Abstract
Due to a growing trend in their biomedical application, biosynthesized nanomaterials are of great interest to researchers nowadays with their biocompatible, low-energy consumption, economic, and tunable characteristics. It is important to understand the mechanism of biosynthesis in order to achieve more efficient applications. Since there are only rare studies on the influences of cellular energy levels on biosynthesis, the influence of energy is often overlooked. Through determination of the intracellular ATP concentrations during the biosynthesis process, significant changes were observed. In addition, ATP synthesis deficiency caused great decreases in quantum dots (QDs) biosynthesis in the Δatp1, Δatp2, Δatp14, and Δatp17 strains. With inductively coupled plasma-atomic emission spectrometry and atomic absorption spectroscopy analyses, it was found that ATP affected the accumulation of the seleno-precursor and helped with the uptake of Cd and the formation of QDs. We successfully enhanced the fluorescence intensity 1.5 or 2 times through genetic modification to increase ATP or SeAM (the seleno analog of S-adenosylmethionine, the product that would accumulate when ATP is accrued). This work explains the mechanism for the correlation of the cellular energy level and QDs biosynthesis in living cells, demonstrates control of the biosynthesis using this mechanism, and thus provides a new manipulation strategy for the biosynthesis of other nanomaterials to widen their applications.
Introduction
Nanomaterials,Citation1 due to their unique properties, have been widely used in cell imaging,Citation2,Citation3 diagnosis,Citation4,Citation5 biosensing,Citation6,Citation7 electro-optic device,Citation8 and so on, especially on medical imaging because of their (surface modified nanomaterials) high biocompatibility to cells. Thus, tremendous interest has arisen in the synthesis of well-dispersed and uniform-sized nanomaterials.Citation9,Citation10 Nanoparticles biosynthesized by organisms, without involving the toxic solvents, strict conditions, or expensive processes that are needed in chemical methods, have become a popular subject.Citation11 Various nanoparticles are reported that are biosynthesized by bacteria,Citation12–Citation14 fungi,Citation15,Citation16 plants,Citation17,Citation18 mammalian cells,Citation19 or even earthworms.Citation20 Considering the simple culture methods, the low cost of the equipment, and the easy way to get the gradients, the biosynthesis of nanoparticles using microorganisms is the most economic and common approach.
There have been many investigations on the biosynthesis of nanomaterials, mainly concerning their applications.Citation21 In order to obtain nanoparticles with a defined expectation in the biosynthesis, whether through optimizing the culture conditions or adjusting the concentrations of primary substrates, former studies were devoted to manipulating the biosynthesis by extrinsic factors, and few were on the intrinsic characters of the microorganisms applied in the biosynthesis. Ayano et alCitation22 found that the optimal culture conditions to biosynthesize CdSe nanoparticles by Pseudomonas aeruginosa were when the cells were cultured at 25°C–40°C, 0.05–10 g L−1 of NaCl concentration, and neutral pH. According to Gericke and Pinches,Citation23 the size and shape of gold nanoparticles biosynthesized by fungus could be manipulated by changing crucial growth parameters. They successfully obtained spherical, hexagonal, and triangular-shaped nanoparticles with different sizes through culturing under various pH and temperature conditions. Besides the studies of the adscititious substances and the culture conditions, there have also been some studies focused on the intrinsic metabolisms of the bioreactor used in the biosynthesis. For example, for Sb2O3 nanoparticles biosynthesized by yeast, reported by Jha et al,Citation24 it was suggested that the membrane-bound and cytosolic oxidoreductases and quinines might be the key influential factors of the process. Phenol oxidases in the Lentinus edodes were proved to be responsible for the biosynthesis of Au nanoparticles.Citation25 In general, current research has mostly focused on the culture conditions or the substances, while the role of energy has hardly been mentioned.
In the previous work of our laboratory, we constructed a convenient way to biosynthesize CdSe quantum dots (QDs) with baker’s yeast Saccharomyces cerevisiaeCitation26 and then promoted the QDs production by genetic modification.Citation27 Glutathione (l-γ-glutamylcysteinylglycine, GSH) was found to be a vital compound in the biosynthesis of CdSe QDs, the intracellular content of which showed synchronized increase with the fluorescence intensities when seleniumized cells were initially incubated with CdCl2.Citation27 Besides the substances that assisted with the biosynthesis of CdSe QDs, GO (gene ontology) analysis revealed that the proteins that encapsulated the CdSe QDs biosynthesized by yeast mostly functioned in cell energy metabolism (unpublished data); thus, this may indicate that energy has a noticeable role in the process. There may be a need for not only micromolecular polypeptides (such as GSH)Citation27 but also cell energy to ensure the ambient temperature and strict conditions for the stable biosynthesis of CdSe QDs with good dispersal and uniformity. With its favorable biocompatibility characteristic, the system was successfully applied to construct a cell beacon using Staphylococcus aureus as a bioreactor.Citation28
Figuring out the actual role that energy plays in the biosynthesis would be conducive to further understanding of the mechanism.Citation26,Citation27 The present study used the concentrations of intracellular ATP (the most commonly used direct energy resource) and fluorescence intensities as indicators to investigate the influences of energy on the biosynthesis of CdSe QDs in yeast. Intracellular Se or Cd concentrations were checked during the cell-seleniumized phase or the crystallization phaseCitation26 with inductively coupled plasma-atomic emission spectrometry (ICP-AES) and atomic absorption spectroscopy (AAS) to confirm the influences on the absorption of the substrates and the crystallization of CdSe QDs. Finally, the fluorescence intensities were improved by genetic modification of the ATP metabolism pathway. In consequence, the biosynthesis can be controlled with defined expectation and it can be used as guidance for the biosynthesis of other similar nanoparticles with yeast or other microorganisms. The present work provides a new perspective for future research on the biosynthesis mechanisms of nanomaterials and makes it easier to convert the biosynthesized nanomaterials into applications.
Materials and methods
Strains and media
Saccharomyces cerevisiae BY4742 (MATα his3-Δ1 leu2-Δ0 lys2-Δ0 ura3-Δ0) (wild-type, WT) and the Δatp1, Δatp2, Δatp14, Δatp17, Δpfk1, and Δrgt2 mutant strains were obtained from the European Saccharomyces cerevisiae Archive for Functional Analysis (Bad Homburg, Germany) (all strain information is listed in Table S1). The PGAL1-ADK1 and PGAL1-SAM2 strains were constructed in this study (Table S2). Strains were cultured in 1 g L−1 yeast extract and 2 g L−1 peptone (YP), supplied with 2 g L−1 glucose (YPGlu) or YP supplied with 2 g L−1 galactose (YPGal) media. Unless otherwise indicated, the reagents used in the present work were obtained from the Sinopharm Chemical Reagent Co. (Shanghai, China).
Biosynthesis of CdSe QDs
The biosynthesis of CdSe QDs with yeast was performed according to the previous study,Citation26 with a slight adjustment, as follows: stationary phase cells were co-incubated with Na2SeO3 at a final concentration of 2.5 mM for 24 hours to get seleniumized (hereafter referred to as seleniumized cells), and then the cells were collected by centrifugation at 3,000 g for 1 min. The cell pellets were resuspended in an equal volume of fresh medium and then co-incubated with CdCl2 at a final concentration of 1 mM to synthesize CdSe QDs (the biosynthesis procedure was presented in Figure S1). After incubation for 24 hours, the fluorescence intensity was measured. The cells were cultivated under 30°C at 200 revolutions per minute (rpm) (cells’ growing curves are shown in Figure S2).
To avoid influences of the cell biomass, all the cultures were adjusted to the same cell density (optical density [OD]600=12.0) before co-incubating with Na2SeO3.
Intracellular CdSe QD fluorescence intensity measurement
Cell samples were collected after biosynthesis for fluorescence measurement (with the OD600 of 6.0) and washed with 1 mL of 1× phosphate-buffered saline (PBS, pH 7.5) and then resuspended with 1 mL of 1× PBS (pH 7.5) before fluorescence intensity determination. The intracellular CdSe QDs fluorescence intensity was calculated by subtraction of the cellular autofluorescence (seleniumized cells) from the total fluorescence. All fluorescence spectra of the cells synthesizing QDs and seleniumized cells were acquired with a Cytation3 Multi-mode Reader from BioTek (Winooski, VT, USA), with an excitation wavelength of 400 nm and the detection emission spectrum of 450 to 700 nm.
Determination of intracellular ATP levels
Samples of 1×107 cells (OD600=1.0) were harvested and washed with 200 μL sterilized ice-cold deionized water three times. Then, cell pellets were resuspended in 500 μL of ATP determination lysis solution and transferred to 2 mL snap-cap tubes with 0.5 g glass beads (425–600 μm in diameter; Sigma, St Louis, MO, USA). Cells were ruptured with a Mini-Bead beater-16 (BioSpec, Bartlesville, OK, USA) for 1 min on and 1 min pause on ice to dissipate heat, and repeated for two times. Then, the tubes were centrifuged at 16,000 g, at 4°C, for 5 min, and the supernatants were collected in new centrifuge tubes. Samples were then processed and intracellular ATP concentrations were determined as described in the protocol of the ATP Assay Kit (S0026; Beyotime, Shanghai, China) with a Cytation3 Multi-mode Reader from BioTek.
Determination of intracellular GSH levels
Samples of 1×107 cells (OD600=1.0) were centrifuged and harvested, and then the pellets were washed with 200 μL of sterilized ice-cold 1× PBS (pH 7.5) two times. The cell pellets were resuspended with 160 μL of sterilized ice-cold deionized water, followed by the addition of 160 μL of sterilized ice-cold 20 mM HCl and 80 μL of sterilized ice-cold 5% (wt/vol) 5-sulfosalicylic acid. The suspensions were transferred to 2 mL snap-cap tubes with 0.5 g acid-washed glass beads, and the cells were ruptured with a Mini-Bead beater-16 (BioSpec) for 1 min on and 2 min pause on ice, and repeated once. Then, the tubes were centrifuged at 13,000 g, at 4°C, for 15 min, and the supernatants were collected in new centrifuge tubes. Samples were then processed and the intracellular GSH concentrations were measured as described in the protocol of the Total Glutathione Assay Kit (S0052; Beyotime) by a Cytation3 Multi-mode Reader from BioTek.
ICP-AES and AAS analysis
Cell suspensions (2 mL) were centrifuged at 5,000 g for 3 min, and then the supernatants were collected in centrifuge tubes before filtering with a 0.22-μm filter unit (Merck Millipore, Billerica, MA, USA). HNO3 and water (Milli-Q, 18.2 MΩ; Merck Millipore) were added to the solutions to get a final concentration of 1% (vol/vol), and the samples were stored at 4°C until needed. The cell pellets were collected and washed with ice-cold 1× PBS before resuspension with 500 μL of water (Milli-Q, 18.2 MΩ), and then transferred to polytetrafluoroethylene reactors. After 2 mL of HNO3 had been added to the samples, they were placed on a heating plate at 120°C for 2 hours and then at 90°C until the residues were around 100 μL. HNO3 (1%, vol/vol) was added to make a total volume of 1 mL and diluted to 10 mL with water (Milli-Q, 18.2 MΩ) prior to determination. The Se concentrations in the supernatants or inside the cells were determined with ICP-AES (IRIS II; Thermo Fisher Scientific, Fair Lawn, NJ, USA). The Cd concentrations were characterized using AAS with a ContrAA 700 high-resolution continuous light source atomic absorption spectrophotometer (Analytik Jena, Jena, Germany).
Seleno-compounds separation and characterization
A wet weight of 0.5 g seleniumized cells was collected and washed with 1× PBS (pH 7.5), and the cell pellet was then resuspended with 1× PBS (pH 7.5). Cells were disrupted with a Mini-Bead beater-16 (BioSpec) for 1 min on and 2 min pause on ice, twice. Samples were centrifuged to get the lysate, the protein concentrations of the samples were determined by the Bradford method,Citation29 and then adjusted to the same value in all samples. Trypsin (100 mg) (Amersco, Solon, OH, USA) and TCS buffer (10×, 0.1 M Tris–Cl, pH 7.5, 10 mM CaCl2, 0.5% SDS) were added to the samples and then they were incubated at 37°C for 20 h. After centrifugation, the supernatants were collected and filtered with 0.22-μm filter units. Then, the samples were subjected to step-by-step ultrafiltration with 100 KD, 50 KD, 10 KD, and 3 KD Millipore ultrafiltration tubes at 4,500 rpm for 15 min. Then, the prepared samples were used for high-performance liquid chromatography (HPLC) with ICP mass spectrometry (MS) (HPLC–ICP-MS) measurement, which was performed on an Agilent 7500 ICP-MS system (Agilent, Santa Clara, CA, USA), coupled with a HPLC system (Shimadzu, Kyoto, Japan) equipped with a CAPCELL PAK C18 column (Shiseido, Tokyo, Japan).
Improving the biosynthesis of CdSe QDs
The promoter of gene ADK1 or SAM2 was replaced with a stronger promoter, PGAL1, through homologous recombination (details of the construction process are in Supplementary materials) to get overexpression. The modified strains were used to biosynthesize CdSe QDs cultured in YPGal medium (with galactose as an inducer of the overexpression).
Statistical analysis
All statistical analyses were performed using Origin 2016. The schematic diagram was made with Photoshop CC. Graph data are presented as means and SD. Two-sample t-tests were used on the data where appropriate.
Results
Intracellular ATP concentrations during yeast QDs biosynthesis
To confirm the influence of cellular ATP levels on the CdSe QDs biosynthesis in S. cerevisiae, cells of different processing stages of CdSe QDs biosynthesis were collected to determine the concentrations of intracellular ATP. The intracellular ATP concentrations of the WT cells were measured during a 12-hour incubation with Na2SeO3 at a final concentration of 2.5 mM; a sharp peak appeared at 2 hours after the introduction of Na2SeO3 into the culture (). Starting from the exponential phase, the ATP concentrations of the sample cells all sharply increased right after either Na2SeO3 or CdCl2 was added to the culture, and then rapidly reduced. The ATP concentrations of the control group remained stable, with a slight fluctuation at 24- to 48-hour cultivation; and after the cells were transformed to fresh medium at 48 hours, the concentrations decreased at first and then increased substantially. Clearly, the intracellular ATP concentrations had obvious changes during the process of QDs biosynthesis in the sample cells, while the control group had no such changes ().
Figure 1 Intracellular ATP concentrations during CdSe QDs biosynthesis.
Notes: (A) ATP concentrations during the 12-hour incubation with Na2SeO3; (B) ATP concentrations at different collection times during the whole process, from the cells in exponential phase to 24 hours after seleniumized cells were co-incubated with CdCl2. Vertical lines divide the process into three phases. The cell’s growing phase is from 0 to 24 hours; Na2SeO3 was added at 24 hours. At 48 hours, cell pellets were transferred to fresh medium and CdCl2 was added to the culture.
Abbreviations: OD, optical density; QDs, quantum dots.
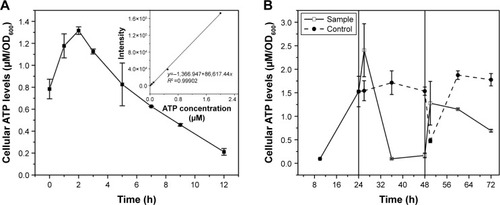
CdSe QDs biosynthesis under low ATP synthesis conditions
To inspect the relation between ATP synthesis and CdSe QDs biosynthesis, several ATP-synthesis-deficient strains were introduced. S. cerevisiae strain Δatp1 displays a complete loss of ATP synthase activity.Citation30 The determination of the intracellular ATP concentration, with half the value of WT cells, proved that the Δatp1 strain was deficient in ATP synthesis (). The fluorescence intensity of Δatp1 was just a quarter of that in the WT cells (), which was consistent with the ATP concentration changes of the two strains. Other gene knock-out strains that were deficient in ATP synthesis, Δatp2,Citation31 Δatp14,Citation32 and Δatp17,Citation33 were also applied in the synthesis of QDs to further confirm the results, with an outcome concurrent with that for the Δatp1 strain, namely much lower intracellular ATP concentrations and QDs fluorescence intensities than for WT cells (). The fluorescence intensities of Δatp2, Δatp14, and Δatp17 cells were 1/2, 2/5, and 1/4 () of the WT cells, whereas the ATP concentrations were 1/2, 1/10, and 1/8 () of the WT cells, respectively. The fluorescence intensities roughly conformed to the intracellular ATP concentrations.
Figure 2 (A) Intracellular fluorescence intensities and (B) ATP concentrations of WT and Δatp1 cells, (C) intracellular fluorescence intensities and (D) ATP concentrations of some ATP-synthesis-deficient strains.
Abbreviations: CPS, counts per second; FL, fluorescence; OD, optical density; WT, wild-type.
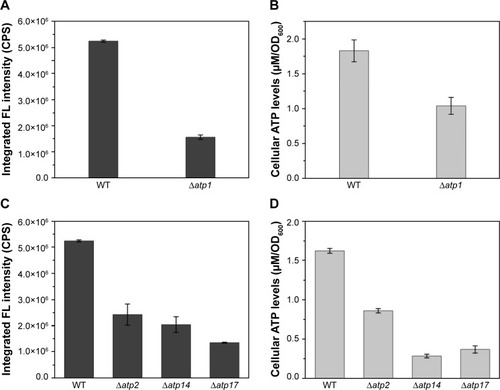
Total intracellular GSH of the Δatp1 strain and its QDs synthesis ability
As to the specific reason for the influence of ATP on QDs biosynthesis, we suspected it was due to effects on intracellular GSH synthesis. In our previous study, we found that GSH was important for the QDs biosynthesis and the intracellular GSH content showed synchronized increase with the fluorescence intensity,Citation26,Citation27 so the GSH contents were checked in this study too. Interestingly, when the total GSH concentrations were measured in the Δatp1 strain after seleniumized cells were treated with CdCl2, that tendency referred to above disappeared. Instead, the GSH level kept steady around a certain value (about 9 μM per 1 OD600 cells) with only a slight fluctuation, yet somehow was higher than that of WT cells (). At time 0, when the CdCl2 was added to the culture, GSH concentrations in Δatp1 and WT cells were 6.72 and 2.95 μM per 1 OD600 cells, respectively. Nevertheless, GSH concentrations in the WT cells presented a trend of gradual increase with culturing time for seleniumized cells incubated with CdCl2. The intracellular fluorescence intensities, however, had the same tendency of gradual increase in both WT and Δatp1 strains, but with a growing difference between them ().
Figure 3 Intracellular GSH contents (A) and intracellular fluorescence intensities (B) of seleniumized WT and Δatp1 cells incubated with CdCl2 during the first 7 hours.
Abbreviations: CPS, counts per second; FL, fluorescence; GSH, glutathione (l-γ-glutamylcysteinylglycine); OD, optical density; WT, wild-type.
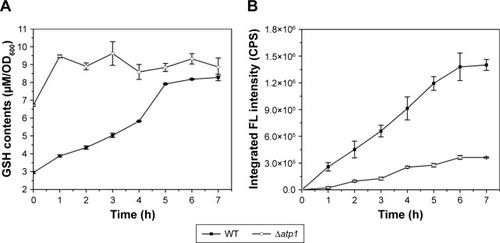
Se and Cd concentrations during co-incubation with yeast cells while synthesizing QDs (ICP-AES and AAS assays)
Both the intracellular and extracellular concentrations of Se and Cd were measured during the QDs biosynthesis processes of WT and Δatp1 strains. Within error, nearly all Se or Cd lost from the medium was absorbed into the cells (). Although compared with the amount of Na2SeO3 (final concentration of 2.5 mM) or CdCl2 (final concentration of 1 mM) added to the culture (which was the optimized amount for CdSe QDs biosynthesis, data not shown), WT and Δatp1 both just took a small amount inside the cells; they did have the same trend of absorption of both compounds (). The differences were evident between WT and Δatp1 strains. The Se uptake of WT was quite conspicuous at first and then slowed down and stabilized after incubation for 12 hours, reaching a final concentration of 0.48±0.029 mM, whereas the Se concentration inside Δatp1 cells was negligible with a concentration of 0.04±0.016 mM. The intracellular Se differences between Δatp1 and WT began to emerge 2 hours after the addition of Na2SeO3 and increased during the culturing over the following 24 hours (). A similar result was observed with the uptake of Cd, the increase tendency of the two strains was the same, only with a lower concentration in Δatp1 cells. The final concentration in Δatp1 cells was 0.10±0.004 mM, less than half of that in WT cells, which was 0.27±0.045 mM ().
Major seleno-amino acid determination in Δatp1 and WT cells
Seleniumized cells of WT and Δatp1 strains were collected, and their total proteins were extracted and digested to oligopeptides with trypsin to measure the main reduced Se species inside. On the basis of the previous study,Citation26 organoSe compounds selenocystine [(Se-Cys)2], Se-methylseleno-l-cysteine (SeMC), and d,l-selenomethionine (Se-Met) were checked. HPLC–ICP-MS showed that the major seleno-amino acid (Se-Cys)2 signal in the WT sample was obviously stronger than that in the Δatp1 sample (). The peak height of (Se-Cys)2 in Δatp1 cells was half that of WT cells.
Figure 5 HPLC-ICP-MS chromatogram of Se species standards (ST) and seleno-amino acids characterized from WT and Δatp1 cells after treatment with Na2SeO3. The Se species standards are: 1, l-selenocystine; 2, Se-methylseleno-l-cysteine; 3, d,l-selenomethionine.
Abbreviations: HPLC-ICP-MS, high-performance liquid chromatography–inductively coupled plasma mass spectrometry; WT, wild-type.
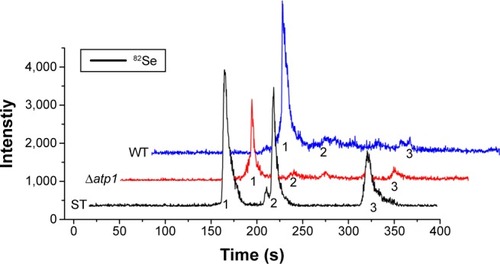
CdSe QDs biosynthesis under the influences of oxidative phosphorylation inhibitor or uncoupler
To investigate the effects of ATP on the absorption of Cd and QDs crystallization, the intracellular fluorescence intensities of cells under different concentrations of oxidative phosphorylation inhibitor (NaN3) or uncoupler (2,4- dinitrophenol, DNP), which were added to the culture during the incubation of seleniumized cells with CdCl2, were measured. The results in show that the intracellular fluorescence intensities decreased to less than half of the normal value along with the increase in the NaN3 concentrations from 75 to 200 mM, indicating the remarkable influence of the addition of NaN3 on QDs biosynthesis. When DNP was introduced into the culture, intracellular fluorescence intensities had a slight yet noticeable reduction (). At the same time, Cd concentrations of seleniumized cells incubated with CdCl2 were checked in the presence of 150 mM NaN3 or 2 mM DNP, with concentrations that had visible impacts on CdSe QDs biosynthesis (). Differences were not noticeable in the fluorescence intensities.
Figure 6 Intracellular fluorescence intensities of WT cells treated with different concentrations of NaN3 (A) or DNP (B), and Cd concentrations in the culture supernatants of cells samples treated with 150 mM NaN3 and 2 mM DNP (C) and when co-incubated with CdCl2.
Abbreviations: DNP, 2,4-dinitrophenol; FL, fluorescence; WT, wild-type.
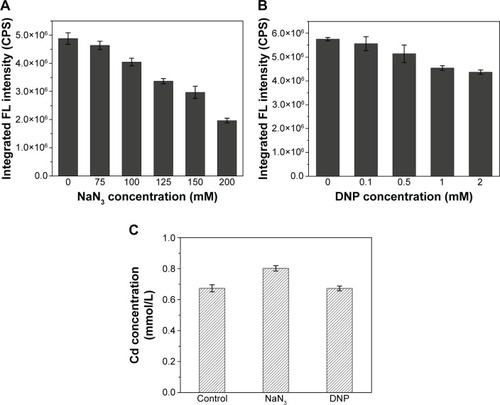
QDs biosynthesis manipulation on the basis of ATP synthesis
To investigate the use of energy influence in the manipulation of biosynthesis, genetic modification of the energy metabolism pathway was introduced. Overexpression of the ADK1 gene leads to an accumulation of ATP levels inside the cells. When the promoter of ADK1 gene was replaced with a stronger promoter, the intracellular ATP concentration increased nearly two times (). shows that the intracellular fluorescence intensity was twice that in the WT cells.
Discussion
This study was approached to determine the specific influence of energy on CdSe QDs biosynthesis by yeast and took advantage of it to manipulate the biosynthesis process, for the reason that most of the proteins that encapsulated the QDs were functioned in cell energy metabolism (with the result of bioinformatics analysis, data not shown).
According to Li et al,Citation27 the system in the present work uses “temporally–spatially coupling strategy” to biosynthesize CdSe QDs. Within this system, there are three phases: the cell-growing phase, the cell-seleniumized phase, and the CdSe QDs crystallization phase. Herein, the influence of ATP on the biosynthesis is carried out through the three crucial phases and mainly through the latter two phases.
To confirm the importance of the influence of ATP on biosynthesis, the intracellular ATP concentrations were determined. The ATP levels had significant variations not only in the cell-seleniumized phase () but also in the whole procedure (). In contrast, the intracellular ATP concentrations of the control group () were consistent with the cell growing state.Citation34 To further confirm our suspicions, we used ATP-synthesis-deficient strains (Δatp1, Δatp2, Δatp14, and Δatp17) to biosynthesize CdSe QDs. The fluorescence intensities of the strains were as expected (). The fluorescence intensities and ATP concentrations of Δatp2, Δatp14, and Δatp17 (fermentation-related genes were not taken into consideration because cells were not undertaking fermentative metabolism to generate ATP in the cell-seleniumized phase, Figures S3 and S4) were not exactly accordant (), which may be due to other influential factors. In this case, we proposed that CdSe QDs biosynthesis by yeast cells is an energy-consuming process, besides having many other necessary conditions.Citation27 In the quasi-biosystem referred to by Gu et al,Citation35 they only need several substrates and co-factors to synthesize nanomaterials, but the temperature must reach at least 80°C–90°C, which was significantly higher than that for the biosynthesis. Hence, it may suggest that ATP synthesis inside the cells meets with the request of energy in the biosynthesis.
As already reported, GSH synthesis is an energy- consuming process.Citation36 It was reasonable to hypothesize that the ATP effects may be due to its influence on the synthesis of GSH with its important role in our system.Citation26,Citation27 Interestingly, when the GSH contents were determined in the Δatp1 strain, the tendency of GSH levels increasing with fluorescence intensities, referred to above, did not appear (). The content level was higher than that of WT cells during the testing period, which may be because the addition of CdCl2 in seleniumized cells stimulated an increase of GSH.Citation37 And because the addition of Na2SeO3 would trigger the upregulation of GSH,Citation27 with lower intracellular Se concentration, the GSH cannot be used effectivelyCitation38 and results in the higher GSH content in Δatp1 cells at time 0 than that of WT cells. These results () attested that within the low ATP synthesis strains (such as Δatp1) mentioned, it was the utilization rather than synthesis of GSH that was impeded by the ATP levels.
With the results above, it was shown that ATP had a significant influence on CdSe QDs biosynthesis by yeast. Determining where ATP had its effects was important and urgent for its not insignificant role in the biosynthesis. Since there were two critical phases in the biosynthesis process, the influences of ATP were investigated through these two phases. shows the intracellular and the extracellular concentrations of the Se or Cd measured during the cell-seleniumized phase or the crystallization phase. The shortage of ATP synthesis caused an absorption deficiency of both elements constructing CdSe QDs, resulting in poor fluorescence intensity. We suspected this could be linked to the way that SeO32− or Cd2+ entered the cells, which might need ATP.Citation39,Citation40
Once inside the cells, Se (+4) ions are reduced to Se (−2) ions, then the Cd2+ interacts with the seleno-precursors and forms QDs crystals. The main seleno-amino acids emerging during the biosynthesis, as the previous study suggested,Citation26 were detected by HPLC–ICP-MS.Citation41 In , it is shown that the major seleno-amino acid peak heights in Δatp1 and WT samples were consistent with the fluorescence intensities between them (). The result was proof that the accumulation of the seleno-precursor was an energy-consuming process. A similar conclusion was reached for the crystallization phase. For the reason that Δatp1 cells cannot normally accumulate seleno-precursors, the oxidative phosphorylation inhibitor and uncoupler were introduced into the system instead. Both the inhibitor and uncoupler could induce the decline of the intracellular ATP concentration, except that the uncoupler had no influence on oxidoreduction.Citation42,Citation43 The introduction of the DNP (uncoupler) was to ensure that the reduction of the selenite was unaffected. It turned out that NaN3 had an evident impact on the crystallization (), whereas DNP had a lesser yet noticeable influence (). Under their influence, the uptake of Cd was nearly impervious () at the concentration when the intracellular ATP concentration became lower (Figure S5) (glycolysis inhibitor had been taken into consideration because the influence on ATP generation was not obvious, Figure S6). As a consequence, without altering the Cd absorption, the importance of ATP in the QDs crystallization was confirmed.
Thus, the specific influence of ATP was made clear. briefly describes the QDs biosynthesis process in yeast and shows the points of ATP action. It shows that ATP guarantees the uptake of the two inorganic compounds, helps with the accumulation of the seleno-precursors, and facilitates the utilization of the GSH accumulated during the crystallization phase. As the most direct energy resource in the organisms, ATP plays indispensable roles in many biological processes,Citation44,Citation45 but its influence in the biosynthesis of nanomaterials has often been overlooked. With the present work, we have determined the difference between the intrinsic processes and the constructed ones, thus allowing better application of the connection to bilaterally control the biosynthesis process.
Figure 8 Schematic illustration of the points of action of ATP in the CdSe QDs biosynthesis process in Saccharomyces cerevisiae.
Abbreviations: GSH, glutathione (l-γ-glutamylcysteinylglycine); QDs, quantum dots.
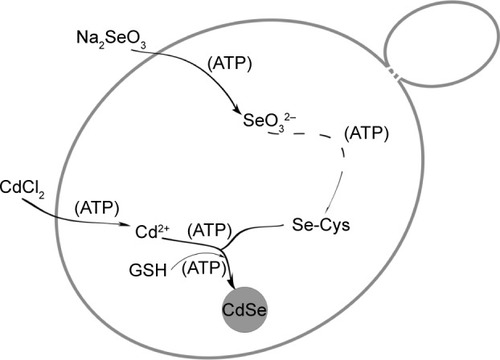
It was quite feasible that we could increase the production of the QDs through accumulation of intracellular ATP according to our findings. The overexpression of the ADK1 gene leads to the augmentation of ATP content.Citation46 Through standard genetic techniques,Citation47 the PGAL1-ADK1 strain was constructed (Figures S7 and S8). The phenotype was confirmed (), and the fluorescence intensity was consistent with the ATP levels. As it was reported, S-adenosylmethionine (SAM), one of the intermediate species of the methionine and cysteine metabolism pathway, needs methionine as a substrate and ATP for synthesis under the action of acyltransferase.Citation48 Its seleno analog, SeAM, the synthesis of which is also affected by ATP content,Citation38 is believed to be an important intermediate compound in the biosynthesis of CdSe QDs in yeast (unpublished data). An increase in ATP could lead to the accumulation of SAM, and under Se-rich culture, it would be SeAM. By direct overexpression of SAM2 gene to achieve the SAM accumulation, a PGAL1-SAM2 strain was successfully constructed (Figures S7 and S9A), and the intracellular SAM content was checked (Figure S9B). shows that the fluorescence intensity of the PGAL1-SAM2 strain was about 1.5 times of that in the WT strain, which was consistent with the results of PGAL1-ADK1 strain. In summary, it does not matter whether the ATP content was indirectly increased or an intermediate compound in the process was directly increased, as it would give the same result, namely, a prominent increase in QDs biosynthesis ability.
Conclusion
In summary, we have uncovered a vital role of ATP played in the biosynthesis of CdSe QDs by yeast without changing the QDs fluorescent properties (Figures S10 and S11). Through investigating the specific interaction processes, the biosynthesis could be promoted simply by genetic modification of the WT strain. With the present work, we can integrate substance flux and energy fluxCitation49 in the biosynthesis process. To the best of our knowledge, the role of energy in the biosynthesis of nanoparticles in microorganisms has not been reported yet, and this is the first study that has focused on energy influence, filling the gap in the research about its mechanism. Other than complementation of the understanding of the biosynthesis process, what’s more important is that this could offer a new perspective on the manipulation of nanoparticles biosynthesis using microorganisms aiding further applications.
Acknowledgments
This work was supported by the National Natural Science Foundation of China (21272182 and 31570090) and the National Basic Research Program of China (973 Program, number 2013CB933904). This project is partially supported by the Chinese 111 Project Grant B06018, the National Fund for Fostering Talents in Basic Sciences (J1103513), National Infrastructure of Natural Resources for Science and Technology Program of China (number NIMR-2015–8), and the Laboratory (Innovative) Research Fund of Wuhan University. We are grateful to XS Feng for ICP-AES and AAS measurement.
Disclosure
The authors report no conflicts of interest in this work.
Supplementary materials
Figure S1 Schematic flow chart of the biosynthetic procedure of the CdSe QDs in Saccharomyces cerevisiae.
Abbreviation: QDs, quantum dots.
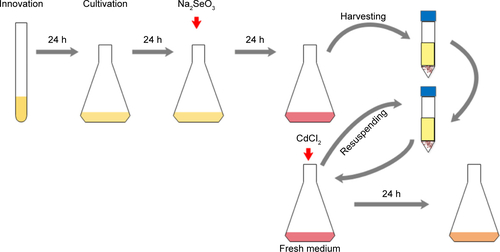
Figure S2 Growth curves of the strains in the present work cultured in YPGlu (A) or YPGal (B).
Abbreviations: OD, optical density; WT, wild-type; YP, 1 g L−1 yeast extract and 2 g L−1 peptone; YPGlu, YP supplied with 2 g L−1 glucose; YPGal, YP supplied with 2 g L−1 galactose.
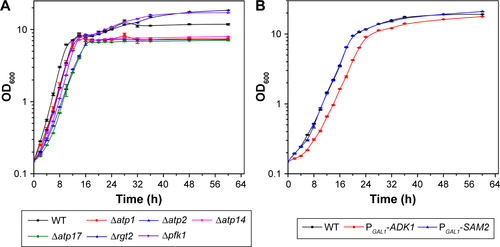
Figure S3 Sugar remaining in the supernatants of different strains after cultivation for 24 hours right before Na2SeO3 was added into the culture.
Abbreviations: A540, absorbance; OD, optical density; WT, wild-type.
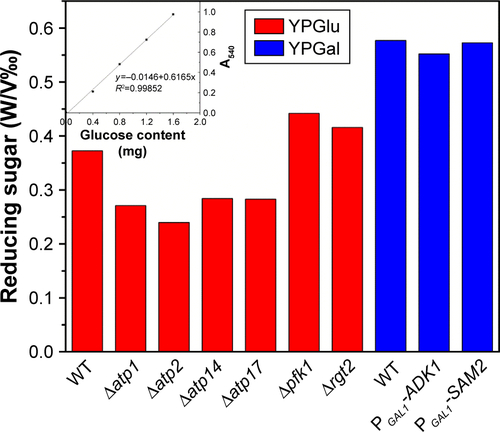
Figure S4 FL intensities (A) and intracellular ATP concentrations (B) of the WT, Δpfk1, and Δrgt2.
Abbreviations: CPS, counts per second; FL, fluorescence; OD, optical density; WT, wild-type.
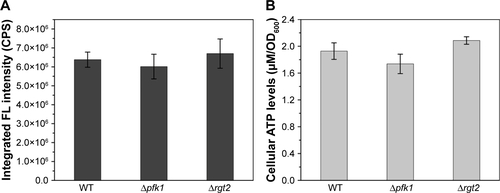
Figure S5 Intracellular ATP concentrations of WT samples and WT cells under the influences of 150 mM NaN3 or 2 mM DNP.
Abbreviations: DNP, 2,4-dinitrophenol; WT, wild-type.
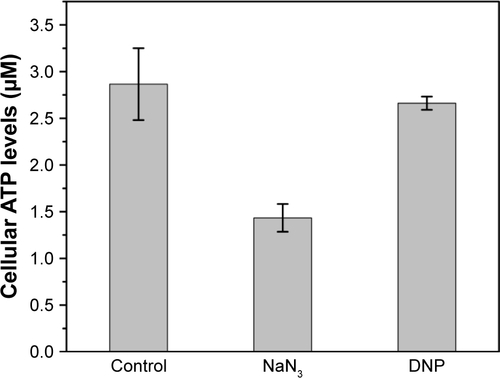
Figure S6 Intracellular ATP concentrations of the WT cells under the effect of 0.2% (wt/vol) 2-dexoglucose (2-DG) added with the introduction of CdCl2. −, blank control without 2-DG; +, 0.2% 2-DG into the culture.
Abbreviations: OD, optical density; WT, wild-type.
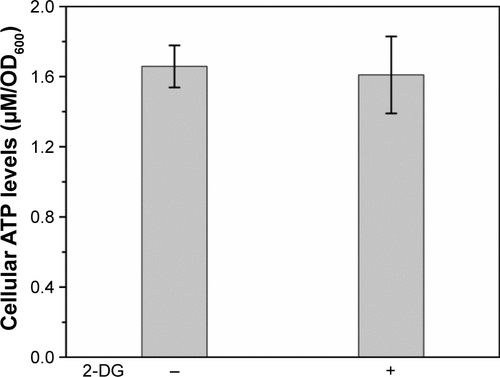
Figure S7 Schematic of the homologous recombination process (A) and diagnostic polymerase chain reaction strategy (B). Gene specific primers CKA and CKB match the upstream and downstream of the insert site on the chromosome; F4 and R2 primers match the inner sequences of the insert DNA.
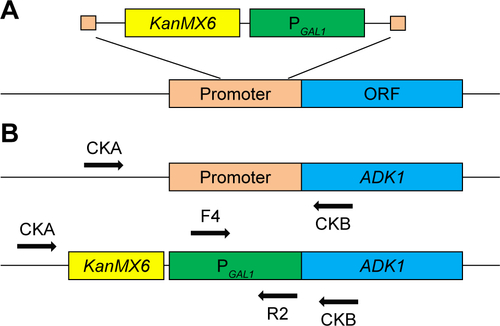
Figure S8 Diagnostic PCR electrophoresis image for confirming the correct replacement of the promoter of the PGAL1-ADK1 strain, the primers used as referred, templates were: 1, transformant genome; 2, WT genome; 3, no template control.
Abbreviation: WT, wild-type.
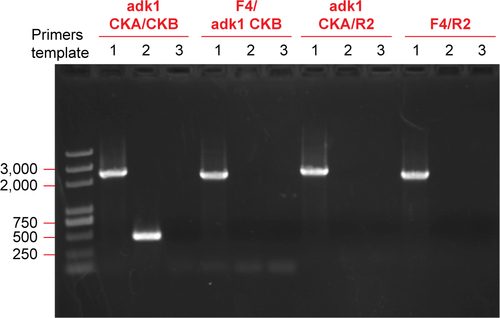
Figure S9 Electrophoresis image of the diagnostic PCR products using the primers referred to in Figure S7; templates were: 1, transformant genome; 2, WT genome; 3, no template control (A), and intracellular SAM concentration of the WT and correct transformant cells (B).
Abbreviations: PCR, polymerase chain reaction; WT, wild-type.
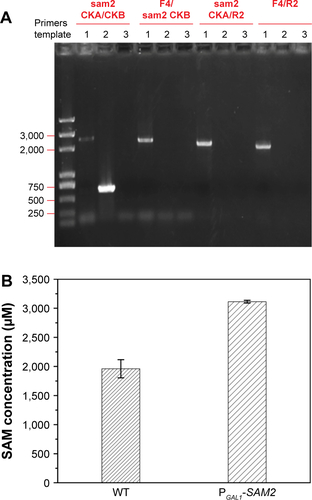
Figure S10 FL intensity of the same WT samples determined three times with a 24 hour interval.
Abbreviations: CPS, counts per second; FL, fluorescence; WT, wild-type.
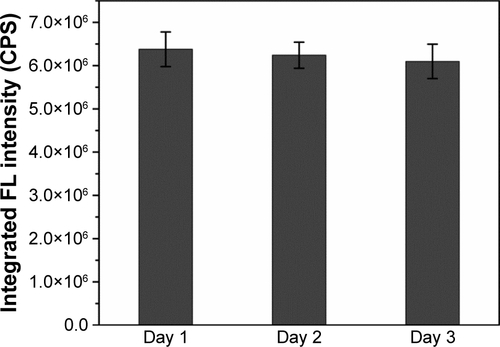
Figure S11 Emission spectrums of the corresponding strains used in the present work cultured in YPGlu (A) or YPGal (B) medium.
Abbreviations: CPS, counts per second; FL, fluorescence; WT, wild-type.
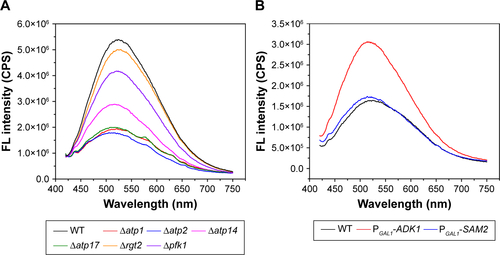
Table S1 List of strains
Table S2 PCR primers used in this study
References
- AlivisatosAPSemiconductor clusters, nanocrystals, and quantum dotsScience19962715251933937
- ShinchiHWakaoMNagataNCadmium-free sugar-chain-immobilized fluorescent nanoparticles containing low-toxicity ZnS-AgInS2 cores for probing lectin and cellsBioconjug Chem201425228629524437371
- GaoXLiuXLinZLiuSSuXCuInS2 quantum dots as a near-infrared fluorescent probe for detecting thrombin in human serumAnalyst2012137235620562423061093
- LiuLWHuRLawWCOptimizing the synthesis of red- and near-infrared CuInS2 and AgInS2 semiconductor nanocrystals for bioimagingAnalyst2013138206144615323967444
- YongKTRoyIHuRSynthesis of ternary CuInS2/ZnS quantum dot bioconjugates and their applications for targeted cancer bioimagingIntegr Biol (Camb)201022–312112920473390
- LiuXJuHCoreactant enhanced anodic electrochemiluminescence of CdTe quantum dots at low potential for sensitive biosensing amplified by enzymatic cycleAnal Chem200880145377538218522432
- LiuXChengLLeiJLiuHJuHFormation of surface traps on quantum dots by bidentate chelation and their application in low-potential electrochemiluminescent biosensingChemistry20101635107641077020799302
- NannTSkinnerWMQuantum dots for electro-optic devicesACS Nano2011575291529521728290
- HuangHHZhaoFCLiuLGEmulsion synthesis of size-tunable CH3NH3PbBr3 quantum dots: an alternative route toward efficient light-emitting diodesACS Appl Mater Interfaces2015751281282813326652661
- PennerRMHybrid electrochemical/chemical synthesis of quantum dotsAcc Chem Res2000332788610673315
- MandalDBolanderMEMukhopadhyayDSarkarGMukherjeePThe use of microorganisms for the formation of metal nanoparticles and their applicationAppl Microbiol Biotechnol200669548549216317546
- RodriguesAGPingLYMarcatoPDBiogenic antimicrobial silver nanoparticles produced by fungiAppl Microbiol Biotechnol201397277578222707055
- DameronCTReeseRNMehraRKBiosynthesis of cadmium-sulfide quantum semiconductor crystallitesNature19893386216596597
- HnainABrooksJLefebvreDDThe synthesis of elemental selenium particles by Synechococcus leopoliensisAppl Microbiol Biotechnol20139724105111051924146080
- ShankarSSAhmadAPasrichaRSastryMBioreduction of chloroaurate ions by geranium leaves and its endophytic fungus yields gold nano-particles of different shapesJ Mater Chem200313718221826
- BhainsaKCD’SouzaSFExtracellular biosynthesis of silver nano-particles using the fungus Aspergillus fumigatusColloids Surf B Biointerfaces200647216016416420977
- ShankarSSRaiAAhmadASastryMRapid synthesis of Au, Ag, and bimetallic Au core-Ag shell nanoparticles using Neem (Azadirachta indica) leaf brothJ Colloid Interface Sci2004275249650215178278
- ChandranSPChaudharyMPasrichaRAhmadASastryMSynthesis of gold nanotriangles and silver nanoparticles using Aloe vera plant extractBiotechnol Prog200622257758316599579
- TanLJWanAJLiHLSynthesis of near-infrared quantum dots in cultured cancer cellsACS Appl Mater Interfaces201461182324344828
- StürzenbaumSRHöcknerMPanneerselvamABiosynthesis of luminescent quantum dots in an earthwormNat Biotechnol2013815760
- XiongLHCuiRZhangZLUniform fluorescent nanobioprobes for pathogen detectionACS Nano2014855116512424779675
- AyanoHKurodaMSodaSIkeMEffects of culture conditions of Pseudomonas aeruginosa strain RB on the synthesis of CdSe nanoparticlesJ Biosci Bioeng2015119444044525454693
- GerickeMPinchesAMicrobial production of gold nanoparticlesGold bulletin20063912228
- JhaAKPrasadKPrasadKA green low-cost biosynthesis of Sb2O3 nanoparticlesBiochem Eng J2009433303306
- VetchinkinaEPLoshchininaEAVodolazovIRKurskyVFDykmanLANikitinaVEBiosynthesis of nanoparticles of metals and metalloids by basidiomycetes. Preparation of gold nanoparticles by using purified fungal phenol oxidasesAppl Microbiol Biotechnol201710131047106227717965
- CuiRLiuHHXieHYLiving yeast cells as a controllable biosynthesizer for fluorescent quantum dotsAdv Funct Mater2009191523592364
- LiYCuiRZhangPMechanism-oriented controllability of intracellular quantum dots formation: the role of glutathione metabolic pathwayACS Nano2013732240224823398777
- XiongLHCuiRZhangZLUniform fluorescent nanobioprobes for pathogen detectionACS Nano2014855116512424779675
- KrugerNJThe Bradford method for protein quantitationMethods Mol Biol199434915
- TakedaMChenWJSaltzgaberJDouglasMGNuclear genes encoding the yeast mitochondrial ATPase complex. Analysis of ATP1 coding the F1-ATPase alpha-subunit and its assemblyJ Biol Chem19862613215126151332876995
- Saltzgaber-MullerJKunapuliSPDouglasMGNuclear genes coding the yeast mitochondrial adenosine triphosphatase complex. Isolation of ATP2 coding the F1-ATPase beta subunitJ Biol Chem19832581911465114706225776
- ArselinGVaillierJGravesPVVeloursJATP synthase of yeast mitochondria. Isolation of the subunit h and disruption of the ATP14 geneJ Biol Chem19962713420284202908702761
- SpannagelCVaillierJArselinGGravesPVVeloursJThe subunit f of mitochondrial yeast ATP synthase. Characterization of the protein and disruption of the structural gene ATP17Eur J Biochem19972473111111179288937
- WeissADelpropostoJGirouxCNHigh-throughput phenotypic profiling of gene-environment interactions by quantitative growth curve analysis in Saccharomyces cerevisiaeAnal Biochem20043271233415033507
- GuYPCuiRZhangZLXieZXPangDWUltrasmall near-infrared Ag2Se quantum dots with tunable fluorescence for in vivo imagingJ Am Chem Soc20121341798222148738
- KiriyamaKHaraKYKondoAOxidized glutathione fermentation using Saccharomyces cerevisiae engineered for glutathione metabolismAppl Microbiol Biotechnol201397167399740423820559
- AdamisPDMannarinoSCEleutherioECGlutathione and gamma-glutamyl transferases are involved in the formation of cadmium-glutathione complexFEBS Lett200958391489149219345220
- KieliszekMBlazejakSGientkaIBzducha-WrobelAAccumulation and metabolism of selenium by yeast cellsAppl Microbiol Biotechnol201599135373538226003453
- McDermottJRRosenBPLiuZJJen1p: a high affinity selenite transporter in yeastMol Biol Cell201021223934394120861301
- GomesDSFragosoLCRigerCJPanekADEleutherioECARegulation of cadmium uptake by Saccharomyces cerevisiaeBBA Gen Subjects2002157312125
- GuoXQHeMNanKYanHChenBBHuBA dual extraction technique combined with HPLC-ICP-MS for speciation of seleno-amino acids in rice and yeast samplesJ Anal At Spectrom2015312406414
- JudahJDThe action of 2:4-dinitrophenol on oxidative phosphorylationBiochem J195149327128514858332
- StannardJNHoreckerBLThe in vitro inhibition of cytochrome oxidase by azide and cyanideFed Proc19476172599608
- LiuLMLiYDuGCChenJIncreasing glycolytic flux in Torulopsis glabrata by redirecting ATP production from oxidative phosphorylation to substrate-level phosphorylationJ Appl Microbiol200610051043105316630005
- ZhouJLiuLChenJImproved ATP supply enhances acid tolerance of Candida glabrata during pyruvic acid productionJ Appl Microbiol20111101445320880146
- SakaiY RTYoneharaTKatoNTaniYHigh–level ATP production by a genetically–engineered Candida YeastNat Biotechnol1994123291293
- LongtineMSMcKenzieAIDemariniDJAdditional modules for versatile and economical PCR-based gene deletion and modification in Saccharomyces cerevisiaeYeast199814109539619717241
- ChenHWangZWangZDouJZhouCImproving methionine and ATP availability by MET6 and SAM2 co-expression combined with sodium citrate feeding enhanced SAM accumulation in Saccharomyces cerevisiaeWorld J Microbiol Biotechnol201632411026596268
- KokSdKozakBUPronkJTvan MarisAJEnergy coupling in Saccharomyces cerevisiae: selected opportunities for metabolic engineeringFEMS Yeast Res201212438739722404754