D
The effect of co-culture of MSCs in synergy with cytokines and TEPA was evaluated on UCB-HSCs expansion. After 10 days of culture, the number of TNCs, CD34+cells, and CFU-C in this system was remarkably increased. Fold increase of TNCs, CD34+ cells, and CFU-C was approximately 118.5 ± 21.78, 110.11 ± 15.3, and 172.9 ± 44.7, respectively.
Immunophenotyping analysis after expansion
We examined the expression of the stem/progenitor cell CD34 and CD38 markers by flow cytometry analysis. The culture condition D included more cells with a less differentiated phenotype CD34+CD38− (39.7 ± 4.86%; P < 0.05) compared to the three other culture conditions (culture condition A: 7.3% ± 0.8, culture condition B: 12.5 ± 2.6%, culture condition C: 15.84 ± 3.8, respectively) ().
Figure 4. Analysis of CD34 and CD38 expression among HSCs before culture and after 10 days expansion in different culture conditions. FL1: Presented CD34, FL2: Presented CD38 3A, before culture (zero time); 3B, Culture condition A (from fig. 3); 3C, Culture condition B; 3D, Culture condition C; and 3E, Culture condition D.
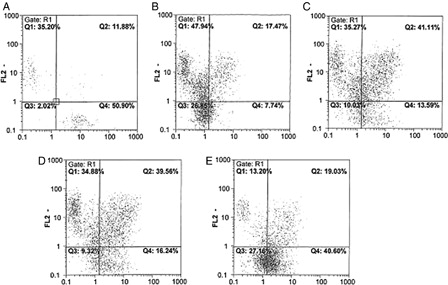
Discussion
Ex vivo expansion of HSCs is a very promising approach for research and therapeutic purposes.Citation35–Citation37 A number of previous studies had sought to optimize ex vivo expansion of CD34+ cells.Citation17,Citation38 HSCs can be expanded in culture media supplemented with cytokines. However, this kind of expansion is accompanied by concomitant differentiation and commitment of cells.Citation14 In recent studies, it has been shown that stromal feeder layers can be able to support HSC expansion mediated by growth factors and cell-to-cell contact.Citation39,Citation40
Besides, it has been shown that decrease of cellular Cu content by TEPA, a synthetic linear polyamine chelating Cu, results in acceleration of HSC expansion.Citation22–Citation25 All previous studies have focused on the effects of MSCs and TEPA on HSCs distinctly, but in the present study the supportive effects of TEPA, MSCs, and cytokines were assessed simultaneously on expansion of HSCs in four culture conditions.
When CD34+-enriched cells were cultivated in cultures supplemented only with recombinant cytokine (culture condition A), the TNCs, CD34+ cells, and CFU-C all increased (about 48-, 18.77-, and 45.5-fold, respectively), but this increase was not significant compared with other conditions. The results were consistent with previous studies.Citation41–Citation43
In order to confirm the role of MSCs on the proliferation and maintenance of UCB-derived HSCs, purified CD34+-enriched cells were cultured over BM-derived MSCs as a cell feeder with recombinant cytokines (culture condition B). TNCs, CD34+ cells, and CFU-C in culture condition B were increased about 62-, 58.5-, and 78.6-fold, respectively, showing a higher expansion rate than those in cultures supplemented only with cytokine (culture condition A). Comparing the results of these two conditions showed that during cultivation, MSCs cause increasing proliferation of TNCs and also maintenance of HSCs in the primary mode. Studies have suggested that MSCs provide a variety of signals (adhesive ligands, cytokines, and extracellular matrix proteins), transcription factors, and signaling molecules shown to regulate proliferation, differentiation, and survival of HSCs in vitro and in vivo.Citation44 Cell-to-cell contact between HSCs and MSCs appears to be an important question for keeping stemness with a significant impact on the function, phenotype, migratory behavior, and gene expression profile of HSCs in vitro.Citation19,Citation45–Citation47 Alakel and colleaguesCitation19 showed that non-adherent HSCs were different from adherent ones in terms of functional, phenotypic, and clonogenic parameters. They showed that the adherent cell fraction includes more primitive cells; hence the MSCs have significant impact on HSCs in ex vivo system. The more primitive HSCs have higher affinity for MSCs.Citation48
In culture condition A, many primary cells were differentiated and lost surface CD34 marker. As a result, MSCs binding is essential for HSCs expansion.
Cu is involved in many cellular processes including regulation of gene expression, cellular protein functions, and cell proliferation and differentiation. However, due to its role in catalyzing the production of reactive oxygen species (ROS), excess intracellular Cu is toxic.Citation49
CD34+-enriched cells cultured with cytokines and TEPA (culture condition C) showed increased expansion rate of TNCs, CD34+ cells, and CFU-C (52-, 44.45-, and 58.94-fold increases, respectively). Although expansion in this culture was higher than that in culture condition A, it was lower than expansion in culture condition B. The reduction of expansion might be as a result of absence of cell-to-cell contact and cytokines secreted from MSCs. It is known that both the proliferation and differentiation processes are affected (in a reciprocal manner) by the Cu content, but differentiation is the primary target in contrast to proliferation.Citation23
Peled et al.Citation23–Citation25 reported the effects of TEPA 10 Mm on expansion of CD34+ cells.Citation23–Citation25 In the first study in 2001, they examined the effect of TEPA, Cu, and ceruloplasmin on cellular Cu and CD34+ cell expansion. Cultures supplemented with recombinant cytokines and TEPA showed higher numbers of total nucleated cells, CD34+ cells and CFU content, low Cu content, and a delay in differentiation compared to cultures supplemented only with the same cytokines, whereas cultures supplemented with Cu showed reverse results.Citation23 These results were coordinated with our results. Peled et al. in another study in 2004, evaluated the effect of TEPA on CD34+ cell expansion as well on their severe combined immunodeficiency (SCID) engraftment potential. Their results indicated that TEPA increase cell expansion and thereby promote engraftment capabilities.Citation24 Although mechanism of TEPA action is unknown, it is suggested that TEPA attenuates ROS level and CCO activity which is essential for maintenance of HSCs in BM.Citation50,Citation51
Additionally, we considered a culture supplemented with BM-derived MSCs as a cell feeder layer in the presence of recombinant cytokines and TEPA (culture condition D) for the first time. TNCs, CD34+ cells, and CFU-C in this culture were increased about 118.5-, 110.11-, and 172.9-fold, respectively. Immunophenotypic analysis of HSCs in various culture conditions suggested that culture condition D could better expand CD34+ CD38− (cells with less differentiation than other culture conditions. Indeed, our experimental work indicated that reducing intracellular Cu using TEPA in co-culture could expand CD34+ cells with higher efficiency compared to other conditions. Application of MSCs and TEPA in combination could be a new approach to enhance HSC expansion. Hopefully, this strategy might be useful for HSC expansion and better transfusion engraft with further research in the future.
Acknowledgements
We would like to thank the Blood transfusion research center for their support.
References
- Conrad PD, Emerson SG. Ex vivo expansion of hematopoietic cells from umbilical cord blood for clinical transplantation. J Leukoc Biol. 1998;64:147–55.
- Lim M, Ye H, Panoskaltsis N, Drakakis EM, Yue X, Cass AE, et al. Intelligent bioprocessing for hematopoietic cell cultures using monitoring and design of experiments. Biotechnol Adv. 2007;25(4):353–68.
- Gordon MY. Stem cells for regenerative medicine – biological attributes and clinical application. Exp Hematol. 2008;36(6):726–32.
- Reya T, Morrison SJ, Clarke MF, Weissman IL. Stem cells, cancer, and cancer stem cells. Nature. 2001;414:105–11.
- Kondo M, Wagers AJ, Manz MG, Prohaska SS, Scherer DC, Beilhack GF, et al. Biology of hematopoietic stem cells and progenitors: implications for clinical application. Annu Rev Immunol. 2003;21:759–806.
- Lane TA. Umbilical cord blood grafts for hematopoietic transplantation in adults: a cup half empty or half full? Transfusion. 2005;45(6):1027–34.
- Gluckman E, Rocha V, Chevret S. Results of unrelated umbilical cord blood hematopoietic stem cell transplantation. Rev Clin Exp Hematol. 2001;5(2):87–99.
- Migliaccio AR, Adamson JW, Stevens CE, Dobrila NL, Carrier CM, Rubinstein P. Cell dose and speed of engraftment in placental/umbilical cord blood transplantation: graft progenitor cell content is a better predictor than nucleated cell quantity. Blood. 2000;96:2717–22.
- Gilmore GL, Depasquale DK, Lister J, Shadduck RK. Ex vivo expansion of human umbilical cord blood and peripheral blood CD34+ hematopoietic stem cells. Exp Hematol. 2000;28(11):1297–305.
- Kelly SS, Sola CB, de Lima M, Shpall E. Ex vivo expansion of cord blood. Bone Marrow Transplant. 2009;44(10):673–81.
- Yao CL, Chu IM, Hsieh TB, Hwang SM. A systematic strategy to optimize ex vivo expansion medium for human hematopoietic stem cells derived from umbilical cord blood mononuclear cells. Exp Hematol. 2004;32(8):720–7.
- Rocha V, Wagner JE, Sobocinski KA, Klein JP, Zhang MJ, Horowitz MM, et al. Graft versus host disease in children who have received a cord blood or bone marrow transplant from an HLA-identical sibling. N Engl J Med. 2000;342:1846–54.
- Shpall EJ, Quinones R, Giller R, Zeng C, Baron AE, Jones RB, et al. Transplantation of ex vivo expanded cord blood. Biol Blood Marrow Transplant. 2002;8(7):368–76.
- Sorrentino BP. Clinical strategies for expansion of haematopoietic stem cells. Nat Rev Immunol. 2004;4(11):878–88.
- Martinez-Agosto JA, Mikkola HK, Hartenstein V, Banerjee U. The hematopoietic stem cell and its niche: a comparative view. Genes Dev. 2007;21:3044–60.
- Calvi LM, Adams GB, Weibrecht KW, Weber JM, Olson DP, Knight MC, et al. Osteoblastic cells regulate the haematopoietic stem cell niche. Nature. 2003;425(6960):778–9.
- Robinson S, Niu T, de Lima M, Ng J, Yang H, McMannis J, et al. Ex vivo expansion of umbilical cord blood. Cytotherapy. 2005;7(3):243–50.
- Gottschling S, Saffrich R, Seckinger A, Krause U, Horsch K, Miesala K, et al. Human mesenchymal stromal cells regulate initial self-renewing divisions of hematopoietic progenitor cells by a beta1-integrin-dependent mechanism. Stem Cell. 2007;25(3):798–806.
- Jing D, Fonseca AV, Alakel N, Fierro FA, Muller K, Bornhauser M, et al. Hematopoietic stem cells in co-culture with mesenchymalstromal cells – modeling the niche compartments in vitro. Hematologica. 2010;95(4):542–50.
- Breems DA, Blokland EA, Siebel KE, Mayen AE, Engels LJ, Ploemacher RE. Stroma-contact prevents loss of hematopoietic stem cell quality during ex vivo expansion of CD34+ mobilized peripheral blood stem cells. Blood. 1998;91(1):111–7.
- Blank U, Karlsson G, Karlsson S. Signaling pathways governing stem cell fate. Blood. 2008;111:492–503.
- Huang X, Pierce LJ, Cobine PA, Winge DR, Spangrude GJ. Copper modulates the differentiation of mouse hematopoietic progenitor cells in culture. Cell Transplant. 2009;18(8):887–97.
- Peled T, Landau E, Prus E, Treves AJ, Nagler A, Fibach E. Cellular copper content modulates differentiation and self-renewal in cultures of cord blood-derived CD34+ cells. Br J Haematol. 2002;116:655–61.
- Peled T, Landau E, Mandel J, Glukhman E, Goudsmid NR, Nagler A, et al. Linear polyamine copper chelator tetraethylenepentamine augments long-term ex vivo expansion of cord blood-derived CD34+ cells and increases their engraftment potential in NOD/SCID mice. Exp Hematol. 2004;32(6):547–55.
- Peled T, Glukhman E, Hasson N, Adi S, Assor H, Yudin D, et al. Chelatable cellular copper modulates differentiation and self-renewal of cord blood-derived hematopoietic progenitor cells. Exp Hematol. 2005;33(10):1092–100.
- Percival SS, Layden-Patrice M. HL-60 cells can be made copper deficient by incubating with tetraethylenepentamine. J Nutr. 1992;122(12):2424–9.
- Bae B, Percival S. Copper uptake and intracellular distribution during retinoic acid-induced differentiation of HL-60 Cells. J Nutr Biochem. 1994;5(9):457–61.
- Huang ZL, Failla ML, Reeves PG. Differentiation of human U937promonocytic cells is impaired by moderate copper deficiency. Exp Biol Med. (Maywood) 2001;226(3):222–8.
- Yang S, Cai H, Jin H, Fan J, Tan WS. Hematopoietic reconstitution of CD34+ cells derived from short-term cultured cord blood mononuclear cells. Biotechnol. Bioprocess Eng. 2009;14(4):429–35.
- Oswald J, Boxberger S, Jørgensen B, Feldmann S, Ehninger G, Bornhäuser M, et al. Mesenchymal stem cells can be differentiated into endothelial cells in vitro. Stem Cells. 2004;22(3):377–84.
- www.Sigmaaldrich.com
- Robinson SN, Ng J, Niu T, Yang H, McMannis JD, Karandish S, et al. Superior ex vivo cord blood expansion following co-culture with bone marrow-derived mesenchymal stem cells. Bone Marrow Transplant. 2006;37(4):359–66.
- Almeida-Porada G, Brown RL, MacKintosh FR, Zanjani ED. Evaluation of serum free culture conditions able to support the ex vivo expansion and engraftment of human hematopoietic stem cells in the human-to-sheep xenograft model. J Hematother Stem Cell Res. 2000;9(5):683–93.
- Jang YK, Jung DH, Jung MH, Kim DH, Yoo KH, Sung KW, et al. Mesenchymal stem cells feeder layer from human umbilical cord blood for ex vivo expanded growth and proliferation of hematopoietic progenitor cells. Ann Hematol. 2006;85(4):212–25.
- Bachier CR, Gokmen E, Teale J, Lanzkron S, Childs C, Franklin W, et al. Ex-vivo expansion of bone marrow progenitor cells for hematopoietic reconstitution following high dose chemotherapy for breast cancer. Exp Hematol. 1999;27(4):615–23.
- Weissman IL. Translating stem and progenitor cell biology to the clinic: barriers and opportunities. Science. 2000;287(5457):1442–6.
- Broxmeyer HE, Douglas GW, Hangoc G, Cooper S, Bard J, English D, et al. Human umbilical cord blood as a potential source of transplantable hematopoietic stem/progenitor cells. Proc Natl Acad Sci USA. 1989;86(10):3828.
- da Silva CL, Gonçalves R, Crapnell KB, Cabral JM, Zanjani ED, Almeida-Porada G. A human stromal-based serum-free culture system supports the ex vivo expansion/maintenance of bone marrow and cord blood hematopoietic stem/progenitor cells. Exp Hematol. 2005;33(7):828–35.
- Köhler T, Plettig R, Wetzstein W, Schaffer B, Ordemann R, Nagels HO, et al. Defining optimum conditions for the ex vivo expansion of human umbilical cord blood cells. Stem Cells. 1999;17(1):19–24.
- Ye ZQ, Burkholder JK, Qiu P, Schultz JC, Shahidi NT, Yang NS. Establishment of an adherent cell feeder layer from human umbilical cord blood for support of long-term hematopoietic progenitor cell growth. Proc Natl Acad Sci USA. 1994;91(25):12140–4.
- Xie CG, Wang JF, Xiang Y, Jia BB, Qiu LY, Wang LJ, et al. Huanga. Marrow mesenchymal stem cells transduced with TPO/FL genes as support for ex vivo expansion of hematopoietic stem/progenitor cells. Cell Mol Life Sci. 2005;62(21):2495–507.
- Xie CG, Wang JF, Xiang Y, Qiu LY, Jia BB, Wang LJ, et al. Cocultivation of umbilical cord blood CD34+ cells with retro-transduced hMSCs leads to effective amplification of long-term culture-initiating cells. World J Gastroenterol. 2006;12(3):393–402.
- Kögler G, Callejas J, Sorg RV, Fischer J, Migliaccio AR, Wernet P. The effect of different thawing Methods, growth factor combinations and media on the ex vivo expansion of umbilical cord blood primitive and committed progenitors. Bone Marrow Transplant. 1998;21(3):233–41.
- Verfaillie CM. Hematopoietic stem cells for transplantation. Nat Immunol. 2002;3(4):314–7.
- Alakel N, Jing D, Muller K, Bornhauser M, Ehninger G, Ordemann R. Direct contact with mesenchymal stromal cells affects migratory behavior and gene expression profile of CD133 hematopoietic stem cells during ex vivo expansion. Exp Hematol. 2009;37(4):504–13.
- Wilson A, Trumpp A. Bone-marrow haematopoietic-stem-cell niches. Nat Rev Immunol. 2006;6(2):93–106.
- Gottschling S, Saffrich R, Seckinger A, Krause U, Horsch K, Miesala K, et al. Human mesenchymal stromal cells regulate initial self-renewing divisions of hematopoietic progenitor cells by a beta1-integrin-dependent mechanism. Stem Cells. 2007;25(3):798–806.
- Wagner W, Wein F, Roderburg C, Saffrich R, Faber A, Krause U, et al. Adhesion of hematopoietic progenitor cells to human mesenchymal stem cells as a model for cell–cell interaction. Exp Hematol. 2007;35(2):314–25.
- Leary SC, Winge DR. The Janus face of copper: it's expanding roles in biology and the pathophysiology of disease. EMBO Rep. 2007;8(3):224–7.
- Chen CT, Shih YR, Kuo TK, Lee OK, Wei YH. Coordinated changes of mitochondrial biogenesis and antioxidant enzymes during osteogenic differentiation of human mesenchymal stem cells. Stem Cells. 2008;26(4):960–8.
- Ito K, Hirao A, Arai F, Matsuoka S, Takubo K, Hamaguchi K, et al. Regulation of oxidative stress by ATM is required for self-renewal of haematopoietic stem cells. Nature. 2004;431:997–1002.