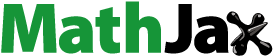
Abstract
Attention-deficit/hyperactivity disorder (ADHD) is the most frequently diagnosed neurodevelopmental disorder. The norepinephrine transporter (NET) inhibitor atomoxetine, the first nonstimulant drug licensed for ADHD treatment, also acts as an N-methyl-D-aspartate receptor (NMDAR) antagonist. The compound’s effects on gene expression and protein levels of NET and NMDAR subunits (1, 2A, and 2B) are unknown. Therefore, adolescent Sprague Dawley rats were treated with atomoxetine (3 mg/kg, intraperitoneal injection [ip]) or saline (0.9%, ip) for 21 consecutive days on postnatal days (PND) 21–41. In humans, atomoxetine’s earliest clinical therapeutic effects emerge after 2–3 weeks. Material from prefrontal cortex, striatum (STR), mesencephalon (MES), and hippocampus (HC) was analyzed either directly after treatment (PND 42) or 2 months after termination of treatment (PND 101) to assess the compound’s long-term effects. In rat brains analyzed immediately after treatment, protein analysis exhibited decreased levels of the NET in HC, and NMDAR subunit 2B in both STR and HC; the transcript levels were unaltered. In rat brains probed 2 months after final atomoxetine exposure, messenger RNA analysis also revealed significantly reduced levels of genes coding for NMDAR subunits in MES and STR. NMDAR protein levels were reduced in STR and HC. Furthermore, the levels of two SNARE (soluble N-ethylmaleimide-sensitive factor attachment protein receptor) proteins, synaptophysin and synaptosomal-associated protein 25, were also significantly altered in both treatment groups. This in vivo study detected atomoxetine’s effects beyond NET inhibition. Taken together, these data reveal that atomoxetine seems to decrease glutamatergic transmission in a brain region-specific manner. Long-term data show that the compound’s impact is not due to an acute pharmacological effect but lasts or even amplifies after a drug-free period of 2 months, leading to altered development of synaptic composition. These alterations might contribute to atomoxetine’s clinical effects in the treatment of ADHD, a neurodevelopmental disorder in which synaptic processes and especially a dysregulated glutamatergic metabolism seem to be involved.
Introduction
The pathogenesis of attention-deficit/hyperactivity disorder (ADHD), the most frequently diagnosed neuropsychiatric disorder in childhood with a prevalence of 5%–6%,Citation1 and the mechanisms of its psychopharmacological treatments are still far from being completely understood. Distinctly increased hyperactivity, pronounced inattention, and impulsivity not appropriate for age are the three cardinal symptoms of ADHD.Citation2,Citation3 ADHD often persists into adulthood; 1%–4% of adults seem to remain affected.Citation4 It is hypothesized that dysfunctional norepinephrinergic (NE) and dopaminergic signal processing within various brain areas, especially in the cortico-striatal-thalamic-cortical loop, give rise to an imbalanced state of arousal. This, in consequence, presumably leads to the noted ADHD symptoms.Citation5 To date, psychostimulants such as methylphenidate or amphetamine salts represent the first-line treatment to attenuate ADHD core symptoms.Citation6 Nonetheless, about 10%–20% of the patients show only limited benefit from stimulants,Citation7 whose major effects seem to depend on the inhibition of the striatal presynaptic dopamine transporter.Citation8,Citation9
A growing body of evidence indicates that alterations of the monoaminergic system alone are not the only primary pathophysiological cause for ADHD symptoms; dysfunctions of the glutamatergic neurotransmitter system also seem to play a major role in the pathophysiology of ADHD.Citation10,Citation11 Glutamate is the main excitatory neurotransmitter in the mammalian brain, and over 60% of all synapses are glutamatergic.Citation12 The glutamate receptors include the G-protein-coupled metabotropic glutamate receptors and the ionotropic cation-permeable receptor-channels: the kainate receptors, the AMPAR (α-amino-3-hydroxy-5-methyl-4-isoxazolepropionic acid receptors), and the NMDAR (N-methyl-D-aspartate receptors) with seven different subunits: NR1, NR2A–D and NR3A, and B.Citation13 The receptor subunits and their isoforms have unique biophysical properties and display a defined regional and developmental expression pattern. The NR2 subunits play a crucial role during brain development, controlling synaptic plasticity and memory function.Citation14 In the rat brain, NR2B-containing receptors contribute to long-term potentiation (LTP) during adolescence, whereas NR1/NR2A receptors mediate LTP in adults.Citation15 Grin2B (NR2B) is predominantly expressed during early developmental stages, and its expression is downregulated around the third postnatal week, whereas the subunit NR2A first appears around birth, dramatically increases thereafter, and peaks 3 weeks after birth.Citation16 NMDARs are not limited to the postsynaptic membrane. They were also identified at extra-, peri-, and presynaptic sites,Citation17–Citation20 where they display diverse physiological roles, eg, signaling through extrasynaptic NMDARs, which mainly contain the NR2B subunit, have inhibitory effects.Citation21 Recently, dysfunctions of the NMDARs have been linked to ADHD.Citation22 LTP is strongly mediated by the NMDARs containing the subunit 2A. The spontaneously hypertensive rat (SHR), the best-characterized ADHD animal model, produces NMDARs more frequently containing NR2B than NR2A.Citation22 Consequently, AMPAR insertion into the postsynaptic membrane is suppressed, which reduces LTP and synaptic plasticity.Citation23,Citation24 Although glutamate has a predominant role in synaptic plasticity, learning and memory, pathological concentrations are highly excitotoxic and lead to neuronal cell death.Citation12 Lou, in a 1996 study, already linked excessive glutamate release in the striatum (STR) to the onset of ADHD.Citation25
Atomoxetine, a selective NE transporter (NET) antagonist,Citation26–Citation28 is the first nonstimulant compound licensed for the treatment of ADHD in children, adolescents, and adults.Citation29 Current knowledge about atomoxetine’s cellular mechanisms of action is still limited. After oral application, increased intrasynaptic NE levels are detectable within hours in the nonhuman primate brain as shown by positron emission tomography (PET) with (S,S)-[18F] FMeNER-D2, a ligand to the NET.Citation30 A second PET study with the same ligand indicates that NET is occupied within 15 minutes after intravenous application of atomoxetine at intracerebral concentrations as low as 16 ng/mL measured in the thalamus. The authors conclude that clinical doses of atomoxetine occupy NET almost completely within 15 minutes.Citation31 Atomoxetine’s earliest therapeutic effects, however, only occur after 2–3 weeks of treatment.Citation32 Therefore, it seems to be very unlikely that the therapeutic effects are solely due to the NET inhibition. In addition, the recommended therapeutic plasma level is 200–1,000 ng/mL,Citation33 a concentration presumably leading to much higher brain levels than measured in the above mentioned PET study.Citation34
Few in vivo or in vitro studies have been conducted to investigate atomoxetine’s cellular and neurochemical effects.Citation35–Citation37 Furthermore, until now no study has characterized its long-term biological effects. The aim of the present study was to ascertain atomoxetine’s further cellular action beyond the inhibition of NET. Previously, we could show that atomoxetine acts as an NMDAR antagonist in clinically relevant doses in vitro.Citation38 Therefore, in the present study, we addressed the issue of whether atomoxetine also alters transcript and protein levels of the NMDAR subunits (NR1, NR2A, and NR2B) and NET. Additionally, we analyzed immediate and long-term effects of atomoxetine on the expression and protein levels of the mentioned NMDAR subunits and NET in the male adolescent brain.
Prefrontal cortex (PFC) and STR, as part of the cortico-striatal-thalamic-cortical circuit, and mesencephalon (MES) and hippocampus (HC), as parts of the limbic system and crucial for learning and memory,Citation22 were investigated separately. Doing so allowed us to detect possible brain region-specific effects.
Materials and methods
Animal housing
Crl:CD(SD) rats (Charles River Laboratories:Cesarean derived [Sprague Dawley]) for breeding were obtained from Charles River Laboratories (Wilmington, MA, USA) and housed in groups of two under controlled temperature (21°C±2°C), humidity (60%–65%), and a 12:12 hour light-dark cycle. Food and water were available ad libitum. Pregnant rats were housed separately, and pups were separated from the dams at postnatal day (PND) 21.
Treatment procedures
Male adolescent rats were treated from PND 21–42 and either analyzed immediately or housed for another 2 months off-drug and analyzed thereafter. The two groups were named “early treatment group” and “late treatment group”, respectively.
Atomoxetine hydrochloride (Sigma-Aldrich, St Louis, MO, USA) was dissolved in 0.9% saline (Fresenius Kabi AG, Bad Homburg, Germany) and was administered by intraperitoneal (ip) injection into rats (n=7–8) at a dose of 3 mg/kg daily dose. Control animals (n=7–8) were age-matched to atomoxetine treated rats (PND 21 days) and received 0.9% saline. Solutions were sterile filtered (0.2 μm). All animal experiments were approved by the Committee for Animal Experimentation of the University of Ulm and the regional administrative authority (Registration Number 944). All procedures were carried out in accordance with the European Communities Council Directive of November 24, 1986 (86/609/EEC).
Extraction of brain tissue
At the end of the treatment period, male adolescent rats were anesthetized with carbon dioxide and the brain was removed. The brain hemispheres were sagittally separated and the STR, MES, PFC, and HC were resected by microdissection. Brain maps from The Rat Brain: In Stereotactic Coordinates.Citation39 were used for orientation. All samples were snap-frozen in liquid nitrogen and stored at −80°C. All preparations were performed in cold sterile Hank’s Balanced Salt Solution (HBSS; PAA Laboratories GmbH, Pasching, Austria).
Extraction of total RNA/quantitative reverse transcription polymerase chain reaction (qRT-PCR)
Isolation of total RNA from frozen brain tissue was performed using the RNeasy kit (QIAGEN, Venlo, the Netherlands) as described by the manufacturer. The total RNA was eluted in 60 μL RNase-free water. For the reverse transcription (RT)-mediated PCR studies, first strand synthesis and qRT-PCR measurements were carried out in a one-step, single-tube format using the QIAGEN QuantiFast SYBR Green RT-PCR kit. The qRT-PCR analysis was performed using the Rotor-Gene® real-time PCR machine (QIAGEN). For each measurement, as much as 1 μL of undiluted total RNA was measured. RT conditions were as follows: 10 minutes at 50°C, 5 minutes at 95°C, followed by 40 cycles of PCR: 10 seconds at 95°C for denaturation, 30 seconds at 60°C for annealing and elongation. All measurements were run in duplicates. To ascertain primer specificity, melting curves were driven from 60°C ramping to 95°C in 1°C steps, while fluorescence was recorded continuously. The RT and PCR reactions were carried out using QIAGEN QuantiTect Primer Assay oligonucleotides amplifying the following transcripts: solute carrier family 6 member 2 (Slc6a2, QT00184604); glutamate receptor ionotropic N-methyl-D-aspartate 1 (Grin1, QT00182287); glutamate receptor ionotropic N-methyl-D-aspartate 2A (Grin2A, QT0037928); glutamate receptor ionotropic N-methyl-D-aspartate 2B (Grin2B, QT00184793); synaptophysin (Syp, QT02338056); and synaptosomal-associated protein 25 kDa (Snap25, QT00196413).
Analysis of qRT-PCR data
Cycle threshold for each run was set to 0.01. Transcript levels of the transcript of interest were normalized to messenger RNA (mRNA) levels of the 60 S ribosomal protein L13a (RPL13A), which was calculated to be the most robustly expressed housekeeping gene among six tested genes using a geometric averaging method (geNorm).Citation40,Citation41 Other genes tested include tyrosine 3-monooxygenase/tryptophan 5-monooxy-genase activation protein, zeta polypeptide, glyceraldehyde-3-phosphate dehydrogenase, cyclophilin A, beta-actin, hydroxymethylbilane synthase, RPL13A. Cycle threshold values (Ctv) were inserted in the following formula:
where VCTG is virtual concentration of the target gene (TG) transcripts, and VCHKG is virtual concentration of the housekeeping gene (HKG) transcripts. To normalize the expression of the TG to the HKG, ratios were calculated as,
where RCTG is relative concentration of the TG compared to the expression of the HKG. All oligonucleotides used in the qRT-PCR were purchased from QIAGEN and display ∼100% efficiency (personal communication with QIA-GEN). Therefore, the value of the “−slope” in the above mentioned formula was set to −3.33, which represents 100% efficiency.
Preparation of tissue lysates
Frozen brain tissues were lysed in 100 μL (STR, PFC) to 200 μL (MES, HC) of sodium dodecyl sulfate (SDS) sample buffer (37.8% glycerol, 6% SDS [2x crist], 2.27% Tris, 2% bromophenol blue solution [10 mg/mL], 0.15% dithioerythritol, pH 6.9). Crude lysates were passed through QIAGEN QIAshredder spin columns for 2 minutes at 16,060 rcf. Samples were denatured for 30 minutes at 70°C and stored at −80°C.
Determination of protein content/coomassie staining
Protein concentrations of brain lysates were assayed via the amido black staining method.Citation42 A calculated 15 μg of each sample collected from one brain region was subjected to the same 10% SDS-polyacrylamide gel electrophoresis. Equal loading was confirmed using total protein stains (GelCode Blue Stain Reagent; Thermo Fisher Scientific, Waltham, MA, USA).Citation43
Immunoblotting
A quantity of 15 μg of protein per sample was subjected to 10% SDS-polyacrylamide gel electrophoresis and separated as appropriate in buffer solution (960 mM glycine, 17 mM SDS, 125 mM Tris). Proteins were electrophoretically transferred (90 V for 105 minutes at 4°C) onto a methanol activated polyvinylidene fluoride membrane using a tank blot transfer system (Bio-Rad Laboratories, Hercules, CA, USA) flooded with buffer solution (195 mM glycine, 25 mM Tris, 20% methanol, 0.1% SDS). Blotted membranes were blocked in 5% fat-free milk dissolved in Tris buffered saline (150 mM NaCl, 50 mM Tris, pH 7.6) supplemented with 0.05% Tween 20 (TBST) for 1.5 hours. Membranes were incubated with primary antibodies diluted in blocking solution for 1–1.5 hours. The following primary antibodies were used: rabbit polyclonal antibody against the NET (SAB2102224; Sigma-Aldrich) at a 1:1,000 dilution,Citation44 rabbit polyclonal antibody against the NMDAR subunit NR1 (SAB4300405; Sigma-Aldrich) at a dilution of 1:1,000, rabbit polyclonal antibody against the NMDAR subunit NR2A (M-264; Sigma-Aldrich) at 1:1,000,Citation45 rabbit polyclonal antibody against the NMDAR subunit NR2B (AP08705PU-N; Acris Antibodies GmbH, Herford, Germany) at 1:1,000, mouse monoclonal antibody against synaptophysin (Syp) (S 5768; Sigma-Aldrich) at 1:1,000,Citation46 and rabbit polyclonal antibody against the synaptosomal-associated protein 25 kDa (Snap25) (ab41455; Abcam, Cambridge, UK) 1:2,000.Citation47 Membranes were washed for 30 minutes with three changes of 0.2% TBST and were incubated in blocking solution supplemented with the following horseradish peroxidase-conjugated secondary antibodies: swine anti-rabbit 1:1,000 (Dako, Glostrup, Denmark) and goat anti-mouse 1:10,000 (DIANOVA GmbH, Hamburg, Germany) for 1 hour, with three 10-minute subsequent wash steps using 0.2% TBST. Blots were exposed to Pierce ECL Western blot detection reagent (Thermo Fisher Scientific). Photosensitive films (GE Healthcare Europe GmbH, Freiburg, Germany) were exposed to horseradish peroxidase-driven light emission. All steps were performed at room temperature.
Quantification of Western blots
Films and coomassie stained SDS gels were digitized by scanning without modifying picture properties eg, gain, color, or contrast (HP MP260; Canon, Tokyo, Japan). Densitometry was performed using ImageJ software (available from the US National Institutes of Health) to measure mean grey values of immunoblot signals and coomassie-stained poly-acrylamide gels loaded with 5 μg of protein. Background areas flanking the respective signals were measured, and the mean background values were subtracted from mean grey values obtained by measuring signals. The background adjusted values of proteins of interest were normalized to the signal intensity of the respective total protein coomassie stainings.
Statistical analysis
Statistical significance of qRT-PCR values, as well as those from the densitometric measurements of Western blots, were analyzed using a two-tailed Wilcoxon–Mann–Whitney test (SPSS software version 17.0; IBM Corporation, Armonk, NY, USA). Statistical significance was accepted at P<0.05, and P<0.1 was considered indicative for possible trends.
Results
Effects of atomoxetine on body weight
The body weight of the animals was monitored daily during the treatment period to exclude any anorexic effect of atomoxetine.Citation48 The adolescent atomoxetine-treated rats displayed no altered weight progression compared to their saline treated littermates ().
Effects of atomoxetine on NMDA receptor and NET
During the 21-day treatment period (PND 21–42), which is equivalent to childhood and adolescence in humans, atomoxetine altered both mRNA and protein doses of NET and NMDAR subunits 1, 2A, and 2B compared to controls. Detected effects were not evenly distributed throughout the brain, but rather, were identified in distinct brain regions (STR, MES, and HC). Major differences in the brains of rats analyzed immediately after the final atomoxetine application (early treatment group) and those which were housed for 2 more months off-drug (late treatment group) were identified. The PFCs in both groups were not affected by the compound. The results of both mRNA and protein analyses are summarized in .
Figure 1 Summary of the mRNA and protein analysis.
Abbreviations:
Grin, glutamate receptor ionotropic N-methyl-D-aspartate; HC, hippocampus; MES, mesencephalon; NET, norepinephrine transporter; NR, N-methyl-D-aspartate receptor subunit; PFC, prefrontal cortex; PND, postnatal day; Slc6a2, solute carrier family 6 member 2; Snap25, synaptosomal-associated protein 25 kDa; STR, striatum; Syp, synaptophysin; mRNA, messenger RNA.
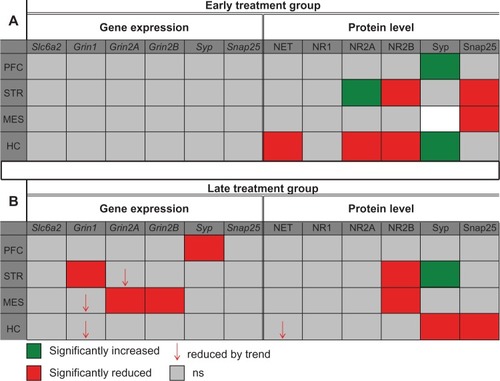
Slc6a2/NET
Since atomoxetine has a very high affinity to NET (dissociation constant [Kd] =0.29 nM),Citation49 we addressed the question as to whether Slc6a2 (NET gene) expression and/or its protein amounts are affected after in vivo atomoxetine exposure over a period of 21 days. Measurements of transcript amounts of Slc6a2 revealed no significant alterations in both early and late treatment groups compared to controls (). In contrast, immunoblotting analysis of samples derived from the hippocampus of both treatment groups displayed reduced NET levels compared to saline controls. More specifically, in the HC of the early treatment group norepinephrine transporter signals were markedly reduced by 32%±3.5% (P=0.0005). In hippocampal samples of rats assigned to the late treatment group, NET levels were also found to be reduced by trend (, ).
Figure 2 Atomoxetine induced changes on Slc6a2 messenger (m)RNA and NET levels.
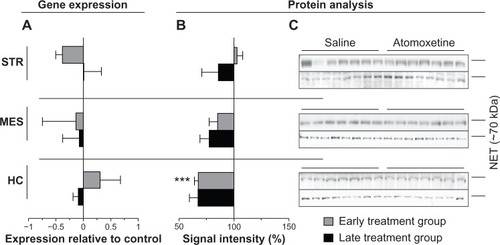
Effects of atomoxetine on the NMDAR subunits
Previously, it has been hypothesized that the onset of ADHD might be linked to a hyperglutamatergic state.Citation11 Several magnetic resonance spectroscopy (MRS) studies revealed dysregulations in the glutamatergic system and effects of ADHD drugs on glutamate metabolism.Citation50–Citation53 In line with these findings, our group recently demonstrated (in vitro) atomoxetine’s antagonistic property at the NMDAR.Citation36 Therefore, we addressed the issue of whether atomoxetine affects the expression and/or the protein levels of the three main NMDAR subunits, NR1 (Grin1), NR2A (Grin2A), and NR2B (Grin2B) in vivo. Atomoxetine exposure for 21 days reduced the expression and protein amount of the NMDAR subunits in the rat brain compared to controls.
Grin1/NR1
In the STR of the late treatment group, atomoxetine significantly reduced both mRNA and protein levels of NR1 by 13%±4.2% (P=0.0498) and 36%±6.7% (P=0.0069), respectively (). We also identified reduction of Grin1 mRNA species in MES and HC of the late treatment group. However, statistical analysis of atomoxetine’s impact on Grin1 expression in MES and HC revealed only a reduction by trend (). The assayed samples collected from rats assigned to the early treatment group did not elicit significant changes after atomoxetine exposure ().
Figure 3 Atomoxetine induced changes on Grin1 messenger (m)RNA and NR1 levels.
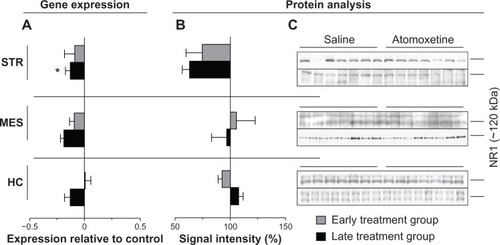
Grin2A/NR2A
The qRT-PCR measurements of total RNA isolated from brain regions of the late treatment group, elicited 34%±3.2% lower Grin2A transcript in MES (P=0.0069) and 16%±4.6% reduced levels of striatal Grin2A (P=0.0829) (). However, the treatment displayed no effect on NR2A protein levels (). Furthermore, atomoxetine exposure affected neither Grin2A expression nor NR2A protein levels in the tested brain regions of the early treatment group ().
Figure 4 Atomoxetine induced changes on Grin2A messenger (m)RNA and NR2A levels.
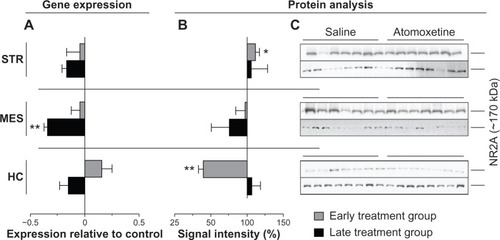
Grin2B/NR2B
Atomoxetine displayed profound impact on Grin2B expression in the late treatment group. The compound markedly reduced Grin2B mRNA levels by 50%±3.7% in the MES (P=0.0206) (), whereas mesencephalic NR2B immunoblot signals remained unaltered (). Additionally, we identified significantly reduced NR2B protein levels in the STR (P=0.0011) in both early (33%±8.2% reduction) and late treatment (66%±3.7% reduction) groups (). Furthermore, hippocampal NR2B amounts in rats assigned to the early treatment group were also found to be lower (38%±8.1% reduction) compared to saline controls (). In contrast, atomoxetine did not affect expression of the probed genes in the analyzed brain regions of animals assigned to the early treatment group.
Figure 5 Atomoxetine induced changes on Grin2B messenger (m)RNA and NR2B levels.
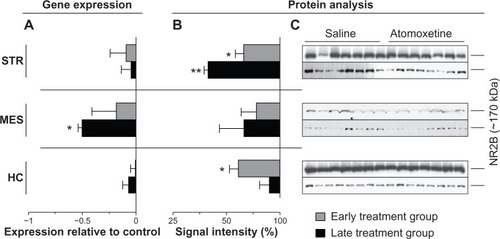
Provoked alterations in synaptic composition – preliminary findings
To test the hypothesis concluded from the above mentioned results, if the detected changes in the glutamatergic system – mainly decreased levels of NMDAR – would lead to further alterations in the synaptic composition, we investigated the levels of two SNARE (soluble N-ethylmaleimide-sensitive factor attachment protein receptor) proteins, Syp and Snap 25. Both were significantly altered in both treatment groups (, , and ).
Figure 6 Atomoxetine induced changes on Syp messenger (m)RNA and protein levels.
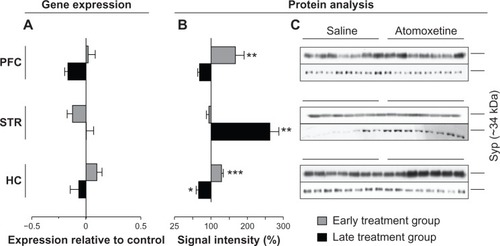
Figure 7 Atomoxetine induced changes on Snap25 messenger (m)RNA and protein levels.
Abbreviation: MES, mesencephalon.
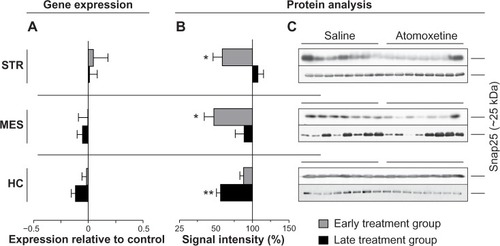
Discussion
In the present study, we assessed the question of immediate and long-term impact of a chronic 3-week application of atomoxetine on the NE and glutamatergic system. The assessment was conducted immediately after the 21 days of exposure (early treatment group) and 2 months later (late treatment group).
The administered atomoxetine ip dosage of 3 mg/kg per day is approximately twice the maximum of the recommended daily dosage in humans. However, considering the much faster metabolism in rats, the applied dosage is well within the therapeutic range. Sprague Dawley rats exposed to this particular atomoxetine dosage for 3 weeks displayed profound changes in both NET and NMDAR subunit transcriptional and translational processes. The effects on mRNA and protein levels of NET and the NMDAR subunits differed across the analyzed treatment groups and assayed brain regions. Taking the age of the animals into account (PND 21–42), which is the treatment period equivalent to childhood and early adolescence in humans, atomoxetine displays strong impact on brain biochemistry, thus underpinning the compound’s impact on cerebral development already after only 21 days of treatment.
Assaying protein levels of the reported primary biochemical target of atomoxetine, NET levels in both early and late treatment group HC samples were reduced compared to controls. However, the impact of the substance had no effect on the transporter’s gene expression. As Bymaster et alCitation27 showed in their combined in vivo and in vitro study, atomoxetine inhibits radioligand binding in cells transfected with human NETs with binding affinity (Ki) values of 5 nM. For illustration purposes, note that recommended serum levels in humans, treated with atomoxetine, are in the range of 200–1,000 ng/mL, equivalent to 0.6–3.4 μM.Citation33 Presumably, brain concentrations are even higher.Citation34 Therefore, atomoxetine probably displays strong NE reuptake inhibitory effects, which in consequence, might have triggered the observed reduction of NET levels. Furthermore, altered NET levels might also be indirectly linked to atomoxetine’s NMDAR antagonistic effect. As reviewed by Duguid and Smart,Citation54 presynaptic NMDARs affect synaptic transmissionCitation55 by modifying neurotransmitter release via Ca2+ entry and influencing transmitter release of presynaptic vesicles and their fusion with the presynaptic membrane.Citation56,Citation57 Pittaluga and RaiteriCitation58 have demonstrated increased NE release in HC modulated by presynaptic NMDARs. Atomoxetine may inhibit these presynaptic NMDARs,Citation23 resulting in altered transmitter release, which modulates the presynapse by affecting proteins localized to the presynapse. To strengthen our hypothesis of atomoxetine’s effect at the presynapse, we additionally assayed the presynaptic vesicle marker Syp and the Snap25, known to be crucial for vesicle fusion and transmitter release into the synaptic cleft. Analysis of the two proteins revealed significant alterations in the STR and HC of both early and late treatment groups (). Furthermore, we observed significantly reduced protein levels of hippocampal NR2B in the early treatment group and lower Grin1 mRNA amounts in the HC of the late treatment group. Due to the nature of the techniques used, we cannot allocate the effects on the NMDAR subunits to the presynapse or postsynapse. However, our findings of altered NMDAR subunit mRNA and protein levels correlate with the changes of NET levels and altered proteins crucial for transmitter release in the HC of both treatment groups. Therefore, we hypothesize that in our in vivo study, atomoxetine treatment had a profound impact on the presynapse.
Previously, the modulatory effect of two other NMDAR antagonists, acamprosate and MK-801 (dizocilpine), on transcript and protein levels of NMDAR subunits has been demonstrated in the rat brain.Citation59 Rammes et al demonstrated significantly increased mRNA and protein levels in the HC and cortex after a single ip dose in adult male Wistar rats.Citation59 At first glance their results seem to be contrary to our findings; however, in the present study, younger rats from a different strain were probed. The two NMDAR inhibitors used by Rammes et al to block the receptor channel have diverse chemical and biochemical properties compared to atomoxetine. The chronic exposure to atomoxetine (21 days) obviously triggers diverse effects on gene expression and protein amounts of the ionotropic glutamate receptors. The discrepancies between the findings of the two studies might be due to the different age of the groups; NMDA receptors undergo prominent changes during brain development,Citation60,Citation61 such as altered subunit composition, which changes their functionalityCitation62 and modifies their pharmacological response.Citation14,Citation63,Citation64
Notably, alterations of gene expression were only found in the late treatment group. The impact on NMDAR subunit protein levels was also evident, as they increased in the late treatment group, indicating that atomoxetine influences brain development far beyond the direct pharmacological effect. Atomoxetine seems to stimulate a change in synaptic composition that even amplifies in the course of development.
Another issue to address is the apparent discrepancy between effects on mRNA and protein levels. As we compared the effects of atomoxetine on mRNA and protein level, we identified statistically significant alterations of both entities, but did not find congruent results. In general, mRNA and protein quantities approximately correlated. However, in a correlation study by Guo et al,Citation65 the expression profile of 71 genes and their respective proteins in circulating monocytes collected from 30 unrelated women were investigated. Considering the whole dataset, the authors found a striking correlation between mRNA and protein amounts, but only five genes displayed close correlation to their corresponding proteins at the study group level. Furthermore, they observed that only 9% of the protein variation was directly linked to changes in the amount of the respective mRNA species. Guo et al concluded that gene expression and protein level correlation differ between individuals.Citation65 Considering that in the present study, an outbred rat strain was analyzed harboring large genetic heterogeneity, our contradictory findings of effects on transcript and protein level are comprehensible. Taken together, atomoxetine seems to reduce gene expression and protein levels of NET and NMDARs, leading to further regulation of synaptic composition.
Clinical implications
The involvement of the glutamatergic system in the pathophysiology of ADHD is recently coming more distinctly into view.Citation10,Citation11 In a first MRS study, MacMaster et alCitation66 detected an increase in glutamatergic tone in untreated children with ADHD compared to controls. In another study, the group investigated 14 children with ADHD, medication-free, and after treatment.Citation52 Eleven out of 14 children were treated with psychostimulants, only three with atomoxetine. The striatal glutamate/glutamine/gamma-aminobutyric acid to creatine/phosphocreatine ratio was the only detectable change in response to medication. The ratio decreased significantly. The authors postulated that an influence on glutamate metabolism might be involved in treatment response in ADHD. Also, Wiguna et al found a decreased glutamate to creatine ratio after treatment by MRS (21 children with ADHD).Citation51 In this study, it was not the STR where the findings were significant but in the PFC.
The ubiquitous neurotransmitter glutamate – nearly 60% of all neuronal synapses in the brain are glutamatergicCitation12 – is essential as a neuronal growth factor and is pivotal during neuronal development.Citation67 Recently, two open-label studies with the NMDA antagonist memantine (20 mg/day) in pediatric and adult ADHD patients showed positive effects on clinical symptoms.Citation68 Earlier studies pointed out the pivotal role of the NR2B subunit in neuron excitability and synaptic plasticity.Citation69,Citation70 Jensen et alCitation22 could show that hippocampal LTP in the SHR (PND 28), the best validated animal model of ADHD, was significantly reduced by an NR2B specific blocker, whereas the blocker had no effect in the age-matched control animals. The authors interpreted their results as a functional predominance of NR2B in SHR, meaning a delayed development. At PND 28, NR2A-containing NMDARs usually contribute substantially to LTP induction.Citation14,Citation15
In the present study, atomoxetine significantly reduced the protein level of NR2B in the HC of the adolescent rats. This action might contribute to the clinical effects of the compound.
Genome-wide association studies on ADHD patients pointed out that the modulation of neuronal and synaptic plasticity seems to be at least one of the essential mechanisms in ADHD pathophysiology,Citation71–Citation73 even though, as reviewed by Franke et al,Citation74 the results of the existing genome-wide association studies showed limited overlap.
Besides dysfunctions in neuronal glutamate transmission, increasing evidence emphasizes that abnormal neuronal development, such as disturbed synaptogenesis and/or dysfunctional neuronal migration, is important in ADHD pathophysiology.Citation75
In the present in vivo study, the majority of the reported effects of atomoxetine were identified in the STR and HC. Already in 1996, LouCitation25 pointed out the unique anatomical characteristics of the STR, a watershed region with convergent glutamatergic afferent projections from almost the entire cortex. In ischemia-induced liberation of glutamate, this accentuated position of the STR is the cause of its particular vulnerability to lesions. Besides genetic factors, pre- and perinatal events are hypothesized to be a predominant cause for ADHD.Citation25 The observed cellular mechanisms of atomoxetine in this in vivo study might at least contribute to the clinical effects ameliorating ADHD core symptoms.
Limitations
This study revealed new insights of atomoxetine’s biological properties at the cellular level. Nevertheless, some limitations of the study must be considered. The Sprague Dawley rats from Charles River are an outbred strain; the heterogeneity of the genetic background probably masked minor effects on gene expression and protein levels. In some cases of probed mRNA species the interindividual variation was very high. A further limitation is the total number of animals (n=7–8) per experiment, which was strongly regulated by regional administrative authorities.
Conclusion
In conclusion, the results of the present exploratory study demonstrate that atomoxetine’s impact on the brain is not solely limited to the inhibition of NE reuptake. The compound alters mRNA and protein levels of the respective genes and proteins crucial for synaptic plasticity, hence influencing the neuronal homoeostasis,Citation76 which presumably is disturbed in ADHD. Two actions especially might be of major interest: first, the reduction of the NMDA receptor subunit NR2B in the adolescent HC since this protein seems to be elevated in the HC of the best validated animal model of ADHD, the SHR,Citation22 and second, the influence on Snap25, a novel candidate gene which has been altered in the coloboma mouse, another animal model for ADHD.Citation77,Citation78
Furthermore, we showed that atomoxetine’s immediate and long-term effects on synaptic proteins differ, thus emphasizing its modulatory influence on synaptic plasticity. However, whether atomoxetine’s impact can directly be linked to its NMDAR and NET antagonism or is due to a still unknown pathway has yet to be elucidated. In the present study, we were able to show immediate and long-term brain region-specific effects. Disease-specific effects must be investigated in animal models of ADHD, ie, in the above mentioned SHR.Citation79,Citation80
Supplementary material
Figure S1 Weight gain of male adolescent rats during treatment period.
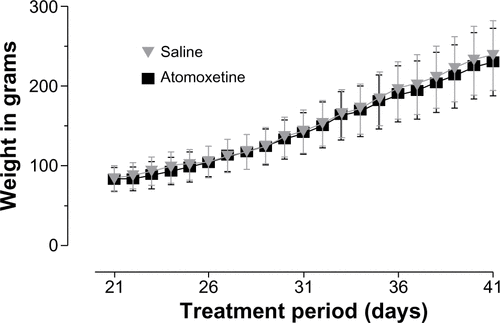
Disclosure
Professor, Dr Andrea G Ludolph has received research funding from UCB/Celltech, Medice, and Novartis. She was/is involved in clinical trials with Boehringer Ingelheim, Shire, and Otsuka. She discloses consulting fees and travel grants from Shire and lecture fees from Janssen and Lilly. Patrick T Udvardi, Dr Stefan Liebau, Carolin Henes, Dr Karl Föhr, Dr Jens Dreyhaupt, and Professor Tobias M Boeckers report no conflicts of interest in this work.
References
- Polanczyk G de Lima MS Horta BL Biederman J Rohde LA The worldwide prevalence of ADHD: a systematic review and metaregression analysis Am J Psychiatry 2007 164 6 942 948 17541055
- Biederman J Faraone SV Attention-deficit hyperactivity disorder Lancet 2005 366 9481 237 248 16023516
- Spencer TJ Biederman J Mick E Attention-deficit/hyperactivity disorder: diagnosis, lifespan, comorbidities, and neurobiology J Pediatr Psychol 2007 32 6 631 642 17556405
- Kessler RC Adler L Barkley R The prevalence and correlates of adult ADHD in the United States: results from the National Comorbidity Survey Replication Am J Psychiatry 2006 163 4 716 723 16585449
- Stahl S Attention deficit hyperactivity disorder and its treatmented Stahl S Stahl’s Essential Psychopharmacocogy 3rd ed New York, NY Cambridge University Press 2008 863 897
- Gibson AP Bettinger TL Patel NC Crismon ML Atomoxetine versus stimulants for treatment of attention deficit/hyperactivity disorder Ann Pharmacother 2006 40 6 1134 1142 16735655
- Molina BS Hinshaw SP Swanson JM MTA Cooperative Group The MTA at 8 years: prospective follow-up of children treated for combined-type ADHD in a multisite study J Am Acad Child Adolesc Psychiatry 2009 48 5 484 500 19318991
- Ferris RM Tang FL Maxwell RA A comparison of the capacities of isomers of amphetamine, deoxypipradrol and methylphenidate to inhibit the uptake of tritiated catecholamines into rat cerebral cortex slices, synaptosomal preparations of rat cerebral cortex, hypothalamus and striatum and into adrenergic nerves of rabbit aorta J Pharmacol Exp Ther 1972 181 3 407 416 5033010
- Schweri MM Skolnick P Rafferty MF Rice KC Janowsky AJ Paul SM [3H]Threo-(+/−)-methylphenidate binding to 3,4-dihydroxyphenylethylamine uptake sites in corpus striatum: correlation with the stimulant properties of ritalinic acid esters J Neurochem 1985 45 4 1062 1070 4031878
- Carrey NJ MacMaster FP Gaudet L Schmidt MH Striatal creatine and glutamate/glutamine in attention-deficit/hyperactivity disorder J Child Adolesc Psychopharmacol 2007 17 1 11 17 17343550
- Lule D Ludolph AC Ludolph AG Neurodevelopmental and neurodegenerative diseases – is there a pathophysiological link? Attention-deficit/hyperactivity disorder and amyotrophic lateral sclerosis as examples Med Hypotheses 2008 70 6 1133 1138 18158219
- Orrego F Villanueva S The chemical nature of the main central excitatory transmitter: a critical appraisal based upon release studies and synaptic vesicle localization Neuroscience 1993 56 3 539 555 7902967
- Davies J Evans RH Francis AA Watkins JC Excitatory amino acid receptors and synaptic excitation in the mammalian central nervous system J Physiol (Paris) 1979 75 6 641 654 232719
- Cull-Candy S Brickley S Farrant M NMDA receptor subunits: diversity, development and disease Curr Opin Neurobiol 2001 11 3 327 335 11399431
- Köhr G Jensen V Koester HJ Intracellular domains of NMDA receptor subtypes are determinants for long-term potentiation induction J Neurosci 2003 23 34 10791 10799 14645471
- Monyer H Burnashev N Laurie DJ Sakmann B Seeburg PH Developmental and regional expression in the rat brain and functional properties of four NMDA receptors Neuron 1994 12 3 529 540 7512349
- Stocca G Vicini S Increased contribution of NR2A subunit to synaptic NMDA receptors in developing rat cortical neurons J Physiol 1998 507 Pt 1 13 24 9490809
- Tovar KR Westbrook GL The incorporation of NMDA receptors with a distinct subunit composition at nascent hippocampal synapses in vitro J Neurosci 1999 19 10 4180 4188 10234045
- Li B Chen N Luo T Otsu Y Murphy TH Raymond LA Differential regulation of synaptic and extra-synaptic NMDA receptors Nat Neurosci 2002 5 9 833 834 12195433
- Köhr G NMDA receptor function: subunit composition versus spatial distribution Cell Tissue Res 2006 326 2 439 446 16862427
- Hardingham GE Fukunaga Y Bading H Extrasynaptic NMDARs oppose synaptic NMDARs by triggering CREB shut-off and cell death pathways Nat Neurosci 2002 5 5 405 414 11953750
- Jensen V Rinholm JE Johansen TJ N-methyl-D-aspartate receptor subunit dysfunction at hippocampal glutamatergic synapses in an animal model of attention-deficit/hyperactivity disorder Neuroscience 2009 158 1 353 364 18571865
- Malinow R Malenka RC AMPA receptor trafficking and synaptic plasticity Annu Review Neurosci 2002 25 103 126
- Bredt DS Nicoll RA AMPA receptor trafficking at excitatory synapses Neuron 2003 40 2 361 379 14556714
- Lou HC Etiology and pathogenesis of attention-deficit hyperactivity disorder (ADHD): significance of prematurity and perinatal hypoxic-haemodynamic encephalopathy Acta Paediatr 1996 85 11 1266 1271 8955450
- Gehlert DR Schober DA Gackenheimer SL Comparison of (R)-[3H] tomoxetine and (R/S)-[3H]nisoxetine binding in rat brain J Neurochem 1995 64 6 2792 2800 7760060
- Bymaster FP Katner JS Nelson DL Atomoxetine increases extracellular levels of norepinephrine and dopamine in prefrontal cortex of rat: a potential mechanism for efficacy in attention deficit/hyperactivity disorder Neuropsychopharmacology 2002 27 5 699 711 12431845
- Easton N Steward C Marshall F Fone K Marsden C Effects of amphetamine isomers, methylphenidate and atomoxetine on synaptosomal and synaptic vesicle accumulation and release of dopamine and noradrenaline in vitro in the rat brain Neuropharmacology 2007 52 2 405 414 17020775
- Peterson K McDonagh MS Fu R Comparative benefits and harms of competing medications for adults with attention-deficit hyperactivity disorder: a systematic review and indirect comparison meta-analysis Psychopharmacology (Berl) 2008 197 1 1 11 18026719
- Seneca N Gulyás B Varrone A Atomoxetine occupies the norepinephrine transporter in a dose-dependent fashion: a PET study in nonhuman primate brain using (S,S)-[18F]FMeNER-D2 Psychopharmacology (Berl) 2006 188 1 119 127 16896954
- Takano A Gulyás B Varrone A Maguire RP Halldin C Saturated norepinephrine transporter occupancy by atomoxetine relevant to clinical doses: a rhesus monkey study with (S,S)-[(18)F]FMeNER-D (2) Eur J Nucl Med Mol Imaging 2009 36 8 1308 1314 19300997
- Kratochvil CJ Heiligenstein JH Dittmann R Atomoxetine and methylphenidate treatment in children with ADHD: a prospective, randomized, open-label trial J Am Acad Child Adolesc Psychiatry 2002 41 7 776 784 12108801
- Hiemke C Baumann P Bergemann N AGNP consensus guidelines for therapeutic drug monitoring in psychiatry: update 2011 Pharmacopsychiatry 2011 44 6 195 235
- Kielbasa W Kalvass JC Stratford R Microdialysis evaluation of atomoxetine brain penetration and central nervous system pharmacokinetics in rats Drug Metab Dispos 2009 37 1 137 142 18936112
- Scherer D Hassel D Bloehs R Selective noradrenaline reuptake inhibitor atomoxetine directly blocks hERG currents Br J Pharmacol 2009 156 2 226 236 19154426
- Ludolph AG Udvardi PT Schaz U Atomoxetine acts as an NMDA receptor blocker in clinically relevant concentrations Br J Pharmacol 2010 160 2 283 291 20423340
- Lempp T Toennes SW Wunder C Altered gene expression in the prefrontal cortex of young rats induced by the ADHD drug atomoxetine Prog Neuropsychopharmacol Biol Psychiatry 2013 40 221 228 22960082
- Ikonomidou C Bittigau P Koch C Neurotransmitters and apoptosis in the developing brain Biochem Pharmacol 2001 62 4 401 405 11448448
- Paxinos G Watson C The Rat Brain: In Stereotactic Coordinates 2nd Ed London Academic Press 1986
- Vandesompele J De Preter K Pattyn F Accurate normalization of real-time quantitative RT-PCR data by geometric averaging of multiple internal control genes Genome biology. RESEARCH0034 2002 3 7
- Mestdagh P Van Vlierberghe P De Weer A A novel and universal method for microRNA RT-qPCR data normalization Genome biology 2009 10 6 R64 19531210
- Schaffner W Weissmann C A rapid, sensitive, and specific method for the determination of protein in dilute solution Anal Biochem 1973 56 2 502 514 4128882
- Aldridge GM Podrebarac DM Greenough WT Weiler IJ The use of total protein stains as loading controls: an alternative to high-abundance single-protein controls in semi-quantitative immunoblotting J Neurosci Methods 2008 172 2 250 254 18571732
- Bayles R Harikrishnan KN Lambert E Epigenetic modification of the norepinephrine transporter gene in postural tachycardia syndrome Arterioscler Thromb Vasc Biol 2012 32 8 1910 1916 22723437
- Altafaj X Ortiz-Abalia J Fernández M Increased NR2A expression and prolonged decay of NMDA-induced calcium transient in cerebellum of TgDyrk1A mice, a mouse model of Down syndrome Neurobiol Dis 2008 32 3 377 384 18773961
- Uriu Y Kiyonaka S Miki T Rab3-interacting molecule gamma isoforms lacking the Rab3-binding domain induce long lasting currents but block neurotransmitter vesicle anchoring in voltage-dependent P/Q-type Ca2+ channels J Biol Chem 2010 285 28 21750 21767 20452978
- Chalazonitis A Tang AA Shang Y Homeodomain interacting protein kinase 2 regulates postnatal development of enteric dopaminergic neurons and glia via BMP signaling J Neurosci 2011 31 39 13746 13757 21957238
- Michelson D Faries D Wernicke J Atomoxetine ADHD Study Group Atomoxetine in the treatment of children and adolescents with attention-deficit/hyperactivity disorder: a randomized, placebo-controlled, dose-response study Pediatrics 2001 108 5 E83 11694667
- Gehlert DR Gackenheimer SL Robertson DW Localization of rat brain binding sites for [3H]tomoxetine, an enantiomerically pure ligand for norepinephrine reuptake sites Neurosci Lett 1993 157 2 203 206 8233054
- Arcos-Burgos M Londoño AC Pineda DA Analysis of brain metabolism by proton magnetic resonance spectroscopy (1H-MRS) in attention-deficit/hyperactivity disorder suggests a generalized differential ontogenic pattern from controls Atten Defic Hyperact Disord 2012 4 4 205 212 23012086
- Wiguna T Guerrero APS Wibisono S Sastroasmoro S Effect of 12-week administration of 20-mg long-acting methylphenidate on Glu/Cr, NAA/Cr, Cho/Cr, and mI/Cr ratios in the prefrontal cortices of school-age children in Indonesia: a study using 1H magnetic resonance spectroscopy (MRS) Clin Neuropharmacol 2012 35 2 81 85 22318191
- Carrey N MacMaster FP Fogel J Metabolite changes resulting from treatment in children with ADHD: a 1H-MRS study Clin Neuropharmacol 2003 26 4 218 221 12897644
- Tafazoli S O’Neill J Bejjani A 1H MRSI of middle frontal gyrus in pediatric ADHD J Psychiatr Res 2013 47 4 505 512 23273650
- Duguid IC Smart TG Presynaptic NMDAR receptors VanDongen AM Biology of the NMDAR Receptor Boca Raton, FL CRC Press 2009 313 328
- Langer SZ Presynaptic autoreceptors regulating transmitter release Neurochem Int 2008 52 1–2 26 30 17583385
- Stevens CF Presynaptic function Curr Opin Neurobiol 2004 14 3 341 345 15194114
- Sudhof TC The synaptic vesicle cycle Annu Rev Neurosci 2004 27 509 547 15217342
- Pittaluga A Raiteri M N-methyl-D-aspartic acid (NMDA) and non-NMDA receptors regulating hippocampal norepinephrine release. III. Changes in the NMDA receptor complex induced by their functional cooperation J Pharmacol Exp Ther 1992 263 1 327 333 1357159
- Rammes G Mahal B Putzke J The anti-craving compound acamprosate acts as a weak NMDA-receptor antagonist, but modulates NMDA-receptor subunit expression similar to memantine and MK-801 Neuropharmacology 2001 40 6 749 760 11369029
- Sheng M Cummings J Roldan LA Jan YN Jan LY Changing subunit composition of heteromeric NMDA receptors during development of rat cortex Nature 1994 368 6467 144 147 8139656
- Wenzel A Fritschy JM Mohler H Benke D NMDA receptor heterogeneity during postnatal development of the rat brain: differential expression of the NR2A, NR2B, and NR2C subunit proteins J Neurochem 1997 68 2 469 478 9003031
- Barria A Malinow R NMDA receptor subunit composition controls synaptic plasticity by regulating binding to CaMKII Neuron 2005 48 2 289 301 16242409
- Martin D Bustos GA Bowe MA Bray SD Nadler JV Autoreceptor regulation of glutamate and aspartate release from slices of the hippocampal CA1 area J Neurochem 1991 56 5 1647 1655 1672884
- Paoletti P Neyton J NMDA receptor subunits: function and pharmacology Curr Opin Pharmacol 2007 7 1 39 47 17088105
- Guo Y Xiao P Lei S How is mRNA expression predictive for protein expression? A correlation study on human circulating monocytes Acta Biochim Biophys Sin (Shanghai) 2008 40 5 426 436 18465028
- MacMaster FP Carrey N Sparkes S Kusumakar V Proton spectroscopy in medication-free pediatric attention-deficit/hyperactivity disorder Biol Psychiatry 2003 53 2 184 187 12547476
- Ikonomidou C Bosch F Miksa M Blockade of NMDA receptors and apoptotic neurodegeneration in the developing brain Science 1999 283 5398 70 74 9872743
- Findling RL Short EJ McNamara NK Methylphenidate in the treatment of children and adolescents with bipolar disorder and attention-deficit/hyperactivity disorder J Am Acad Child Adolesc Psychiatry 2007 46 11 1445 1453 18049294
- Standaert DG Testa CM Young AB Penney JB Organization of N-methyl-D-aspartate glutamate receptor gene expression in the basal ganglia of the rat J Comp Neurol 1994 343 1 1 16 8027428
- Puliti A Rossi PI Caridi G Albuminuria and glomerular damage in mice lacking the metabotropic glutamate receptor 1 Am J Pathol 2011 178 3 1257 1269 21356376
- Neale BM Lasky-Su J Anney R Genome-wide association scan of attention deficit hyperactivity disorder Am J Med Genet B Neuropsychiatr Genet 2008 147B 8 1337 1344 18980221
- Lesch KP Timmesfeld N Renner TJ Molecular genetics of adult ADHD: converging evidence from genome-wide association and extended pedigree linkage studies J Neural Transm 2008 115 11 1573 1585 18839057
- Romanos M Freitag C Jacob C Genome-wide linkage analysis of ADHD using high-density SNP arrays: novel loci at 5q13.1 and 14q12 Mol Psychiatry 2008 13 5 522 530 18301393
- Franke B Neale BM Faraone SV Genome-wide association studies in ADHD Hum Genet 2009 126 1 13 50 19384554
- Poelmans G Pauls DL Buitelaar JK Franke B Integrated genome-wide association study findings: identification of a neurodevelopmental network for attention deficit hyperactivity disorder Am J Psychiatry 2011 168 4 365 377 21324949
- Ramocki MB Zoghbi HY Failure of neuronal homeostasis results in common neuropsychiatric phenotypes Nature 2008 455 7215 912 918 18923513
- Hess EJ Jinnah HA Kozak CA Wilson MC Spontaneous locomotor hyperactivity in a mouse mutant with a deletion including the Snap gene on chromosome 2 J Neurosci 1992 12 7 2865 2874 1613559
- Hess EJ Collins KA Wilson MC Mouse model of hyperkinesis implicates SNAP-25 in behavioral regulation J Neurosci 1996 16 9 3104 3111 8622140
- Sagvolden T Behavioral validation of the spontaneously hypertensive rat (SHR) as an animal model of attention-deficit/hyperactivity disorder (AD/HD) Neurosci Biobehav Rev 2000 24 1 31 39 10654658
- Sagvolden T Johansen EB Aase H Russell VA A dynamic developmental theory of attention-deficit/hyperactivity disorder (ADHD) predominantly hyperactive/impulsive and combined subtypes Behav Brain Sci 2005 28 3 397 419 discussion 419–468 16209748