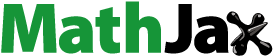
Abstract
Chitosan (CS) iron oxide magnetic nanoparticles (MNPs) were coated with phytic acid (PTA) to form phytic acid-chitosan-iron oxide nanocomposite (PTA-CS-MNP). The obtained nanocomposite and nanocarrier were characterized by powder X-ray diffraction, Fourier transform infrared spectroscopy, vibrating sample magnetometry, transmission electron microscopy, and thermogravimetric and differential thermogravimetric analyses. Fourier transform infrared spectra and thermal analysis of MNPs and PTA-CS-MNP nanocomposite confirmed the binding of CS on the surface of MNPs and the loading of PTA in the PTA-CS-MNP nanocomposite. The coating process enhanced the thermal stability of the anticancer nanocomposite obtained. X-ray diffraction results showed that the MNPs and PTA-CS-MNP nanocomposite are pure magnetite. Drug loading was estimated using ultraviolet-visible spectroscopy and showing a 12.9% in the designed nanocomposite. Magnetization curves demonstrated that the synthesized MNPs and nanocomposite were superparamagnetic with saturation magnetizations of 53.25 emu/g and 42.15 emu/g, respectively. The release study showed that around 86% and 93% of PTA from PTA-CS-MNP nanocomposite could be released within 127 and 56 hours by a phosphate buffer solution at pH 7.4 and 4.8, respectively, in a sustained manner and governed by pseudo-second order kinetic model. The cytotoxicity of the compounds on HT-29 colon cancer cells was evaluated by 3-(4,5-dimethylthiazol-2-yl)-2,5-diphenyltetrazolium bromide assay. The HT-29 cell line was more sensitive against PTA-CS-MNP nanocomposite than PTA alone. No cytotoxic effect was observed on normal cells (3T3 fibroblast cells). This result indicates that PTA-CS-MNP nanocomposite can inhibit the proliferation of colon cancer cells without causing any harm to normal cell.
Introduction
Nanotechnology has incredible potential for revolutionizing the medical world by developing personalized medicine both for diagnosis and therapy. Nanomedicine (nanosized therapeutics and imaging agents) has exhibited a strong growth in nanodrugs where designed ingenious biocompatible nanocomposites for drug delivery purposes. These nanocomposites are widely used as nanodelivery systems that enhance the biodistribution of drugs and selectively target diseased tissues while protecting healthy tissues.Citation1,Citation2
Various nanocarriers including liposomes,Citation3–Citation6 cell-based nanocarriers,Citation7 polymers,Citation8 carbon materials,Citation9,Citation10 dendrimers,Citation11 and inorganic nanoparticlesCitation12,Citation13 have been employed for gene and drug delivery systems, which have shown efficacy both in vitro and in vivo. Recently, much attention has been devoted to polymer-based nanoparticles (typically chitosan [CS]-iron oxide nanoparticles) as novel cellular delivery vectors in cancer therapy because of their excellent properties such as high encapsulation efficiency, sustained release properties, effective and convenient routes of administration, less toxicity, endocytosis efficiency, surface modifying capacity, ability to differentiate between cancer and normal cells,Citation14 superparamagnetic behavior and contrast agent, and low health care cost.Citation15
Iron oxide magnetic nanoparticles (MNPs) with general formula Fe3O4 have been widely used for delivery of various drugs owing to some advantages: ease of preparation, superparamagnetic property,Citation16–Citation18 biocompatibility, external control, controllable parameters,Citation19,Citation20 and hypothermal behavior.Citation21,Citation22 However, these nanoparticles due to the presence of OH− groups with a large surface area to volume ratio and dipole–dipole attraction without any surface coating will be agglomerated and the size of particles will increase. Therefore, it is necessary to use polymers in the coating process to prevent the nuclear growth of iron oxide, preclude nanoparticles aggregation, and reduce toxic effect.Citation23–Citation25
CS, a natural polymer and deacetylated derivative of chitin, is most commonly used as a coating material for nanoparticles in anticancer drug delivery systems because of its unique biological features including biocompatibility,Citation26 anti-carcinogenicity, biodegradability, nontoxicity, hydrophilicity,Citation23,Citation27–Citation29 and great antimicrobial property.Citation30 The CS polymer reacts with iron oxide nanoparticles through glycosidic bonds and improves the stability and chemical reactivity of nanoparticles.Citation31
Extensive studies using CS-MNPs nanocarrier have been performed in recent years to enhance anticancer drug efficacy and reduce the side effects of the drug. For instance, Javid et alCitation32 demonstrated that CS-MNPs nanoreservoir with a higher drug loading capacity increased the drug efficiency and anticancer properties of doxorubicin. The results emphasized that doxorubicin-CS-MNP nanocomposite showed a greater growth inhibitory effect on ovarian cancer cells (A2780 and OVCAR-3) than free drug.Citation32
Phytic acid (PTA; C6H18O24P6) is a natural compound discovered in 1903. It is the major storage form of phosphorous in plants and legumes, including wheat bran, corn, soy beans, rice bran, and nuts,Citation33 and it is reported to possess a broad range of pharmaceutical properties, such as antioxidant,Citation34,Citation35 chemopreventive,Citation36,Citation37 hepatoprotective, and anticancer properties. Many studies presented that PTA inhibited cancer cell growth in leukemia, prostate, breast, liver, colon, and skin cancers.Citation38–Citation40 However, this anticancer drug has very short plasma half-life and is released very fast.
Therefore, considering the aforementioned advantages of CS-MNPs, we report here the synthesis of a new nanohybrid, phytic acid-chitosan-MNPs (PTA-CS-MNP). The objective of this research was to explore the potential use of CS-MNPs for the delivery of anticancer drug PTA. We investigated the sustained release behavior of PTA from CS-MNPs nanocarrier and evaluated the cytotoxic effects against cancer cells and normal fibroblast (3T3) cell lines in vitro.
Materials and methods
Materials
The chemicals in this study were of analytical grade and were used as received without further purification. PTA sodium salt from rice (molecular formula: C6H18O24P6 xNa+·yH2O; molecular weight: 660.04) with ≥90 purity and CS, deacetylated at 75%–85%, sodium amp salt (>98% purity; molecular weight: 371.4 g/mol) were acquired from Sigma-Aldrich (St Louis, MO, USA). Aqueous acetic acid solution (99.8%) was supplied by Hamburg Industries (Hamburg, Germany). Ferrous chloride tetrahydrate (FeCl2·4H2O, ≥99% purity) and ferric chloride hexahydrate (FeCl3·6H2O, ≥99% purity) were obtained from Merck KGaA (Darmstadt, Germany). Ammonia solution (25%) was purchased from Scharlau (Sentmenat, Barcelona, Spain). Deionized water was used in each experiment.
Synthesis of MNPs
The MNPs were prepared by a coprecipitation method as previously reported.Citation41 They were prepared by mixing solutions of 2.43 g of ferrous chloride tetrahydrate (FeCl2·4H2O), 0.99 g ferric chloride hexahydrate (FeCl3·6H2O), and 80 mL of deionized water in the presence of 6 mL of ammonia (25% by mass). The pH value of the solution was maintained at 10. Then the mixture was sonicated for 1 hour at room temperature. Finally, the precipitate was centrifuged and washed three times with deionized water.
Synthesis of CS-MNPs
The solution of CS was prepared by dissolving 1 g of CS powder in 1% acetic acid solution. It was followed by the addition of CS solution to MNPs suspension and then the mixture was stirred for 18 hours at room temperature. The obtained mixture was centrifuged and washed three times with deionized water and dried at 70°C. The product was labeled CS-MNPs.Citation23
Synthesis of PTA-CS-MNP nanocomposite
The PTA-CS-MNP nanocomposite was obtained by mixing PTA sodium salt solution (2 g in 100 mL deionized water) with a known amount of CS-MNPs. The solution was then kept under vigorous stirring for 24 hours. The final slurry was centrifuged, washed, and finally dried at 60°C oven.
Characterization of PTA-CS-MNP nanocomposite
The magnetic property of the samples was evaluated using Lake Shore 7404 vibrating sample magnetometer (Lake Shore Cryotronics, Inc., Westerville, OH, USA). The thermal analyses of the materials were performed using Mettler Toledo thermogravimetric (TGA) and differential thermogravimetric (DTG) instruments with a heating rate of 10°C per minute at temperature 20°C–1,000°C under nitrogen atmosphere (N2 flow rate 50 mL per minute). Powder X-ray diffraction (PXRD) patterns were used to determine the crystal structures of the samples over a range of 5°–70°, using an XRD-6000 diffractometer (Shimadzu, Tokyo, Japan) with CuKα radiation (λ =1.5406 Å) at 30 kV and 30 mA. Fourier transform infrared (FTIR) spectra of the materials were recorded over a range of 400–4,000 cm−1, using a Nexus, Smart Orbit spectrometer (Thermo Fisher Scientific, Waltham, MA, USA) and KBr disk method. The ultraviolet-visible (UV-Vis) spectrophotometer (Shimadzu 1650 series; Shimadzu) was used to determine the optical and controlled-release properties of PTA from PTA-CS-MNP nanocomposite. The mean particle size and size distribution were determined using a transmission electron microscope (Hitachi H-7100; Hitachi, Tokyo, Japan) at an accelerating voltage of 80 and 200 kV.
Loading and release of PTA from PTA-CS-MNP nanocomposite
The percentage of PTA loading in PTA-CS-MNP nanocomposite was measured using UV-Vis spectrophotometer (Shimadzu 1650 series; Shimadzu) and calibration curve equation. Approximately 5 mg of the nanocomposite was dissolved in concentrated HCl/HNO3. In this condition 100% PTA content would be released from the nanocomposite. The amount of PTA released was measured by UV-Vis spectroscopy at a wavelength of 275 nm and using calibration curves.
PTA release profiles from the nanocomposite were determined at room temperature using 0.01 M phosphate-buffered saline (PBS) solution at pH 4.8 and 7.4. A total of 85 mg of PTA-CS-MNP nanocomposite was added to 500 mL of PBS medium. The cumulative amount of PTA released into the solution was measured at predetermined time intervals at λmax =275 nm using a UV-Vis spectrophotometer. The release rate of PTA from PTA-CS-MNP nanocomposite was compared with that from physical mixture that contained PTA sodium salt, CS, and MNPs.
Cytotoxicity assay of PTA-CS-MNP nanocomposite
HT-29 and 3T3 cells were obtained from American Type Culture Collection (ATCC, VA, USA), and these cells were cultured and plated at a density of 1×104 cells by adding 100 μL of cell suspension to each well of a 96-well tissue culture plate. The plates were incubated for sufficient time to ensure attachment of cells. The growth rate was ~70%–80% confluency. The media were aspirated off and replaced with fresh media (100 μL) containing different concentrations of PTA-CS-MNP nanocomposite as well as pure compound, PTA (25–500 μg/mL). The treated plates were incubated at 37°C in 5% CO2 for 72 hours with appropriate control. After incubation with the compounds, 3-(4,5-dimethylthiazol-2-yl)-2,5-diphenyltetrazolium bromide (MTT) solution (5 mg/mL) in a total volume of 10 μL was added to every well and mixed gently with the media, which was later incubated for 4 hours at 37°C in 5% CO2. The MTT solution containing the medium was then removed carefully and replaced with dimethyl sulfoxide (DMSO) (100 μL per well) to dissolve the formazan crystals. Finally, the culture plates were read in a microplate reader at 570 nm. The concentration of drug needed to inhibit cell growth by 50% (IC50) was generated from the dose–response curves for each compound and each cell line.
Results and discussion
PXRD
illustrates the PXRD patterns of the MNPs, PTA-CS-MNP, and PTA sodium salt, respectively. For MNPs and PTA-CS-MNP nanocomposite, six characteristic peaks at 2θ=30.2°, 35.5°, 43.2°, 53.5°, 57.2°, and 62.7° were indexed to (220), (311), (400), (422), (511), and (440), respectively, (JCPDS No 19-629) which are due to the cubic spinel structure of pure Fe3O4.Citation42 reveals that the coating process did not affect the phase change of MNPs as there is no changes in the position of the peaks.Citation43 However, the coating process resulted in a lower peak intensity and crystallinity of the PTA-CS-MNP nanocomposite. The mean grain size was calculated from XRD data by Debye-Scherrer equation that determines the connection between average particle size and peak broadening in XRD.
where D is the average particle size, K is the Debye-Scherrer constant (0.89), λ is the X-ray wavelength (0.15406 nm), β is the peak width of half-maximum, and θ is the diffraction angle. The mean particle size of MNPs obtained from this equation was ~8 nm.
FTIR spectroscopy
The FTIR spectra of MNPs, PTA-CS-MNP nanocomposite, and PTA sodium salt are presented in . The MNPs in shows an absorption peak at 569 cm−1 that is due to the stretching of Fe-O in Fe3O4. However, this peak was shifted to 565 cm−1 in the PTA-CS-MNP nanocomposite (), which confirm the presence of MNPs in the nanocomposites.Citation44 The broad absorption peak observed at 3,415 cm−1 in is ascribed to the O–H stretching. In the PTA-CS-MNP nanocomposite spectra (), the characteristic band for CS was observed at 1,560 cm−1, which proves that MNPs were successfully coated with CS.Citation45
Figure 2 Fourier transform infrared spectra of iron oxide magnetic nanoparticles (A), PTA-CS-MNP nanocomposite (B), and phytic acid sodium salt (C).
Abbreviation: PTA-CS-MNP, phytic acid-chitosan-iron oxide nanocomposite.
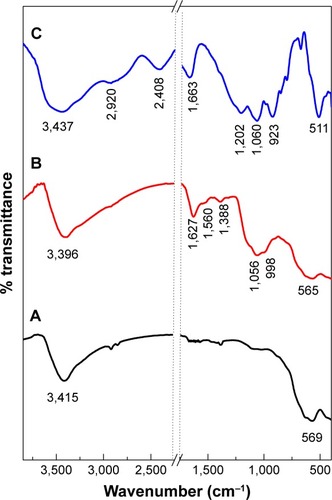
The FTIR spectra of PTA sodium salt shown in displays some intense, sharp absorption peaks due to different functional groups in the molecules. The characteristic bands at 1,202 and 1,060 cm−1 can be assigned to the stretching vibration of P=O and P–O–C, respectively, and in the nanocomposite spectrum (), the bands shifted to 1,388 and 1,056 cm−1, respectively, due to coating process. The stretch at 511 cm−1 corresponded to the O–Na and O–P=O vibrations were revealed at 2,408 and 2,920 cm−1 ().Citation46 The absorption peaks of OH group of water molecules present in the PTA sodium salt appeared at 1,663 and 3,437 cm−1,Citation46 while the peaks were recorded at 1,627 and 3,396 cm−1 in the nanocomposite spectra ().
shows the interaction between the MNPs, CS, and PTA. It is clear that MNPs interacted with CS through glycosidic bonds (), whereas PTA () interacted with CS polymer through hydrogen bonds.Citation47,Citation48
Thermal analysis
The thermal behavior of PTA sodium salt, MNPs, and PTA-CS-MNP nanocomposite was examined by TGA and DTG analyses (). The PTA sodium salt thermal decomposition () showed three weight loss events. The first event occurred at 123°C with a weight loss of 25.9%, which was due to the removal of water present in the sample. The second weight loss of 20.6% at 205°C might be due to the carbonization process and dehydration that takes place due to the decomposition of OH groups. This third one was followed by the decomposition of phytate groups and the elimination of elemental carbon formed in the previous step at 309°C with a mass reduction of 41.2%.Citation49 The TGA/DTG thermograms of MNPs () exhibited a single-stage mass reduction at 44°C with a weight loss of 9.7%, which can be attributed to the loss of surface hydroxyl groups and residual water.Citation25 For PTA-CS-MNP nanocomposite, three main thermal phenomena were observed (). The first weight loss of 9.6% at 62°C was related to the removal of surface physisorbed water molecules. The second and third weight loses of 23.4% and 12.1% at 242°C and 610°C, respectively, corresponded to the decomposition of PTA and CS biopolymer. The temperature region of the nanocomposite appeared to be clearly higher than that of the free drug due to the electrostatic attraction between the MNPs, CS, and PTA.
Magnetic properties
Superparamagnetism is an essential and important property for magnetic-targeting carriers in drug-delivery system.Citation50 Magnetic properties of MNPs and PTA-CS-MNP nanocomposite were characterized by vibrating sample magnetometer as a function of the magnetic field at room temperature (). The saturation magnetization of MNPs was 53.25 emu/g compared to that of PTA-CS-MNP nanocomposite which was 42.15 emu/g, and negligible coercivity and remanent magnetization were observed for both samples, which confirmed the superparamagnetic properties of the samples. The decrease in saturation magnetization of PTA-CS-MNPs nanocomposite could be due to the existence of coated materials on the surface of MNPs and electron exchange between CS polymer coating and Fe atoms.Citation51–Citation53 This could affect surface magnetic anisotropy and enhance surface spins disorientation.Citation54
Determination of average size and size distribution properties
Transmission electron microscopy images and size distribution of pristine Fe3O4, CS-MNPs, and PTA-CS-MNP nanocomposite are presented in . The images show that nanosized particles were successfully prepared using coprecipitation method, and all the samples such as MNPs, CS-MNPs, and PTA-CS-MNP nanocomposite showed roughly spherical shape (). The average size of MNPs was ~15±6 nm (), whereas the mean sizes of CS-MNPs and PTA-CS-MNP nanocomposite were around 12±4 nm and 8±3 nm, respectively (). The decrease in the size of CS-MNPs and PTA-CS-MNP nanocomposite after coating might be due to prolonged vigorous stirring at high speeds after adding CS and PTA.Citation52
Figure 6 TEM micrographs of iron oxide magnetic nanoparticles (A), chitosan-iron oxide magnetic nanoparticles (B), and PTA-CS-MNP nanocomposite (C). Particle size distribution of iron oxide magnetic nanoparticles (D), chitosan-iron oxide magnetic nanoparticles (E), and PTA-CS-MNP nanocomposite (F).
Abbreviations: PTA-CS-MNP, phytic acid-chitosan-iron oxide nanocomposite; TEM, transmission electron microscopy.
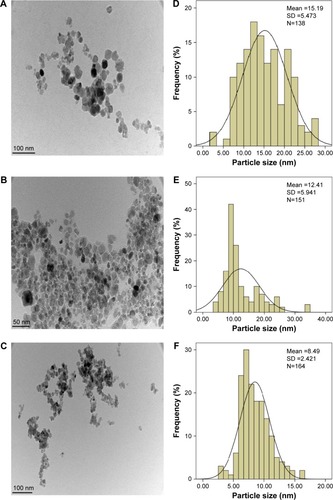
In vitro release study of PTA from PTA-CS-MNP nanocomposite
The UV-Vis absorption spectroscopy and calculations by calibration curve equation showed that the percentage loading of PTA in the PTA-CS-MNP nanocomposite was 12.9%. The release profiles of PTA from the PTA-CS-MNP nanocomposite and physical mixture are shown in . The physical mixture of PTA sodium salt with CS and MNPs exposed to pH 4.8 or pH 7.4 environment shows that the release of PTA was fast and completed within 60 seconds (inset in ). However, the release rate of PTA from the PTA-CS-MNP nanocomposite was markedly slower than that from the physical mixture, indicating that the PTA-CS-MNP nanocomposite is a potential controlled release drug-delivery system. This result may be attributed to the interaction between the negatively charged PTA and positively charged protonated CS.
Figure 7 Release profiles of phytic acid from PTA-CS-MNP nanocomposite into phosphate-buffered saline solution at pH 7.4 and pH 4.8. The inset shows the release profiles of a physical mixture of phytic acid sodium salt with chitosan and iron oxide nanoparticles into phosphate-buffered saline solution at pH 7.4 and pH 4.8.
Abbreviation: PTA-CS-MNP, phytic acid-chitosan-iron oxide nanocomposite.
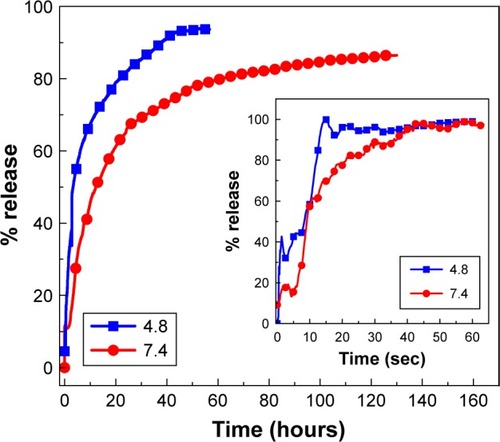
The release rate of PTA from PTA-CS-MNP nanocomposite is dependent on the pH of the environment; the release rate of PTA at pH 7.4 was remarkably lower than that at pH 4.8. shows that the percent release of drug from nanocomposite reached 93% within 56 hours when exposed to a pH environment of 4.8 and 86% by 127 hours at pH 7.4 PBS.
Such a difference in the release rate at pH 4.8 and 7.4 might be due to a possible difference in the release mechanism of PTA from the nanocomposite. At acidic pH (pH 4.8), PTA-CS-MNP nanocomposite is unstable, but at pH 7.4, it is more stable.Citation55,Citation56 As a result, the release would occur through ion exchange between PTA anions and negative anions available on the PBS.
Release kinetics of PTA from PTA-CS-MNP nanocomposite
A number of kinetic models describe the total release of PTA from PTA-CS-MNP nanocomposite. The common models used include pseudo-first order, pseudo-second order, and parabolic diffusion models. Pseudo-first order kinetic equation is written as follows:Citation57
where qe and qt are the equilibrium release amount and the release amount at time t, respectively, and k is the constant of the corresponding release rate.
In pseudo-second order kinetic model, the release behavior of drugs from nanocomposite is described by the following equation:Citation58
By plotting t/qt against t, a straight line is obtained and the release rate constant k, as well as qe, can be calculated using a the following relation:
The parabolic diffusion kinetic model is written as follows:Citation59
where Mo and Mt are the PTA content that remain in the nanocomposite at release time 0 and t, respectively.
Among these three kinetic models, it was found that the release kinetic processes of PTA from PTA-CS-MNP nanocomposite at pH 7.4 and 4.8 were well governed by the pseudo-second order model, with correlation coefficients of (R2) 0.9996 and 0.9980 and release rate constant (k) values of 1.69×10−5 and 2.86×10−5 mg/min, respectively ( and ).
Table 1 Correlation coefficient (R2), rate constant (k), and half-life (t1/2) values obtained by fitting the release data of phytic acid from PTA-CS-MNP nanocomposite into phosphate-buffered saline solution at pH 4.8 and 7.4
Figure 8 Fitting of the data for phytic acid release from PTA-CS-MNP nanocomposite into various solutions to the pseudo-first order, pseudo-second order kinetics and parabolic diffusion model for pH 7.4 (A–C) and pH 4.8 (D–F).
Abbreviation: PTA-CS-MNP, phytic acid-chitosan-iron oxide nanocomposite.
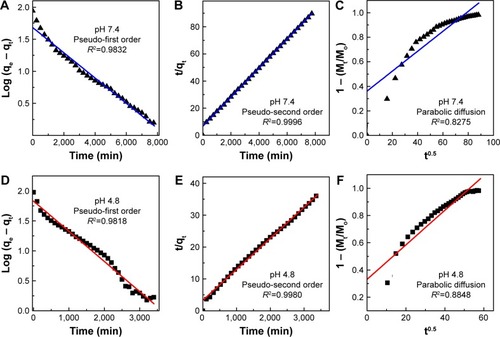
The results of kinetic model gained in this work are similar to the release kinetic study of the chlorogenic acid intercalated into the Zn/Al-LDH, Mg/Al-LDH by ion-exchange and coprecipitation routes and ZLHCitation58,Citation60,Citation61 and also very similar to release of protocatechuic acid from Zn/Al-layered double hydroxide and zinc layered hydroxide.Citation62,Citation63
Cellular sensitivity of cells to PTA and PTA-CS-MNP nanocomposite
The efficacy of PTA-CS-MNP nanocomposite in suppressing the growth of HT29 colorectal adenocarcinoma cells was assessed using colorimetric assay (MTT assay). The 3T3 normal fibroblast cells were employed as normal control to ensure the nontoxic nature of nanocomposite. As illustrated in , HT29 cells displayed a dose-dependent reduction in cell viability after 72 hours of incubation with nanocomposite. PTA-CS-MNP nanocomposite exerted a cytotoxic effect on HT29 at higher concentrations and showed a moderate inhibition at lower concentrations. In general, pure compound exhibited a much lower cytotoxic effect on HT29 cells with a higher half maximal inhibitory concentration value (IC50) of 188.5 μg/mL compared to PTA-CS-MNP nanocomposite, whose IC50 value was 45.63 μg/mL. This shows that the introduction of CS-MNPs into PTA improved the efficacy of PTA in HT29 cells. It is apparent from that both free PTA and nanocomposite did not induce toxicity in 3T3 normal fibroblast cells. Both compounds did not affect cell viability in the tested range, as the survival was consistently greater than 80% or similar to control. In this case, it can be suggested that PTA-CS-MNP nanocomposite possessed good anticancer activities when compared with pure compound PTA and demonstrated selectivity between cancerous and normal cells.
Conclusion
PTA-CS-MNP nanocomposite was successfully prepared by coating MNPs with CS and then loading it with PTA drug. The loading percentage of PTA into the nanocomposite was 12.9% and the superparamagnetic properties of PTA-CS-MNP nanocomposite and MNPs were shown by vibrating sample magnetometry studies. The vibration modes of the coated CS to MNPs and loaded PTA, which supported the formation of PTA-CS-MNP nanocomposite, were confirmed by FTIR. The release profiles of the drug from nanocomposite into PBS were of sustained manner with total release equilibria of 86% and 93% when exposed to environments of pH 7.4 and 4.8 at 127 and 56 hours, respectively. The pseudo-second order kinetics model was found to be the best fitting mathematical model. Overall, the findings of this study suggest that PTA-CS-MNP nanocomposites possess good anticancer potential against colon cancer cells and do not cause any cytotoxicity to normal fibroblast cells. Further, cellular and molecular studies are needed to find out the specific cellular mechanism that describes the specific action of nanocomposite against colon cancer cells. Also, in vivo studies are to be carried out to prove the therapeutic potential of the present tumor-specific delivery system.
Acknowledgments
We thank the Ministry of Science Technology and Innovation of Malaysia (MOSTI) for funding the project under grant No: 02-01-04-SF2141.
Disclosure
The authors report no conflicts of interest in this work.
References
- BoisseauPLoubatonBNanomedicine, nanotechnology in medicineComptes Rendus Physique2011127620636
- MisraRAcharyaSSahooSKCancer nanotechnology: application of nanotechnology in cancer therapyDrug Discov Today20101519–2084285020727417
- NguyenTXHuangLGauthierMYangGWangQRecent advances in liposome surface modification for oral drug deliveryNanomedicine (Lond)20161191169118527074098
- LiMHYuHWangTFTamoxifen embedded in lipid bilayer improves the oncotarget of liposomal daunorubicin in vivoJ Mater Chem B201421216191625
- DuDChangNSunSThe role of glucose transporters in the distribution of p-aminophenyl-α-d-mannopyranoside modified liposomes within mice brainJ Control Release20141829911024631863
- CaoJWangRGaoNA7RC peptide modified paclitaxel liposomes dually target breast cancerBiomater Sci20153121545155426291480
- WangQChengHPengHZhouHLiPYLangerRNon-genetic engineering of cells for drug delivery and cell-based therapyAdv Drug Deliv Rev20159112514025543006
- PengHLiuXWangGPolymeric multifunctional nanomaterials for theranosticsJ Mater Chem B201533468566870
- McCallionCBurthemJRees-UnwinKGolovanovAPluenAGraphene in therapeutics delivery: problems, solutions and future opportunitiesEur J Pharm Biopharm201610423525027113141
- BarahuieFSaifullahBDornianiDGraphene oxide as a nanocarrier for controlled release and targeted delivery of an anticancer active agent, chlorogenic acidMater Sci Eng C201774177185
- KesharwaniPJainKJainNKDendrimer as nanocarrier for drug deliveryProg Polym Sci201439268307
- PengHWangCXuXYuCWangQAn intestinal Trojan horse for gene deliveryNanoscale20157104354436025619169
- BarahuieFHusseinMZFakuraziSZainalZDevelopment of drug delivery systems based on layered hydroxides for nanomedicineInt J Mol Sci20141557750778624802876
- ParsianMUnsoyGMutluPYalcinSTezcanerAGunduzULoading of Gemcitabine on chitosan magnetic nanoparticles increases the anti-cancer efficacy of the drugEur J Pharmacol201678412112827181067
- XiaoYLinZTChenYHigh molecular weight chitosan derivative polymeric micelles encapsulating superparamagnetic iron oxide for tumor-targeted magnetic resonance imagingInt J Nanomedicine2015101155117225709439
- ZhaoYBQiuZMHuangJYPreparation and analysis of Fe3O4 magnetic nanoparticles used as targeted-drug carriersChinese J Chem Eng2008163451455
- GhotbiMYbin HusseinMZControlled release study of an anti-carcinogenic agent, gallate from the surface of magnetite nanoparticlesJ Phys Chem Solids2012737936942
- AhmadTRheeIHongSChangYLeeJNi-Fe2O4 nanoparticles as contrast agents for magnetic resonance imagingJ Nanosci Nanotechnol20111175645565022121585
- LaurentSForgeDPortMMagnetic iron oxide nanoparticles: synthesis, stabilization, vectorization, physicochemical characterizations, and biological applicationsChem Rev200810862064211018543879
- ParveenSMisraRSahooSKNanoparticles: a boon to drug delivery, therapeutics, diagnostics and imagingNanomedicine20128214716621703993
- BéalleGDi CoratoRKolosnjaj-TabiJUltra magnetic liposomes for MR imaging, targeting, and hyperthermiaLangmuir20122832118341184222799267
- HirschLRStaffordRJBanksonJANanoshell-mediated near-infrared thermal therapy of tumours under magnetic resonance guidanceProc Natl Acad Sci U S A200310023135491355414597719
- QuJLiuGWangYHongRPreparation of Fe3O4-chitosan nanoparticles used for hyperthermiaAdv Powder Technol2010214461467
- DenkbaşEBKiliçayEBirliksevenCÖztürkEMagnetic chitosan microspheres: preparation and characterizationReact Funct Polym2002503225232
- LiGYJiangYRHuangKLDingPChenJPreparation and properties of magnetic Fe3O4–chitosan nanoparticlesJ Alloys Compd20084661451456
- ArturssonPLindmarkTDavisSSIllumLEffect of chitosan on the permeability of monolayers of intestinal epithelial cells (Caco-2)Pharm Res200411913581361
- HejaziRAmijiMChitosan-based gastrointestinal delivery systemsJ Control Release200389215116512711440
- MiaoYTanSNAmperometric hydrogen peroxide biosensor based on immobilization of peroxidase in chitosan matrix crosslinked with glutaraldehydeAnalyst2000125915911594
- Ravi KumarMNA review of chitin and chitosan applicationsReact Funct Polym2000461127
- RabeaEIBadawyMEStevensCVSmaggheGSteurbautWChitosan as antimicrobial agent: applications and mode of actionBiomacromolecules2003461457146514606868
- Hussein-Al-AliSHEl ZowalatyMEHusseinMZGeilichBMWebsterTJSynthesis, characterization, and antimicrobial activity of an ampicillin-conjugated magnetic nanoantibiotic for medical applicationsInt J Nanomedicine201493801381425143729
- JavidAAhmadianSSabouryAAKalantarSMRezaei-ZarchSChitosan-coated superparamagnetic iron oxide nanoparticles for doxorubicin delivery: synthesis and anticancer effect against human ovarian cancer cellsChem Biol Drug Des201382329630623594157
- CananCCruzFTDelarozaFStudies on the extraction and purification of phytic acid from rice branJ Food Comp Anal201124710571063
- IrshadMAhmadIMehdiSJGoelHCRizviMMAntioxidant capacity and phenolic content of the aqueous extract of commonly consumed cucurbitsInt J Food Prop2014171179186
- CananCDelarozaFCasagrandeRBaracatMMShimokomakiMIdaEIAntioxidant capacity of phytic acid purified from rice branActa Sci Technol2012344457463
- TanBLNorhaizanMEScientific evidence of rice by-products for cancer prevention: chemopreventive properties of waste products from rice milling on carcinogenesis in vitro and in vivoBio Med Res Int201720179017902
- HendersonAJOllilaCAKumarAChemopreventive properties of dietary rice bran: current status and future prospectsAdv Nutr2012364365322983843
- NorhaizanMENgSKNorashareenaMSAbdahMAAntioxidant and cytotoxicity effect of rice bran phytic acid as an anticancer agent on ovarian, breast and liver cancer cell linesMalays J Nutr201117336737522655458
- NorazalinaSNorhaizanMEHairuszahINorashareenaMSAnticarcinogenic efficacy of phytic acid extracted from rice bran on azoxymethane-induced colon carcinogenesis in ratsExp Toxicol Pathol201062325926819464858
- Al-FatlawiAARizviMMAhmadAAnticarcinogenic activity of rice bran phytic acid against human breast cancer cell line (mcf-7)Asian J Pharm Clin Res201471151155
- LeeHShaoHHuangYKwakBSynthesis of MRI contrast agent by coating superparamagnetic iron oxide with chitosanIEEE Trans Magn2005411041024104
- CalmonMFde SouzaATCandidoNMA systematic study of transfection efficiency and cytotoxicity in HeLa cells using iron oxide nanoparticles prepared with organic and inorganic basesColloids Surf B Biointerfaces201210017718422766295
- DodiGHritcuDLisaGPopaMICore–shell magnetic chitosan particles functionalized by grafting: synthesis and characterizationChem Eng J2012203130141
- UnsoyGYalcinSKhodadustRGunduzGGunduzUSynthesis optimization and characterization of chitosan-coated iron oxide nanoparticles produced for biomedical applicationsJ Nanopart Res20121411964
- MarchessaultRHRavenelleFZhuXXPolysaccharides for Drug Delivery and Pharmaceutical Applications934Washington, DCAmerican Chemical Society2006
- SaburovKAKamilovKMStructure of phytic acid and phytatesChem Nat Compd1989256695698
- QuJBShaoHHJingGLHuangFPEG-chitosan-coated iron oxide nanoparticles with high saturated magnetization as carriers of 10-hydroxycamptothecin: preparation, characterization and cytotoxicity studiesColloids Surf B Biointerfaces2013102374423000675
- Hussein-Al-AliSHEl ZowalatyMEHusseinMZIsmailMWebsterTJSynthesis, characterization, controlled release, and antibacterial studies of a novel streptomycin chitosan magnetic nanoantibioticInt J Nanomedicine2014954955724549109
- DanelutiALVelascoMVBabyARMatosJDThermal behavior and free-radical-scavenging activity of phytic acid alone and incorporated in cosmetic emulsionsCosmetics201523248258
- KayalSRamanujanRVDoxorubicin loaded PVA coated iron oxide nanoparticles for targeted drug deliveryMater Sci Eng C2010303484490
- KumarRInbarajBSChenBHSurface modification of superparamagnetic iron nanoparticles with calcium salt of poly (γ-glutamic acid) as coating materialMater Res Bull2010451116031607
- YuSChowGMCarboxyl group (−CO2H) functionalized ferrimagnetic iron oxide nanoparticles for potential bio-applicationsJ Mater Chem2004141827812786
- ShanZYangWSZhangXHuangQMYeHPreparation and characterization of carboxyl-group functionalized superparamagnetic nanoparticles and the potential for bio-applicationsJ Braz Chem Soc200718713291335
- GeYZhangYXiaJEffect of surface charge and agglomerate degree of magnetic iron oxide nanoparticles on KB cellular uptake in vitroColloids Surf B Biointerfaces200973229430119564099
- BarahuieFHusseinMZHussein-Al-AliSHArulselvanPFakuraziSZainalZPreparation and controlled-release studies of protocatechuic acid-magnesium/aluminium-layered double hydroxide nanocompositeInt J Nanomedicine201381975198723737666
- KhanAIO’HareDIntercalation chemistry of layered double hydroxides: recent developments and applicationsJ Mater Chem2002121131913198
- DongLYanLHouWGLiuSJSynthesis and release behavior of composites of camptothecin and layered double hydroxideJ Solid State Chem2010183818111816
- BarahuieFHusseinMZArulselvanPFakuraziSZainalZDevelopment of the anticancer potential of a chlorogenate-zinc layered hydroxide nanohybrid with controlled release property against various cancer cellsSci Adv Mater201351219831993
- HoYSOfomajaAEPseudo-second-order model for lead ion sorption from aqueous solutions onto palm kernel fiberJ Hazard Mater20061291–313714216188379
- BarahuieFHusseinMZArulselvanPFakuraziSZainalZDrug delivery system for an anticancer agent, chlorogenate-Zn/Al-layered double hydroxide nanohybrid synthesised using direct co-precipitation and ion exchange methodsJ Solid State Chem20142173141
- BarahuieFHusseinMZArulselvanPFakuraziSZainalZControlled in vitro release of the anticancer drug chlorogenic acid using magnesium/aluminium-layered double hydroxide as a nanomatrixSci Adv Mater201683501513
- BarahuieFHusseinMZAbd GaniSFakuraziSZainalZSynthesis of protocatechuic acid-zinc/aluminium layered double hydroxide nanocomposite as an anticancer nanodelivery systemJ Solid State Chem20152212131
- BarahuieFHusseinMZAbd GaniSFakuraziSZainalZAnticancer nanodelivery system with controlled release property based on protocatechuate-zinc layered hydroxide nanohybridInt J Nanomedicine201493137314925061291