Abstract
Tunable, battery free light emission is demonstrated in a solid state device that is compatible with lab on a chip technology and easily fabricated via solution processing techniques. A porous one dimensional (1D) photonic crystal (also called Bragg stack or mirror) is infiltrated by chemiluminescence (CL) rubrene-based reagents. The Bragg mirror has been designed to have the photonic band gap overlapping with the emission spectrum of rubrene. The CL reaction occurs in the pores of the photonic crystal and the emission spectrum of the dye is modulated according to the photonic band gap position. This is a compact, powerless emitting source that can be exploited in disposable photonic chips for sensing and point of care applications.
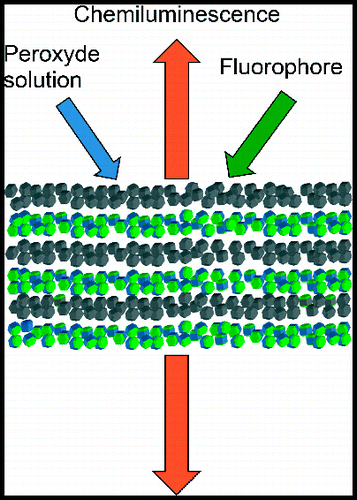
Introduction
The emission of electromagnetic radiation via release of energy in a chemical reaction is called chemiluminescence (CL).Citation1,Citation2 The steps of the light generating process are shown in .Citation3
In reaction (1), an oxalic acid derivative reacts with hydrogenperoxide, resulting in an intermediate highly energetic molecular species. In reaction (2), the intermediate transfers its energy to an acceptor fluorophore, which becomes electronically excited and emits its energy in form of light, as sketched in .
In opto-chips, where light signals are generated by integrated sources such as LEDs and lasers, a substantial fraction of the energy consumed is employed for powering the emitter. The exploitation of CL can lead to a consistent reduction of the electrical energy consumption, since CL is self-sustained and it does not require an external power source. In general, a battery free light emitter can be used in a manifold of applications such as lab on a chip and other integrated photonic circuits, highly sensitive portable sensors, new concept displays, and integrated communication boards. On the other hand, the main drawbacks of CL are the problematic confinement in space of the reaction and its low quantum efficiency. For this reasons, many research groups are developing novel CL methods, acting on the reaction conditions, changing reactants or adding catalysts.Citation4–Citation9 A better way to approach the problem would however be to exploit different mechanisms of excitation and process control, not only from a chemical point of view. An example still to be consolidated is the exploitation of plasmonic effects,Citation10 while the use of optical confinement in photonic crystals seems the most promising.Citation11,Citation12 In the last years, porous one-dimensional (1D) photonic crystals (stacks of alternated porous layers, also called Bragg mirrorsCitation13–Citation21) have received growing attention in photonics. In addition to a high-efficient photonic band gap (i.e. with the transmission that tends to zero),Citation22 they have the additional feature of porosity, as obtained by using proper materials and fabrication processes.Citation23,Citation24 This allows the infiltration of active chromophores that brings about a number of new functions such as optical sensing,Citation25–Citation29 enhanced light confinement,Citation30 lasing,Citation31–Citation33 optical switching,Citation34,Citation35 and enhanced light harvesting.Citation36–Citation38
In this work, we demonstrate CL emission modulation by the employment of nanoparticle-based porous 1D Bragg mirrors. These 1D photonic crystals have been infiltrated with the standard reagents of the CL solution. The CL occurs within the photonic crystal and the emission spectrum of the dye is thus modulated by the photonic band gap of the structure. In this way, one can combine and separately tune the optical properties of the structure (via its fabrication parameters) and of the chemical process (via the choice of the reactants and of the fluorophore). Encounter, intermediate formation and radiactive decay take place in the confined micro environment defined by the cavities in the porous medium: this approach is thus highly preferable for integration in solid state devices as photonic crystals technology is well established.
Authors have employed two different types of fabrication for the Bragg mirrors, i.e. spin coating and pulse laser deposition, to demonstrate the possibility of an easy integration of a chemiluminescent process in a layered photonic structure. Our application is thus a substantial upgrade of an existing photonic component that allows for a reduction in the energy consumption of the device in which it is integrated, without losing other established features. This is a step toward a powerless lab on a chip.
Experimental details
The two CL reagent solutions used in the experiment were prepared accordingly to the general procedure described in the original patent.Citation39 The first solution was made by mixing 9 mg of the rubrene fluorophore and 90 mg of bis(2,4,6 trichlorophenyl)oxalate in a volumetric glass flask, then filling with ethyl acetate to a volume of 20 mL. The second solution was made by filling a volumetric glass flask containing 480 mg of sodium salicylate and 10 mL of 30% hydrogen peroxide solution to a volume of 20 mL using methanol. All components were purchased from Sigma–Aldrich.
In preparation for our experiment, a seven bilayers (i.e. 14 layers) Bragg mirror was fabricated by means of a spin-coating technique. The starting materials employed being a colloidal solution of silica particles with an average size of 10–15 nm (Sigma Aldrich Ludox SM-30), further diluted in water to a concentration of 5% in weight, and a solution of titanium dioxide nanoparticles with an average size of 20 nm, obtained by diluting in ethanol a commercially available paste (Dyesol 18 nr-t) to a 1: 9 weight ratio. The crystal was therefore made by alternated deposition of the solutions on a glass substrate, previously cleaned in acetone and isopropanol sonic baths lasting approximately 10 min each. Every deposition was followed by a spinning of the substrate at a speed of 3800 rpm for 60 s using a Laurell WS-400-6NPP-Lite spin coater, and by drying in air at a temperature of 350°C for 20 min. Moreover, two eight bilayer (i.e. 16 layers) Bragg mirrors were fabricated by pulse laser deposition, which allows to obtain different layer (in our case of titanium dioxide) with different porosity, resulting in a significant refractive index contrast. The detailed experimental procedure has been published elsewhere.Citation40
The optical characterization of the Bragg stacks has been performed by using a tungsten lamp and concave grating spectrometer, acquired from Stellarnet (spectral resolution 1.5 nm). Authors used a linearly polarized light for the optical characterization of the photonic structure (even if we have observed that, in our experiments, light polarization has not significantly affected the optical properties of the structure).
Results and discussion
A schematic of the CL reaction in the porous photonic crystal is shown in . With two syringes we have infiltrated the peroxide solution and the fluorophore-containing one, prepared as described previously, in the pores formed between the nanoparticles composing the layers of the photonic crystal. The two solutions, after a percolation along the pore network, coalesce resulting in a bright CL, with a rise time in the range 0.5–1.5 s and a duration of few minutes.
Figure 2. Schematic of the chemiluminescence (CL) reaction in the porous one-dimensional (1D) photonic crystal. The CL reagents are infiltrated at the same time in the porous structure. The CL spectrum will be modulated by the photonic structure
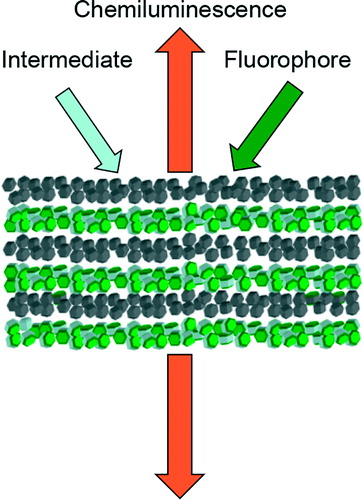
The fabricated photonic crystals show band gaps at about 550 nm. The spectral position of the photonic band gap is owing to the thicknesses of the layers and their effective refractive indexes. The effective refractive index in the bare photonic crystal is related to the refractive indexes of metal oxide and air, while in the infiltrated photonic crystal the effective refractive index is influenced by the filling of the pores with the CL reagent solutions. For this reason, the infiltrated photonic crystals show a red shift of 40–45 nm of the photonic band gap with respect to the one of the bare photonic crystal ().
Figure 3. Transmission spectrum of the porous one-dimensional (1D) photonic crystal (PhC) before and after infiltration of the chemiluminescence (CL) reagents. Authors observe a shift of 40–45 nm with infiltration, owing to the change of the effective refractive index of the photonic crystal layers
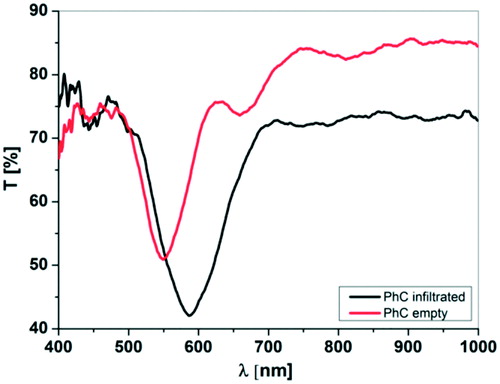
Chemiluminescence, as realized in different photonic crystals, is shown in . In the Figure, the blue dashed curve represented the typical CL spectrum of the rubrene fluorophore, while the red curve indicates the modulated CL in the porous photonic structures. The photonic crystals display different photonic band gaps (in terms of spectral position), resulting in a diverse modulation of the fluorophore emission (the position of the photonic band gap is determined, following the Bragg–Snell law,Citation19 by the refractive index of the employed materials and thickness of the layers). In the CL is modulated by a nanoparticle-based photonic crystal, fabricated with spin coating technique, while in the CL is modulated by two porous photonic crystals with alternated layers of different porosity, made by pulse laser deposition.
Figure 4. Chemiluminescence (CL) emission with rubrene filtrated by the one-dimensional porous photonic crystal made by (a) spin coating technique and (b) and (c) by pulsed laser deposition with two different porosities of the layers. The black curves show the transmission spectra of the photonic crystals. The blue dashed curves depict the CL spectrum of rubrene, while the red curves represent the CL spectra of rubrene with the CL reagents infiltrated in the porous photonic crystals
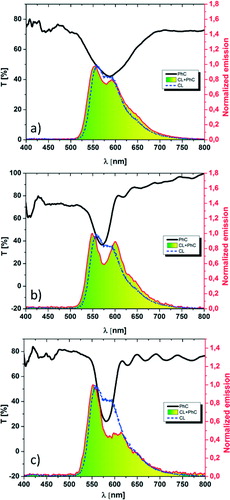
The photonic crystals, if properly designed, and with a high quality factor, can provide a feedback to the CL intensity. This can be obtained by fabricating very high quality photonic crystals by optimizing the fabrication procedure and by matching the maximum of the emission of the chosen dye with one of the edges of the photonic band gap, exploiting the high photon density of states in such spectral regions.Citation31–Citation33 The procedure can result in a chemiluminscence enhancement, as it was already demonstrated for opals.Citation41
Conclusions
In this paper, we have demonstrated CL emission in a porous 1D Bragg mirror. This was achieved by infiltrating the photonic structure with the CL reagents. The CL spectrum is modulated by the photonic band gap, providing a handle for spectral tuning of the emission. The proposed system provides efficient emission without an electrical bias or an external pumping light source. This paves the way for the fabrication of portable battery-free light sources, displays active pixels and microfluidic chips for sensing applications. A possible lab on a chip could employ the integration of microchannels that inject the CL reagents in the porous Bragg mirrors.
Acknowledgments
Francesco Scotognella acknowledges financial support from Italian Ministry of University and Research (project PRIN 2010-2011 ‘DSSCX’, Contract 20104XET32).
References
- Kricka L. J.: ‘Chemiluminescence and bioluminescence’, Anal. Chem., 1995, 67, 499–502.
- Roda A. and Guardigli M.: ‘Analytical chemiluminescence and bioluminescence: latest achievements and new horizons’, Anal. Bioanal. Chem., 2012, 402, 69–76.
- Garcia-Campana A. M.: ‘Chemiluminescence in analytical chemistry’; 2001, New York, CRC Press.
- Dodeigne C., Thunus L. and Lejeune R.: ‘Chemiluminescence as diagnostic tool. A review’, Talanta, 2000, 51, 415–439.
- Li X., Sun L., Ge A. and Guo Y.: ‘Enhanced chemiluminescence detection of thrombin based on cerium oxide nanoparticles’, Chem. Commun., 2000, 47, 947–949.
- Wang Z., Liu F. and Lu C.: ‘Mg-Al-carbonate layered double hydroxides as a novel catalyst of luminol chemiluminescence’, Chem. Commun., 2011, 47, 5479–5481.
- Huang Y., Zhao S., Liu Y. -M., Chen J., Chen Z. -F., Shi M. and Liang H.: ‘An amplified single-walled carbon nanotube-mediated chemiluminescence turn-on sensing platform for ultrasensitive DNA detection’, Chem. Commun., 2012, 48, 9400–9402.
- Yang L., Guan G., Wang S. and Zhang Z.: ‘Nano-anatase-enhanced peroxyoxalate chemiluminescence and its sensing application’, J. Phys. Chem. C, 2012, 116, 3356–3362.
- Lin Z., Xue W., Chen H. and Lin J. -M.: ‘Classical oxidant induced chemiluminescence of fluorescent carbon dots’, Chem. Commun., 2012, 48, 1051–1053.
- Aslan K. and Geddes C. D.: ‘Metal-enhanced chemiluminescence: advanced chemiluminescence concepts for the 21st century’, Chem. Soc. Rev., 2009, 38, 2556–2564.
- Yablonovitch E.: ‘Inhibited spontaneous emission in solid-state physics and electronics’, Phys. Rev. Lett., 1987, 58, 2059–2062.
- John S.: ‘Strong localization of photons in certain disordered dielectric superlattices’, Phys. Rev. Lett., 1987, 58, 2486–2489.
- Joannopoulos J. D., Johnson S. G., Winn J. N. and Meade R. D.: ‘Photonic crystals: molding the flow of light’; 2008, Princeton, Princeton University Press.
- Yoshino K., Tatsuhara S., Kawagishi Y., Ozaki M., Zakhidov A. A. and Vardeny Z. V.: ‘Amplified spontaneous emission and lasing in conducting polymers and fluorescent dyes in opals as photonic crystals’, Appl. Phys. Lett., 1999, 74, 2590–2592.
- Painter O., Lee R. K., Scherer A., Yariv A., O'Brien J. D., Dapkus P. D. and Kim I.: ‘Two-dimensional photonic band-gap defect mode laser’, Science, 1999, 284, 1819–1821.
- Criante L., Lucchetta D. E., Vita F., Castagna R. and Simoni F.: ‘Distributed feedback all-organic microlaser based on holographic polymer dispersed liquid crystals’, Appl. Phys. Lett., 2009, 94, 111114.
- Mekis A., Chen J., Kurland I., Fan S., Villeneuve P. and Joannopoulos J.: ‘High transmission through sharp bends in photonic crystal waveguides’, Phys. Rev. Lett., 1996, 77, 3787–3790.
- Serbin J. and Gu M.: ‘Experimental evidence for superprism effects in three-dimensional polymer photonic crystals’, Adv. Mater., 2006, 18, 221–224.
- Morandi V., Marabelli F., Amendola V., Meneghetti M. and Comoretto D.: ‘Colloidal photonic crystals doped with gold nanoparticles: spectroscopy and optical switching properties’, Adv. Funct. Mater., 2007, 17, 2779–2786.
- Busch K.: ‘Photonic crystals advances in design, fabrication, and characterization’; 2004, Weinheim, John Wiley & Sons.
- Valligatla S., Chiasera A., Varas S., Bazzanella N., Rao D. N., Righini G. C. and Ferrari M.: ‘High quality factor 1-D Er3+-activated dielectric microcavity fabricated by RF-sputtering’, Opt. Express, 2012, 20, 21214–21222.
- Born M. and Wolf E.: ‘Principles of optics: electromagnetic theory of propagation, interference and diffraction of light’; 2000, London, Cambridge University Press.
- Bonifacio L. D., Lotsch B. V., Puzzo D. P., Scotognella F. and Ozin G. A.: ‘Stacking the nanochemistry deck: structural and compositional diversity in one-dimensional photonic crystals’, Adv. Mater., 2009, 21, 1641–1646.
- Puzzo D. P., Bonifacio L. D., Oreopoulos J., Yip C. M., Manners I. and Ozin G. A.: ‘Color from colorless nanomaterials: bragg reflectors made of nanoparticles’, J. Mater. Chem., 2009, 19, 3500–5506.
- Bonifacio L. D., Puzzo D. P., Breslav S., Willey B. M., McGeer A. and Ozin G. A.: ‘Towards the photonic nose: a novel platform for molecule and bacteria identification’, Adv. Mater., 2010, 22, 1351–1354.
- Bonifacio L. D., Ozin G. A. and Arsenault A. C.: ‘Photonic nose-sensor platform for water and food quality control’, Small, 2011, 7, 3153–3157.
- Pavlichenko I., Exner A. T., Guehl M., Lugli P., Scarpa G. and Lotsch B. V.: ‘Humidity-enhanced thermally tunable TiO2/SiO2 bragg stacks’, J. Phys. Chem. C, 2011, 116, 298–305.
- Exner A. T., Pavlichenko I., Lotsch B. V., Scarpa G. and Lugli P.: ‘Low-cost thermo-optic imaging sensors: a detection principle based on tunable one-dimensional photonic crystals’, ACS Appl. Mater. Interfaces, 2013, 5, 1575–1582.
- Chiappini A., Armellini C., Carpentiero A., Minati L., Righini G. C. and Ferrari M.: ‘Solvent sensitive polymer composite structures’, Opt. Mater., 2013, 36, 130–134.
- Puzzo D. P., Helander M. G., O'Brien P. G., Wang Z., Soheilnia N., Kherani N., Lu Z. and Ozin G. A.: ‘Organic light-emitting diode microcavities from transparent conducting metal oxide photonic crystals’, Nano Lett., 2011, 11, 1457–1462.
- Scotognella F., Puzzo D. P., Zavelani-Rossi M., Clark J., Sebastian M., Ozin G. A. and Lanzani G.: ‘Two-photon poly(phenylenevinylene) DFB laser’, Chem. Mater., 2011, 23, 805–809.
- Scotognella F., Puzzo D. P., Monguzzi A., Wiersma D. S., Maschke D., Tubino R. and Ozin G. A.: ‘Nanoparticle one-dimensional photonic-crystal dye laser’, Small, 2009, 5, 2048–2052.
- Puzzo D. P., Scotognella F., Zavelani-Rossi M., Sebastian M., Lough A. J., Manners I., Lanzani G., Tubino R. and Ozin G. A.: ‘Distributed feedback lasing from a composite poly(phenylene vinylene)-nanoparticle one-dimensional photonic crystal’, Nano Lett., 2009, 9, 4273–4278.
- Criante L. and Scotognella F.: ‘Low-voltage tuning in a nanoparticle/liquid crystal photonic structure’, J. Phys. Chem. C, 2012, 116, 21572–21576.
- Criante L. and Scotognella F.: ‘Infiltration of E7 liquid crystal in a nanoparticle-based multilayer photonic crystal: fabrication and electro-optical characterization’, Mol. Cryst. Liq. Cryst., 2013, 572, 31–39.
- Colodrero S., Mihi A., Anta J. A., Ocaña M. and Míguez H.: ‘Experimental demonstration of the mechanism of light harvesting enhancement in photonic-crystal-based dye-sensitized solar cells’, J. Phys. Chem. C, 2009, 113, 1150–1154.
- Lozano G., Colodrero S., Caulier O., Calvo M. E. and Míguez H.: ‘Theoretical analysis of the performance of one-dimensional photonic crystal-based dye-sensitized solar cells’, J. Phys. Chem. C, 2010, 114, 3681–3687.
- Colodrero S., Forneli A., ópez-López C. L., Pellejà L., Míguez H. and Palomares E.: ‘Efficient transparent thin dye solar cells based on highly porous 1D photonic crystals’, Adv. Funct. Mater., 2012, 22, 1303–1310.
- Chopdekar V. M., Schleck J. R., Guo C. and Hall A. J.: ‘Two-component chemiluminescent composition’, US Patent 5597517, 1997, published 28 January.
- Passoni L., Criante L., Fumagalli F., Scotognella F., Lanzani G. and Di Fonzo F.: ‘Self-assembled hierarchical nanostructures for high-efficiency porous photonic crystals’, ACS Nano, 2014, 8, 12167–12174.
- Shi X., Li M., Ye C., Shen W., Wen Y., Chen L., Yang Q., Shi L., Jiang L. and Song Y.: ‘Photonic crystal boosted chemiluminescence reaction’, Laser Photonics Rev., 2013, 7, L39–L43.