Abstract
Genetic analysis is providing new information on the biological basis of epilepsy at a rapid pace; this article identifies factors acting as major barriers to use of these data for therapy development. Disease heterogeneity is a primary obstacle since so many genes can cause or predispose to epilepsy and the clinical presentation of epilepsy is so diverse, thus making it difficult to define the most therapeutically relevant targets. Further, many epilepsy genes affect brain development, an observation that represents a barrier unto itself given the challenge of reversing or preventing genetically mediated alterations of brain pathway formation. Finally, the lack of appropriate models for testing new therapies is also recognized as a fundamental limitation. Overcoming these barriers will be aided by full characterization of the genetic landscape of epilepsy, elucidation of key pathway points for therapeutic intervention and creation of unique experimental models to validate results.
Epilepsy is one of the most common brain diseases and one for which a wide range of pharmacotherapeutic agents are currently being marketed worldwide. Nonetheless, many epilepsy patients do not achieve adequate seizure control with available medications and new treatments are needed urgently. A strong effort has been put forth to identify novel targets and develop new anti-epilepsy drugs, but despite a marked increase in the number of therapeutic agents that have been introduced onto the market over the past several decades, there has not been an appreciable change in the proportion of epilepsy patients whose seizures are refractory to drug treatment.[Citation1] Advancements in molecular genetic techniques have begun to more fully characterize the genetic architecture of epilepsy by identifying new genes that cause or contribute to the onset of epilepsy and it has been anticipated that such discoveries would lead to the development of novel drugs or other more personalized therapeutic strategies and permit successful treatment of a larger fraction of epilepsy patients. Such progress has unfortunately not been realized. This article attempts to shed light on some major factors that represent barriers to the use of genetic information for the development of new treatments for epilepsy.
Genetic heterogeneity
Studies of experimental animal models and human studies document that a multitude of factors, both biological and environmental, underlie the expression of seizures and that seizure characteristics may be highly variable from individual to individual. Animal models have been most useful due to the ability to manipulate gene sequences and gene expression levels [Citation2] and studies have led to the concept that hundreds of genes, when mutated appropriately, may cause epilepsy-related phenotypes. Biological (i.e., genetic) factors in human epilepsy are discovered primarily by cloning gene mutations in DNA from members of rare families in which the disease segregates as a Mendelian trait, or by conducting genetic association studies in large populations of unrelated patients and controls. Variation in gene copy number has also been documented to be a source of genetic risk for epilepsy.[Citation3] Most recently, the use of advanced DNA sequencing methods to analyze the genome of sporadic cases of epilepsy, particularly cases of epileptic encephalopathies, has led to results suggesting that single locus causes of epilepsy are more frequent than previously recognized,[Citation4] thus challenging the hypothesis that polygenic mechanisms are responsible for disease in the vast majority of epilepsy patients. The defects identified in these studies circumscribe an even broader set of genes and gene classes with regard to the pathogenesis of epilepsy, and several unifying concepts that could lead to therapeutic innovation have emerged from this research. These concepts move beyond the perspective of epilepsies as ion channelopathies [Citation5] and invoke mechanisms related to processes such as neuronal migration [Citation6] and cellular energy metabolism.[Citation7] The focus on dysregulation of developmental gene expression pathways has been intensified given the number of transcription factor gene mutations that have been linked to rare epilepsy-related syndromes such as tuberous sclerosis, focal cortical dysplasia, hemimegalencephaly and ganglioglioma, conditions that are grouped under the term “mTORopathies” since they are caused by alterations of the mTOR (mammalian target of rapamycin) signaling pathway.[Citation8] Importantly, this work has encouraged clinical trials of mTOR inhibitors such as rapamycin and everolimus in epilepsy patients with tuberous sclerosis although to date the results of these studies have been equivocal.
Genome-wide association studies
Despite the recently accelerated pace of discovery of gene mutations in epileptic encephalopathies and related rare monogenic forms of epilepsy, it is still not clear to what extent these findings are relevant to the more common genetically complex (i.e., polygenic) epilepsies. Discovery and confirmation of genetic variants that have but partial effects on the development of epilepsy is much more challenging, and few crucial leads for new therapies have emerged from the many targeted genetic association studies that have been performed. Over the past several years, a series of genome-wide association studies (GWAS) of epilepsy have been published [Citation9–Citation12]; however, the GWAS results have been disappointing with only several convincing susceptibility variants reported including variants of SCN1A, a gene well documented to cause monogenic epilepsies. Failure to uncover more epilepsy-risk gene variants via GWAS, even when using meta-analyses to combine extant data sets,[Citation12] suggests that common genetic variation does not play a major role in the development of epilepsy or that risk comes from a large number of variants each with a weak effect on clinical phenotype. Thus, it is possible that the low yield of significant GWAS findings is primarily a statistical problem since to date the number of epilepsy patients analyzed by GWAS is modest compared to the numbers of patients included in GWAS of other common brain diseases such as schizophrenia. Increasing the size of the epilepsy population in future GWAS will permit reliable detection of variants that exert individually small phenotypic effects and lead to identification of novel genes and pathways as potential targets for therapeutic intervention. The relative dearth of epilepsy risk alleles reported so far in GWAS may also be related to the great clinical heterogeneity characteristic of the disease and it is possible that future studies aimed at refining phenotypic measures will lead to the identification of additional genetic influences as well. The relatively weak GWAS results notwithstanding, it is clear that the genetic heterogeneity associated with epilepsy is staggering, with a wide range of genes and genetic variation already defined.[Citation13] This circumstance is both a help and a hindrance with regard to new therapy development as the abundance of drug targets offers many research opportunities but simultaneously may dilute focus and mask critical findings.
Elephant in the room: SCN1A
Of all the genetic factors that have been identified as playing a role in epilepsy, SCN1A has accumulated the greatest amount of support. As the gene that encodes the major functional component of the NaV1.1 sodium channel, its expression controls the generation of action potentials and regulates neuronal excitability throughout the brain. The first epilepsy-causing SCN1A mutations were reported in GEFS+ and severe myoclonic epilepsy of infancy [Citation14] and have subsequently been discovered in other monogenic forms of epilepsy as well.[Citation15] At the same time, GWAS has documented that common SCN1A variants increase risk for common epilepsies.[Citation12] Discovery of epilepsy-causing and epilepsy-risk variants of SCN1A is not surprising given what is known about the pharmacology of many anti-epilepsy drugs. Thus, mainstay treatments such as phenytoin, carbamazepine and lamotrigine all act to reduce the activity of SCN1A-containing sodium channels.[Citation16] This observation underscores the importance of sodium channels as therapeutic targets in epilepsy and suggests that SCN1A-containing sodium channels are a final common pathway for the expression of seizures. Importantly, both loss-of-function and gain-of-function mutations in SCN1A can cause epilepsy,[Citation17] a finding that poses an obstacle to creating more effective SCN1A-interacting drugs. Better understanding of relationships between causative SCN1A mutations and SCN1A risk variants may allow the refinement of biophysical drug characteristics required to appropriately modulate SCN1A function in individual patients.
Developmental abnormalities
Various lines of evidence have documented that alteration of normal brain development can result in epilepsy. The discovery of epilepsy-causing mutations in genes coding for trophic factors, transcription factors and cell adhesion molecules are consistent with proposed neural network defects.[Citation18] Like other neurodevelopmental diseases, epilepsy may be considered to involve inappropriate connections between individual neurons and/or groups of neurons that become established early in life. Once genetic defects cause aberrant connections to form, and recurrent seizures begin to be expressed, successful rewiring through drug or gene therapy will be difficult to achieve. Pre- or postnatal screening could potentially identify developmental gene defects at time points early enough to institute restorative gene therapy. However, this would require full elucidation of the genetic architecture of epilepsy and refinement of techniques for delivering replacement genes to the brain, goals that are still in the distance. In the near term, therapeutic approaches most likely to be successful in developmental epilepsies are those which ameliorate seizures, the predominant symptom of the disease.
Validation models
The process used to develop new treatments for disease relies upon experimental validation in model systems. In the case of the epilepsies, the establishment of models has itself been hindered by clinical and genetic heterogeneity. In fact, due to the numerous ways in which recurrent seizures can arise and the different types of seizures that can manifest, epilepsy may be considered as a group of diseases, each one potentially requiring a different model and strategy for development of maximally effective treatment. Until now, anti-epilepsy drugs have been devised primarily through work in rodents using seizure models such those involving electroconvulsive shock, kindling or chemoconvulsant drugs.[Citation19] Although the utility of acute seizure tests is proven by the efficacy of the drugs that have been introduced into clinical practice and that represent the current standard of care for treating epilepsy, they are not germane to all forms of epilepsy and cannot be used to evaluate therapies intended to cure or prevent epilepsy. Expansion of rodent models to include genetic mutants with spontaneous seizure phenotypes and humanized rodents engineered genetically to harbor mutations discovered in patients with epilepsy would help to optimize new therapy development. Until that time, potential new treatments will continue to rely on the use of models that are variably relevant to the broad spectrum of epilepsies.
Breaking down the barriers
Collection of genetic information required to characterize epilepsy is increasing at a rapid pace, but it is far from complete. In order to optimize the value of epilepsy genetic data for treatment development, the full comport of genetic influences on epilepsy, including factors that simply increase risk in addition to frank causative factors, must be established. Elucidating the impact of epigenetics is also critical. Doing so will identify genes and gene networks that may converge to allow unification of apparently disparate discoveries and lead to formulation of new therapeutic strategies. Some of these strategies may have wide potential applicability depending upon the mechanism and its relation to final common pathways of seizure activity. The numerous available anti-epilepsy drugs that interact with SCN1A proteins reflect the key position of sodium channels in seizure pathways and underscore their value as anti-epilepsy drug targets. Identification of other common seizure pathways would be transformative in leading to new pharmacotherapeutics for epilepsy. One molecule that may represent an alternative common final pathway for seizures is Kir4.1, an inward rectifier potassium channel subunit that mediates regulation of extracellular potassium levels.[Citation20] Identification and refinement of chemical compounds that can activate this protein is underway currently [Citation21] and may result in a new class of anti-epilepsy drugs. The mTOR cascade may represent another such final common pathway that is ‘drugable’.
Apart from the major fraction of epilepsy patients whose disease is multifactorial in nature, breakthroughs in treating monogenic epilepsies may be fundamentally different and emerge largely on the basis of the characterization of specific genetic defects. Such approaches would not be specific to treating monogenic epilepsy but rather could be applicable to any monogenic disease. Examples include anti-sense knockdown of aberrantly spliced transcripts or gene duplication events, drug-mediated transcriptional read-through for point mutations that create premature stop codons and gene replacement with viral vectors for deletions.[Citation22] These are all strategies that are being investigated intensively, but which still require substantial refinement prior to use as therapeutic interventions.
Beyond the discovery of genetic variants in epilepsy patients, refined rodent or other animal models must be established and validated for different types of epilepsy both to document the phenotypic effect of variants as well as to evaluate treatments. Experimental models will be especially crucial for elucidating mechanisms of genetically programmed developmental phenomena that lead to epilepsy. Ultimately, there is hope that a combination of comprehensive genomics, bioinformatics and unique model systems can be integrated to generate new ideas that will begin to break down the barriers that currently hinder development of the next generation of therapies for patients with epilepsy.
Financial & competing interests disclosure
The author has no relevant affiliations or financial involvement with any organization or entity with a financial interest in or financial conflict with the subject matter or materials discussed in the manuscript. This includes employment, consultancies, honoraria, stock ownership or options, expert testimony, grants or patents received or pending, or royalties.
Additional information
Notes on contributors
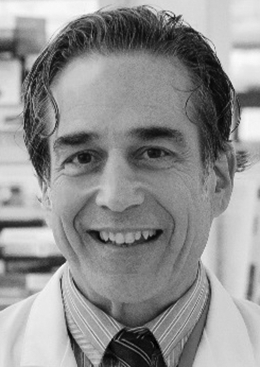
Thomas N. Ferraro
References
- Miziak B, Chrościńska-Krawczyk M, Błaszczyk B, et al. Novel approaches to anticonvulsant drug discovery. Expert Opin Drug Discov. 2013;8(11):1415–1427. doi:10.1517/17460441.2013.837047. Epub2013 Sep 19.
- Frankel WN. Genetics of complex neurological disease: challenges and opportunities for modeling epilepsy in mice and rats. Trends Genet. 2009;25(8):361–367. doi:10.1016/j.tig.2009.07.001. Epub2009 Aug 6.
- Olson H, Shen Y, Avallone J, et al. Copy number variation plays an important role in clinical epilepsy. Ann Neurol. 2014;75(6):943–958. doi:10.1002/ana.24178. Epub2014 Jun 13.
- Mercimek-Mahmutoglu S, Patel J, Cordeiro D, et al. Diagnostic yield of genetic testing in epileptic encephalopathy in childhood. Epilepsia. 2015;56(5):707–716. doi:10.1111/epi.12954. Epub2015 Mar 25.
- Moulard B, Picard F, Le Hellard S, et al. Ion channel variation causes epilepsies. Brain Res Brain Res Rev. 2001;36(2–3):275–284.
- Liu JS. Molecular genetics of neuronal migration disorders. Curr Neurol Neurosci Rep. 2011;11(2):171–178. doi:10.1007/s11910-010-0176-5.
- Otáhal J, Folbergrová J, Kovacs R, et al. Epileptic focus and alteration of metabolism. Int Rev Neurobiol. 2014;114:209–243. doi:10.1016/B978-0-12-418693-4.00009-1.
- Curatolo P1, Moavero R. mTOR inhibitors as a new therapeutic option for epilepsy. Expert Rev Neurother. 2013;13(6):627–638. doi:10.1586/ern.13.49.
- Kasperaviciūte D, Catarino CB, Heinzen EL, et al. Common genetic variation and susceptibility to partial epilepsies: a genome-wide association study. Brain. 2010;133(Pt 7):2136–2147. doi:10.1093/brain/awq130. Epub2010 Jun 3.
- Guo Y, Baum LW, Sham PC, et al. Two-stage genome-wide association study identifies variants in CAMSAP1L1 as susceptibility loci for epilepsy in Chinese. Hum Mol Genet. 2012;21(5):1184–1189. doi:10.1093/hmg/ddr550. Epub2011 Nov 24.
- EPICURE Consortium; EMINet Consortium, Steffens M, Leu C, Ruppert AK, et al. Genome-wide association analysis of genetic generalized epilepsies implicates susceptibility loci at 1q43, 2p16.1, 2q22.3 and 17q21.32. Hum Mol Genet. 2012;21(24):5359–5372. doi:10.1093/hmg/dds373. Epub2012 Sep 4.
- International League Against Epilepsy Consortium on Complex Epilepsies. Genetic determinants of common epilepsies: a meta-analysis of genome-wide association studies. Lancet Neurol. 2014;13(9):893–903. doi:10.1016/S1474-4422(14)70171-1. Epub2014 Jul 30.
- Ferraro TN. The relationship between genes affecting the development of epilepsy and approaches to epilepsy therapy. Expert Rev Neurother. 2014;14(3):329–352. doi:10.1586/14737175.2014.888651.
- Lossin C, Rhodes TH, Desai RR, et al. Epilepsy-associated dysfunction in the voltage-gated neuronal sodium channel SCN1A. J Neurosci. 2003;23(36):11289–11295.
- Freilich ER, Jones JM, Gaillard WD, et al. Novel SCN1A mutation in a proband with malignant migrating partial seizures of infancy. Arch Neurol. 2011;68(5):665–671. doi:10.1001/archneurol.2011.98.
- Rogawski MA, Löscher W. The neurobiology of antiepileptic drugs. Nat Rev Neurosci. 2004;5(7):553–564.
- Rhodes TH, Lossin C, Vanoye CG, et al. Noninactivating voltage-gated sodium channels in severe myoclonic epilepsy of infancy. Proc Natl Acad Sci USA. 2004;101(30):11147–11152. Epub 2004 Jul 19.
- Yaffe RB, Borger P, Megevand P, et al. Physiology of functional and effective networks in epilepsy. Clin Neurophysiol. 2015;126(2):227–236. doi:10.1016/j.clinph.2014.09.009. Epub2014 Sep 22.
- Yuen ES, Trocóniz IF. Can pentylenetetrazole and maximal electroshock rodent seizure models quantitatively predict antiepileptic efficacy in humans? Seizure. 2015;24:21–27. doi:10.1016/j.seizure.2014.
- Sibille J, Dao Duc K, Holcman D, et al. The neuroglial potassium cycle during neurotransmission: role of Kir4.1 channels. PLoS Comput Biol. 2015;11(3):e1004137. eCollection 2015. doi:10.1371/journal.pcbi.1004137.
- Swale DR, Kharade SV, Denton JS, Cardiac and renal inward rectifier potassium channel pharmacology: emerging tools for integrative physiology and therapeutics. Curr Opin Pharmacol. 2014;15:7–15. doi:10.1016/j.coph.2013.11.002. Epub2013 Nov 26.
- Maguire CA, Ramirez SH, Merkel SF, et al. Gene therapy for the nervous system: challenges and new strategies. Neurotherapeutics. 2014;11(4):817–839. doi:10.1007/s13311-014-0299-5.