Abstract
Polymeric micelles (PMs) have been widely investigated as nanocarriers for drug delivery and cancer treatments due to their excellent physicochemical properties, drug loading and release capacities, facile preparation methods, biocompatibility, and tumor targetability. They can be easily engineered with various functional moieties to further improve their performance in terms of bioavailability, circulation time, tumor specificity, and anticancer activity. The stimuli-sensitive PMs capable of responding to various extra- and intracellular biological stimuli (eg, acidic pH, altered redox potential, and upregulated enzyme), as well as external artificial stimuli (eg, magnetic field, light, temperature, and ultrasound), are considered as “smart” nanocarriers for delivery of anticancer drugs and/or imaging agents for various therapeutic and diagnostic applications. In this article, the recent advances in the development of stimuli-responsive PMs for drug delivery, imaging, and cancer therapy are reviewed. The article covers the generalities of stimuli-responsive PMs with a focus on their major delivery strategies and newly emerging technologies/nanomaterials, discusses their drawbacks and limitations, and provides their future perspectives.
Introduction
Cancer remains one of the leading causes of human death worldwide. Although significant progress has been made in cancer biology, genomics, proteomics, and clinical oncology during the past several decades, anticancer treatments are unsatisfactory and the overall survival of many cancer patients stays low.Citation1 Chemotherapy is one of the most commonly used cancer treatment options in the clinic. Typically, chemotherapy drugs are toxic chemicals and administrated systemically via either parenteral or oral routes. However, these drugs often have drawbacks, including poor solubility and bioavailability, unfavorable pharmacokinetics, and nonselective biodistribution, which may complicate their clinical use and lead to undesirable side effects.Citation2,Citation3
The nanotechnology-based medicines, that is, nanomedicines, have shown excellence in drug delivery, diagnosis, and therapy, particularly in cancer research. Nanoscale drug delivery systems (NDDSs) or nanocarriers play an important role in nanomedicine to overcome the aforementioned drawbacks of chemotherapy drugs. Compared to traditional drug delivery systems, nanocarriers have shown greater potential in improving drug bioavailability, prolonging drug circulation time, controlling drug release, and targeting tumor. In addition to performing these basic functions, the newly emerging technology and nanomaterials may offer extra functions or opportunities to nanomedicines/nanocarriers for various purposes of cancer diagnosis and treatments.Citation4,Citation5 Various nanocarriers have been developed, of which the most commonly studied are the organic nanocarriers, such as liposomes, polymeric micelles (PMs), and dendrimers, and inorganic nanocarriers, such as carbon nanotubes, silica nanoparticles, gold nanoparticles, magnetic nanoparticles, and quantum dots.Citation6,Citation7
PMs are one of the first polymeric self-assemblies. Over the past few decades, they have received tremendous attention in biomedical sciences, especially as drug carriers, since they provide several physicochemical and biological advantages over other types of nanoassemblies, including easy preparation, simple architecture, drug solubilization, improved biocompatibility, pharmacokinetics, and biodistribution, and additional opportunities for further engineering.Citation8,Citation9 It is worth noting that several drug-loaded PM formulations have been approved for clinical trials for cancer treatments, and some PMs showed the promising anticancer activity ().Citation10,Citation11 For instance, Genexol®-PM is a paclitaxel (PTX)-loaded PM formulation which consists of a block copolymer, poly(ethylene glycol) (PEG)-poly(d,l-lactide) (PLA). PTX is one of the most commonly used chemotherapy drugs for treating ovarian, breast, lung, cervical, and pancreatic cancers. However, it has very low water solubility and is currently used as a solution in Cremophor EL/ethanol (1:1, w/w). Unfortunately, the Cremophor may cause unwanted side effects such as hypersensitivity, nephrotoxicity, and neu-rotoxicity. Moreover, the dose-limiting toxicity such as neuropathy and neutropenia is another concern. In preclinical studies, Genexol-PM demonstrated a threefold increase in the maximum tolerated dose and a significantly increased antitumor efficacy compared with free PTX. Currently, a number of clinical studies over Genexol-PM are underway.
Table 1 Current clinical trials of anticancer drug-loaded polymer micelles
In order to exert anticancer effects, drugs have to be released from the PMs efficiently and specifically in tumor tissues or inside tumor cells. However, highly stable PMs may result in slow and incomplete drug release and lower the efficacy of the loaded drugs. Therefore, various stimuli-responsive PMs have been developed for “smartly” controlling drug release as well as drug efficacy.Citation12,Citation13 The idea of stimuli-responsive drug delivery systems comes from the fact that the tumor tissue possesses a variety of unique features compared with the normal tissue, including acidic pH, altered redox potential, and overexpressed proteins and enzymes. In addition, stimuli such as temperature, light, ultrasound, and magnetic fields can be applied to the tumor site externally to allow PMs to bypass the biological barriers, reach their targets, and release their loaded drugs. This article aims to summarize and discuss the recent advances of stimuli-responsive PMs in drug delivery and cancer therapy.
Polymeric micelles
PMs are spherical colloidal particles with a size typically ranging from 10 to 100 nm. They are composed of amphiphilic copolymers that have distinct hydrophobic and hydrophilic blocks.Citation14 Usually, these copolymers are amphiphilic di-block (hydrophilic–hydrophobic) or tri-block (hydrophilic–hydrophobic–hydrophilic) polymers. The most commonly used hydrophilic blocks are PEG with a molecular weight of 2–15 kDa, while the hydrophobic blocks typically are polyesters, polyethers, or polyamino acids, such as poly(l-aspartic acid), PLA, poly(ε-caprolactone) (PCL), and poly(propylene oxide) (PPO).Citation15 The defining characteristic of PMs is the polymers’ ability of spontaneous self-assembly into nanoscale aggregates. In aqueous solutions, the hydrophobic blocks self-associate into a semisolid core surrounded by the hydrophilic segments as a corona/shell. The hydrophilic shell provides steric stability and minimizes nonspecific uptake by the reticuloendothelial system (RES), resulting in the prolonged circulation time in the body.Citation16,Citation17 Given the fact that many anticancer drugs are water insoluble, the hydrophobic core of PMs provides a perfect place for drug loading. By this way, the insoluble drugs can be “solubilized”. The critical micelle concentration (CMC) is the minimum concentration of copolymers required for micelle formation. At the concentration lower than CMC, the copolymers exist as monomers throughout the solution, while at the concentration around or above CMC, the polymer chains associate and the self-assemblies/micelles are formed. Most reported PMs showed quite low CMC in comparison with low-molecular-weight surfactant micelles.Citation17
In the early 1980s, Gros et al first reported PMs as drug delivery vehicles for cancer treatment.Citation18 Since then, a great number of PMs have been developed to deliver various anticancer drugs and other bioactive molecules for cancer diagnosis and therapy. As drug carriers, the core–shell-structured PMs offer several advantages and unique properties for cancer-targeted drug delivery. First of all, as mentioned previously, PMs can load the water-insoluble drugs by physical entrapment or chemical conjugation, resulting in greatly increased drug solubility. As an example, 7-ethyl-10-hydroxy-camptothecin (SN-38), a highly effective anticancer agent, which acts as a DNA topoisomerase I inhibitor, cannot be administered intravenously due to its poor water solubility and high toxicity. By conjugation of the drug to PEG-poly(glutamic acid) (NK012), the solubility of the drug is significantly increased. As a result, the IC50 value of NK012 is deceased up to 5.8 times compared to that of free SN-38.Citation19 In solid tumors, the tumor specificity of the nanodrug carriers considerably relies on the enhanced permeability and retention (EPR) effect, a passive targeting strategy.Citation20,Citation21 For passive targeting, nanoparticles have to circulate in the blood for a long period of time to ensure that sufficient drugs are delivered to the tumor tissue, in which their size plays a very important role. Nanoparticles smaller than 10 nm are easily eliminated through the renal glomeruli, whereas nanoparticles larger than 100 nm can be cleared from the bloodstream rapidly by the liver and spleen.Citation22–Citation24 On the basis of this knowledge, PMs with a suitable size (10–100 nm) can avoid their quick clearance from the body, thus allowing for their accumulation in the tumor tissues via the EPR effect. In addition, the biocom-patible polymeric corona of PMs can suppress nonspecific interactions with biological components and lead to reduced recognition by the RES, prolonging the circulation time in the bloodstream. Nevertheless, the EPR effect of PMs may not always benefit the treatments particularly in the human clinical trials due to the heterogeneity and complexity of the tumor environment. Thus, PMs can be further modified with ligands for active targeting to increase their tumor selectivity and intracellular drug delivery but reduce their systemic toxicity and other adverse effects.Citation25 Compared to nontargeted PMs, a number of studies have showed the superior results of the active-targeting PMs, such as improved cellular uptake, cytotoxicity, and tumor regression, making the active targeting strategy an important addition to the passive targeting PMs. All these advantages demonstrate PMs may be a promising and powerful drug platform for cancer therapeutic applications.
Stimuli-responsive PMs
Despite the successful applications of PMs as anticancer drug carriers, some problems remain. For example, PMs are highly associated with premature drug release in the circulation and inadequate drug release upon accumulation in the targeted tissue.Citation26 Ideally, the drug is retained in the PMs during circulation but is released after accumulation in the tumor interstitium. The premature drug release can be prevented by appropriate micelle stabilization or enhancement of drug interaction in the micelle core via specific bonding strengths like hydrogen bonds or cleavable covalent bonds. As to achieving tumor-targeted drug delivery and adequate release, a very effective way is the design of stimuli-responsive PMs. With the increasing knowledge of tumor biology, we know that tumor tissues possess a low pH in solid tumors with an even lower pH inside tumor cells, overexpressed specific enzymes, high levels of glutathione (GSH) in the cytoplasm, as well as a higher temperature compared with the normal tissues. These unique features can be utilized as internal triggers along with external stimuli such as magnetic field, ultrasound, and light to permit the destabilization of stimuli-responsive PMs and achieve temporally and spatially controlled drug delivery and release.Citation13 The strategies for the development of stimuli-responsive PMs used in cancer therapy are reviewed individually below (typical stimuli-responsive PMs are summarized in ).
Table 2 Examples of stimuli-responsive polymeric micelles for cancer therapy
pH-responsive PMs
The pH-responsive PMs have recently emerged as important nanocarriers for anticancer drug/imaging agent delivery.Citation27–Citation30 As a result of enhanced metabolic rates and increased aerobic glycolysis, the microenvironment of most solid tumors is intrinsically acidic (pH 6.5–7.2), while the pH value in the blood and normal tissue is about 7.4. Moreover, an even lower acidic pH is found in endosomes (pH 6.5–5.5) and lysosomes (pH 4.5–5.0).Citation31 Accordingly, the difference in pH has been widely exploited to achieve tumor site- or organelle-specific activation of pH-responsive PMs and on-demand drug release. The pH sensitivity of polymers mainly results from the protonation of ionizable groups or the degradation of pH-sensitive linkages. Therefore, one possible approach to impart pH sensitivity to PMs is to incorporate the ionizable groups into the micellar polymers to ionize at the tumor extracellular or intracellular acidic pH. After the micelle decomposition or destabilization, their payloads are released at the tumor tissues. For example, a pH-sensitive mixed micelle was prepared by Wu et al for cytosolic delivery of doxorubi-cin (DOX), which was composed of two block polymers, 1,2-distearoyl-sn-glycero-3-phosphorylethanolamine-PEG and pH-responsive poly(histidine) (PHis)-PEG.Citation32 PHis is a commonly employed pH-sensitive polymer as the imidazole ring has an electron lone pair on the unsaturated nitrogen that endows PHis with an amphoteric nature by protonation–deprotonation. Such systems could not only stabilize the micelles at neutral and tumor extracellular pH (pHe) but also facilitate micelle’s disintegration and then result in quick drug release in response to the low endocytic pH. Similarly, Hu et al utilized polysulfadimethoxine (PSDM) and PHis to construct a micellar system to realize sequential pH responsiveness at the tumor extracellular and endosomal pH.Citation33 Cationic micelles were prepared from a block copolymer of PHis-poly(ethylene imine) (PEI), which was shielded by the negatively charged methoxy PEG (mPEG)-PSDM at pH 7.4 and deshielded at the tumor extracellular pH due to the pH-responsive sulfonamide group of PSDM. After endocytosis, PHis was protonated in the endosomal compartment, leading to dissociation of the micelle and destabilization of the endosomal membrane to promote the delivery of drugs to the cytosol. The in vivo results demonstrated that the PMs showed significant anticancer efficacy and deep penetration into tumor tissues.
Another common pH-sensitive strategy utilizes the degradation of pH-sensitive linkages at compartments with lower pH. In recent years, one major branch of our research focused on stimuli-responsive nanodrug delivery systems, and our group has made several attempts to construct pH-sensitive PMs for cancer therapy.Citation32,Citation34,Citation35 In a study, a novel delivery system based on luteinizing hormone-releasing hormone (LHRH)-PEG-PHis-DOX/DOX-trans-activating transcriptional activator (TAT) acid-sensitive micelle was developed, as shown in , which could dissociate and release DOX-TAT when responding to the tumor extracellular pH.Citation34 Such systems could efficiently go across the cell membrane and reach the cytosol of the multidrug-resistant cancer cells, resulting in the remarkable antitumor efficacy and negligible systematic toxicity. Zhou et al developed an N-(2-hydroxypropyl)methacrylamide (HPMA) copolymer-based micellar drug delivery system, in which the hydrophobic drug DOX and β-sitosterol were conjugated to the hydrophilic HPMA polymer backbone via a pH-sensitive hydrazone linker.Citation36 The pH-responsive linker was stable at physiological pH but could be easily hydrolyzed at acidic pH. In the in vitro studies, the PMs could quickly collapse with 80% of the drug released at pH 5, the lyso/endosomal pH. In the in vivo studies, the PMs showed significantly higher anticancer effect.
Figure 1 (A) Delivery strategy of LHRH-PEG-PHis-DOX/DOX-TAT micelles. (B) Images of the tumors on day 14 after the inoculation of A2780/DOXR tumor cells in the mice and in vivo antitumor efficacy.
Notes: Reprinted from Yang T, Li F, Zhang H, et al. Multifunctional pH-sensitive micelles for tumor-specific uptake and cellular delivery. Polym Chem. 2015;6(8):1373–1382.Citation34 With permission from the Royal Society of Chemistry. Copyright 2014 Royal Society of Chemistry.
Abbreviations: LHRH, luteinizing hormone-releasing hormone; PEG, poly(ethylene glycol); PHis, poly(histidine); DOX, doxorubicin; TAT, trans-activating transcriptional activator.
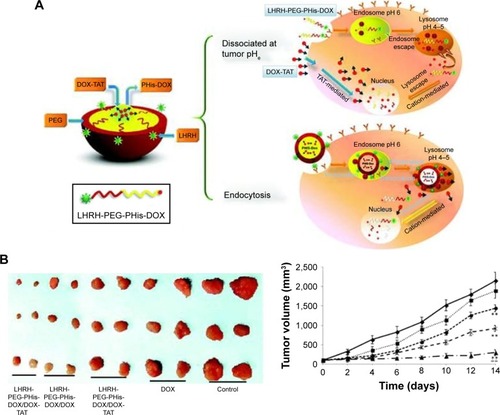
As we know, the positively charged carriers could effectively interact with the negatively charged cell membrane by electrostatic attraction and trigger efficient cell internalization.Citation37,Citation38 However, for in vivo drug delivery, positively charged surface is not favorable due to nonspecific interactions and resultant short circulation half-life.Citation39,Citation40 Thus, strategies that make use of charge conversion or charge reversal are highly required and always in pursue for pH-sensitive drug delivery, in which PMs are usually neutral or negatively charged at physiological pH and are capable of converting their surface charge into positive one in response to the tumor extracellular pH. In our previous work, a dual pH-sensitive charge-reversing poly(β-l-malic acid) (PMLA)-based micellar nanocomplex (PMLA-PEI-DOX-TAT@PEG-2,3-dimethylmaleic anhydride (DMMA)) was developed for effective tumor-targeted drug delivery, enhanced cellular uptake, and intracellular drug release, which is illustrated in .Citation35 The prepared micellar nanocomplex showed a negative surface charge at the physiological pH, while at the tumor extracellular environment, charge reversal of PEG-DMMA took place and the positively charged and TAT-exposed PMs were released, resulting in enhanced cellular internalization. Moreover, after endocytosis, the PMs showed pH-sensitive quick drug release at the endo/lysosomal pH because of the pH-sensitive linker.
Figure 2 (A) Preparation of nanocomplex PMLA-PEI-DOX-TAT@PEG-DMMA. (B) Schematic illustration of the dual pH-sensitive DOX-loading nanocomplex with the charge-conversional function for effective tumor-targeted drug delivery, enhanced cellular uptake, and intracellular drug release.
Notes: Reprinted from Zhou Q, Hou Y, Zhang L, et al. Dual-pH sensitive charge-reversal nanocomplex for tumor-targeted drug delivery with enhanced anticancer activity. Theranostics. 2017;7(7):1806–1819.Citation35 Creative Commons Attribution (CC BY-NC) license, available from: https://creativecommons.org/licenses/by-nc/4.0/
Abbreviations: PMLA, poly(β-l-malic acid); PEI, poly(ethylene imine); DOX, doxorubicin; TAT, trans-activating transcriptional activator; PEG, poly(ethylene glycol); DMMA, 2,3-dimethylmaleic anhydride.
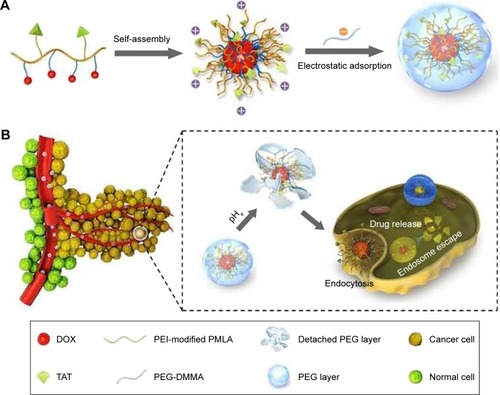
Redox-responsive PMs
Redox potential has been regarded as a viable biomarker to distinguish between the extracellular and intracellular environments, as well as between the tumor and normal tissues due to the difference in the GSH concentration. The concentration of GSH in cytoplasm (approximately 2–10 mM) could be 100–1,000 times higher than that in the extracellular environments (approximately 2–10 μM).Citation41–Citation43 In comparison with healthy tissues, some tumor tissues are found to be highly reducing and hypoxic, with the intracellular GSH concentration being at least four times higher than that of the normal cells.Citation41,Citation43 In the past decade, a tremendous progress was made in the development of redox-responsive nanovehicles for targeted intracellular drug or gene delivery as the redox response is exceedingly fast and efficient in the presence of high concentration of GSH in the tumor or intracellular environments. In addition, unlike pH-sensitive nanocarriers that are usually designed to release drugs in the lyso/endosomal compartments, redox-responsive systems can disassemble and release drugs in the cytosol and cell nucleus. Disulfide bonds, prone to rapid cleavage by GSH, are commonly used to gain redox sensitivity. The chemical structure of disulfide-containing cross-linking agents is shown in . Until now, a number of redox-responsive PMs have been designed by incorporating a disulfide bond either in the hydrophobic blocks or between the hydrophobic and hydrophilic segments.Citation44–Citation46 Under the redox environment, these PMs can be reductively degradable or rapidly disassembled to release payloads.
Figure 3 Chemical structures of disulfide-containing redox-sensitive linkers.
Abbreviations: SAND, sulfosuccinimidyl 2-(m-azido-o-nitrobenzamido)-ethyl-1,3′-dithio propionate; SAED, sulfosuccinimidyl-2-(7-amino-4-methylcoumarin-3-acetamido)-ethyl-1,3-dithiopropionate; LC-SPDP, succinimidyl 6-(3(2-pyridyldithio)propionamido)hexanoate; LC-SMPT, 4-succinimidyloxycarbonyl-α-methyl-α(2-pyridyldithio)toluene; SFAD, sulfosuccinimidyl-(perfluoroazidobenzamido)-ethyl-1,3′-dithiopropionate.
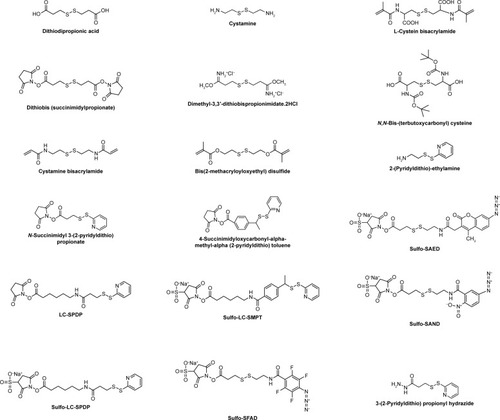
Shi et al used four-arm PCL-PEG copolymers to develop a multifunctional star-shaped micellar system by combination of active targeting ability and redox-responsive behavior.Citation47 The redox-responsive behavior was realized by connecting PCL and PEG via disulfide bonds, and active targeting ability was achieved by the introduction of folate (FA) ligands to the end groups of the hydrophilic segment, as shown in . DOX was trapped into the micelles during the self-assembly of the star-shaped PCL-PEG copolymers. The prepared redox-responsive PMs could be specifically internalized by tumor cells through the FA receptor-mediated endocytosis, and the disulfide bonds could be immediately cleaved in response to the intracellular high level of GSH, resulting in quick DOX release. The same group reported another redox-responsive polyanhydride-based micelle for cancer therapy.Citation48 The amphiphilic polyanhydride copolymer contained disulfide bonds between the hydrophilic and hydrophobic segments. Redox sensitivity of the micelles was demonstrated by the changes of the micellar size, morphology, and molecular weight. Particularly, an approximate zero-order in vitro drug (curcumin) release mode in a short time was observed in a reducing and acidic environment which was similar to that of the tumor cell, leading to significant in vitro and in vivo antitumor activities.
Figure 4 Schematic illustration of DOX-loaded star-shaped micelles based on FA-functionalized and redox-responsive star copolymer (star-PCL-ss-PEG-FA) for FR-mediated endocytosis and GSH-triggered intracellular drug release.
Notes: Reprinted from Shi C, Guo X, Qu Q, Tang Z, Wang Y, Zhou S. Actively targeted delivery of anticancer drug to tumor cells by redox-responsive star-shaped micelles. Biomaterials. 2014;35(30):8711–8722.Citation47 With permission from Elsevier. Copyright 2014 Elsevier.
Abbreviations: DOX, doxorubicin; FA, folate; PCL, poly(ε-caprolactone); FR, folate receptor; GSH, glutathione; PEG, poly(ethylene glycol).
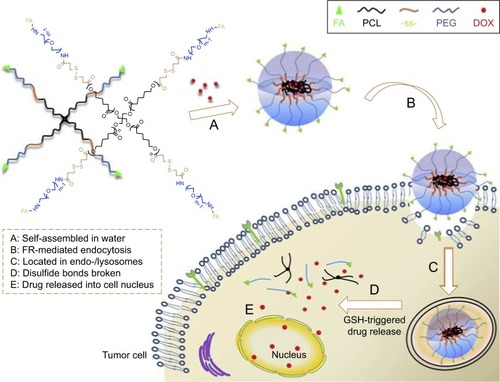
Enzyme-responsive PMs
Enzymes play critical roles in many biological and metabolic processes inside the body due to their exceptional specificity and outstanding catalytic properties.Citation49 The dysregulation of enzyme expression and activity has been observed in a variety of different pathological situations and is associated with many diseases. For example, the expression of several types of enzymes like proteases, peptidases, and lipases in solid tumors is often greater compared to their concentrations in normal tissues since high concentrations of these enzymes play a very important role in tumor cell growth, angiogenesis, invasion, and metastasis.Citation50–Citation52 Dysregulated enzymes have been considered as biomarkers for diagnostics and prognostics in different types and stages of cancer, which are also emerging as promising biological triggers for targeted cancer therapy. Using enzymes as a trigger could provide a number of benefits as most enzymes catalyze chemical reactions under mild conditions (eg, low temperature and neutral pH value), however, many conventional nonenzymatic chemical reactions may not happen under such conditions. Moreover, enzymes possess highly exceptional selectivity for their substrates, thus allowing for specific, complicated, biologically inspired chemical reactions. Based on these principles, in the past few years, a wide variety of NDDSs have been developed as the enzyme-responsive systems for selective and efficient targeted drug delivery, including polymeric nanoparticles, liposomes, and inorganic nanoparticles.Citation49,Citation53–Citation56 The enzyme responsiveness can be rendered to the PMs for anticancer drug delivery by incorporating specific moieties in their main chain or side groups that can be selectively recognized and degraded by overexpressed enzymes in the extra/intracellular environment of the tumor, enabling tumor-targeted and spatiotemporal drug release with reduced unwanted side effects in normal tissues.
Matrix metalloproteinases (MMPs), a kind of extracellular matrix (ECM)-remodeling proteases, have been found highly associated with tumor invasion and metastasis.Citation50,Citation51 They are a family of zinc- and calcium-dependent endopeptidases that are responsible for the degradation and remodeling of the ECM and are relatively overexpressed in almost all tumors compared with normal tissues. Thus, MMPs can be employed to achieve enzyme-responsive site-specific drug release. Among them, MMP2 and MMP9 are the most widely explored enzymes for enzymatic drug delivery. For example, a unique tumor-targeted micellar drug delivery platform was reported by Zhu et al, which was prepared by self-assembly of the block copolymers of MMP2-sensitive PEG2000-PTX conjugate, transactivating transcriptional activator peptide-PEG1000-phosphoethanolamine (PE), and PEG1000-PE, acting as MMP2-sensitive functional polymer, cell-penetrating enhancer, and nanocarrier building block, respectively ().Citation57 The MMP2 sensitivity of PEG2000-peptide-PTX as well as the whole micelle formation was well character-ized. Compared to nonsensitive counterparts, like free PTX and conventional micelles, both in vitro and in vivo studies indicated that this novel design showed superior cell internalization, cytotoxicity, tumor targeting, and antitumor efficacy, which had great potential for effective intracellular delivery of drug into cancer cells. More recently, they designed another MMP2-sensitive multifunctional PM for tumor-specific co-delivery of siRNA and hydrophobic drugs.Citation58 This micellar platform was constructed by an MMP2-sensitive copolymer (PEG-pp-PEI-PE) via self-assembly, which exhibited excellent stability, efficient siRNA condensation by PEI, PTX solubilization in the lipid core, and tumor targeting via both the EPR effect and MMP2 sensitivity.
Figure 5 (A) Drug delivery strategy. (B) Enzymatic cleavage. To determine the digestion of PEG2000-peptide-PTX (left) and its nanopreparation (right), the samples were treated with 5 ng/μL MMP2 followed by TLC and Dragendorff’s reagent staining. (C) Cellular uptake in A549 cell monolayer. Cells were treated with NBD-PE-labeled formulations (green) for 2 hours before measurement. (a) PEG1000-PE, (b) TATp-PEG1000-PE, (c and d) TATp-PEG1000-PE/PEG2000-peptide-PTX. For FACS (left), cells were trypsinized and collected. For confocal microscopy (right), cells were fixed and stained with Hoechst 33342. (D) Cytotoxicity of the nanopreparations in A549 cell monolayer. Cells were treated with moderate-to-low doses of PTX formulations for 72 hours before CellTiter-Blue Cell Viability Assay. (E) Tumor growth inhibition (% of the starting tumor volume). (a) HBSS, (b) TATp-PEG1000-PE/PEG2000-peptide-PTX uncleavable, (c) PEG2000-PE/PTX, (d) Free PTX, (e) TATp-PEG1000-PE/PEG2000-peptide-PTX. *P<0.05 compared with other groups.
Notes: Reprinted from Zhu L, Wang T, Perche F, Taigind A, Torchilin VP. Enhanced anticancer activity of nanopreparation containing an MMP2-sensitive PEG-drug conjugate and cell-penetrating moiety. Proc Natl Acad Sci USA. 2013;110(42):17047–17052.Citation57 With permission from the National Academy of Sciences. Copyright 2013 National Academy of Sciences.
Abbreviations: PEG, poly(ethylene glycol); PTX, paclitaxel; MMP2, matrix metalloproteinase 2; TLC, thin-layer chromatography; NBD, Nitro-2-1,3-BenzoxaDiazol-4-yl; PE, phosphoethanolamine; FACS, fluorescence-activated cell sorting; TATp, transactivating transcriptional activator peptide; GPLG, Gly-Pro-Leu-Gly; IAGQ, Ile-Ala-Gly-Gln.
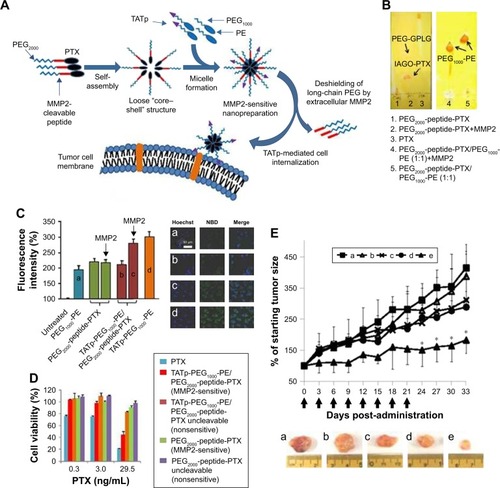
Thermo-responsive PMs
Temperature, which acts as either an internal or an external stimulus, is one of the most often investigated stimuli for drug delivery. Alteration of the temperature in specific tissues can either occur due to certain diseases (eg, tumor, inflammation, or infection) or be achieved by the application of an external heating source/device.Citation59,Citation60 In addition, the elevated temperature, or “hyperthermia”, can also inflict additional damage to tumor cells, such as inhibition of DNA synthesis and repair, disruption of microtubules, and alteration of the receptor expression. Therefore, temperature/thermo-responsive drug delivery systems are among the most investigated stimuli-responsive strategies for cancer therapy.Citation61–Citation64 The thermo-sensitive PMs comprise polymers with thermo-responsive blocks which undergo a sharp change of their physical properties in response to the change of temperature to destabilize the micelles and trigger drug release. Thermo-responsive polymers tend to undergo a phase transition at a certain temperature, known as lower critical solution temperature (LCST).Citation65–Citation67 Below the LCST, the polymers are soluble in water by forming hydrogen bonds between water and the polymer chains, but become insoluble upon heating (above the LCST) due to disruption of hydrogen bonds. Moreover, the polymers can be modulated by introduction of hydrophobic or hydrophilic comonomers to obtain LCST values within a desired range (37°C–42°C), higher than normal body temperature but lower than the hyperthermia of tumors.
The most frequently used thermo-responsive polymer for the preparation of thermo-sensitive PMs is poly(N-isopropylacrylamide) (PNIPAAm) with an LCST of around 32°C, which is close to the physiological temperature of the human body.Citation68–Citation71 The LCST of PNIPAAm can be optimized by random copolymerization with other monomers in order to get improved targeting and drug release. For example, Panja et al developed a smart thermo-responsive micelle from novel four-arm star-shaped block copolymers, namely PE-PCL-b-PNIPAAm-FA and PE-PCL-b-poly(N-vinylcaprolactam)-FA ().Citation72 A series of temperature-responsive polymers with specific LCST (ranging from 30°C to 39°C) were synthesized by tailoring the block length of the thermo-responsive segments. A high rate of DOX release (78.57% after 24 hours) at a temperature above LCST can be achieved due to the temperature-induced size reduction (57%) of the PMs. Moreover, the DOX release rate can also be tuned by on-demand administration of temperature. The in vivo study showed a drastic inhibition of the tumor volume by ∼83.9% without any significant systemic toxicity after administration of the prepared DOX-loaded PMs into the C6 glioma rat model. Similarly, Ahn et al used amphiphilic alginate-g-PNIPAAm to prepare a DOX-loaded and near-infrared (NIR) fluorescent dye-tagged PM for cancer imaging and therapy.Citation73 Amino-PNIPAAm was conjugated to the alginate backbone by carbodiimide chemistry, the obtained alginate-g-PNIPAAm was dissolved in distilled water at room temperature, and self-assembled PMs were formed at 37°C. The in vitro result showed a sustained release of DOX from the PMs at 37°C. The resultant DOX-loaded alginate-g-PNIPAAm PMs exhibited excellent anticancer efficacy in a mouse model without any significant side effects, indicating high potential for the application of cancer imaging and therapy.
Figure 6 (A) Schematic presentation of temperature-induced shrinkage of micelles followed by drug release. (B) Fluorescence microscopic image of DOX-loaded FA-PM-treated C6 glioma cell line over a time span of 1–4 hours. The nuclei were stained with DAPI. (C) Tumor volume of the rat. (D) Variation of tumor volume with time. (E) Microscopic image of in vivo accumulation of control, free DOX, DOX-loaded PMs, and DOX-loaded FA-PMs into the tumor tissue.
Notes: Fluorescence microscopies: red for DOX; blue for DAPI. Reprinted with permission from Panja S, Dey G, Bharti R, et al. Tailor-made temperature-sensitive micelle for targeted and on-demand release of anticancer drugs. ACS Appl Mater Interfaces. 2016;8(19):12063–12074.Citation72 Copyright 2016 American Chemical Society.
Abbreviations: DOX, doxorubicin; FA, folate; PM, polymeric micelle; DAPI, 4′,6-diamidino-2-phenylindole; LCST, lower critical solution temperature.
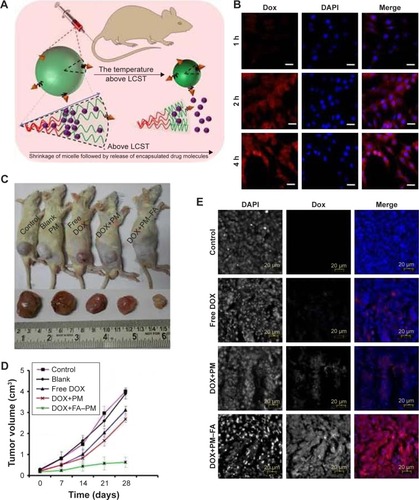
Magnetic field-responsive PMs
Magnetic field-responsive PMs, typically incorporating the therapeutic payloads along with a magnetically active component, could be used not only for tumor targeting under an external magnetic field but also for quick drug release induced by the increased temperature caused by the alternating magnetic field, displaying great potential for cancer treatment.Citation74,Citation75 In addition, owning to its deep penetration, magnetic field represents a promising trigger for the in vivo application. Iron oxide nanoparticles with a size less than 10 nm, like magnetite (Fe3O4) and maghemite (Fe2O3), are most commonly used to impart the magnetic sensitivity.Citation76–Citation78 They are also referred to as superparamagnetic iron oxide nanoparticles (SPIONs) due to their property of superparamagnetism and small sizes. They can be attracted by a magnetic field, but do not retain the residual magnetism upon removal of the field. Apart from magnetic drug targeting, SPIONs have also been widely used for other biomedical applications including magnetic resonance imaging (MRI), magnetic transfection, and magnetic hyperthermia.Citation79 The magnetic hyperthermia is a good example. Under alternating magnetic field, SPIONs would lead to the increased local temperature, which could be utilized to either directly kill tumors or make them more susceptible in combination with chemotherapy. Therefore, magnetic-sensitive polymeric nanoparticles have been explored for remotely controlled drug release as well as a combination of cancer therapy and diagnosis.
Targeted delivery of therapeutics and diagnostics using nanotechnology holds great promise to minimize the side effects of conventional chemotherapy and enable specific and real-time detection of diseases. In this regard, Wei et al recently reported the development of a clickable and imageable nanovehicle assembled from multiblock polyurethanes for precise tumor theranosis.Citation80 The soft segments of the polymers were based on detachable PEG and degradable PCL, and the hard segments were constructed from lysine and cystine derivatives bearing reduction-responsive disulfide linkages and click-active alkynyl moieties, allowing for post-conjugation of targeting ligands via a click chemistry (). They found that the cleavage of PEG corona bearing a pH-sensitive benzoic-imine linkage could act as an on–off switch, which was capable of activating the clicked targeting ligands under extracellular acidic environment, followed by triggering the core degradation and payload release inside tumor cells. Furthermore, in combination with SPION entrapped in the micellar core, the prepared PMs exhibited excellent MRI contrast effects and T2 relaxation in vitro, as well as magnetically guided MRI and multimodal targeting of therapeutics to tumor precisely, resulting in significant inhibition of cancer with minimal side effect.
Figure 7 Design of clickable and imageable multiblock polymer micelles. (A) Schematic chemical structure and (B) molecular architecture of clickable MPUs. The waved lines in (A) represent PCL soft segments. (C) Self-assembly of MPU micelles and post-conjugation of FA via click chemistry. (D) Schematic illustration of FA residues on the interface of polymer micelles. (E) Illustration of magnetic-guided and PEG-switched targeting and release properties of MPU nanocarriers.
Note: Reprinted from Wei J, Shuai X, Wang R, et al. Clickable and imageable multiblock polymer micelles with magnetically guided and PEG-switched targeting and release property for precise tumor theranosis. Biomaterials. 2017;145:138–153.Citation80 With permission from Elsevier. Copyright 2017 Elsevier.
Abbreviations: 1MPU, multiblock polyurethane; PCL, poly(ε-caprolactone); FA, folate; PEG, poly(ethylene glycol); BPEG, benzoic-imine linkage bearing PEG; Cys-PA, L-cystine-propargylamine; AzFA, azido modified FA; SSPHPU, BPEG-(PCL- Cys-PA)-BPEG; SPIONs, superparamagnetic iron oxide nanoparticles; GSH, glutathione.
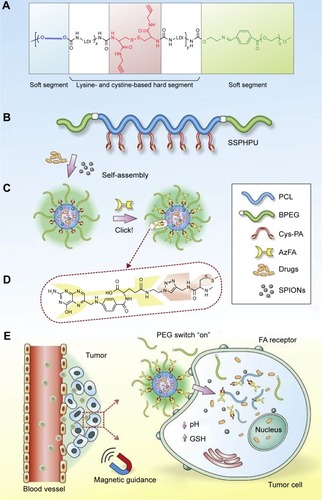
In a similar study, FA-bovine serum albumin (BSA)-functionalized SPION-loaded PMs were prepared by Li et al for tumor targeting and MRI.Citation81 The SPION-loaded PMs were prepared by self-assembly of amphiphilic poly(2,2,3,4,4,4-hexafluorobutyl methacrylate-co-methacryloxyethyl trimethyl ammonium chloride)-g-methoxy PEG monomethacrylate (poly(HFMA-co-MOTAC)-g-PEGMA) copolymers and oleic acid-modified Fe3O4 nanoparticles, and then functionalized with FA-BSA by electrostatic interaction. In vivo MRI conducted on the nude mice bearing the Bel-7402 xenografts after bolus intravenous administration showed excellent tumor targeting and MRI capabilities, especially at 24 hours post-injection. A multifunctional theranostic micellar drug delivery system utilizing cationic poly(2-(dimethylamino)ethyl methacrylate) (PDMA)-b-PCL micelles was developed for the delivery of various agents, including SN-38, ultrasmall SPIONs, and siRNA that targets human vascular endothelial growth factor (VEGF).Citation82 The prepared PMs could passively target tumor regions and synergistically facilitate VEGF silencing and chemotherapy, as well as acted as a negative MRI contrast agent in T2-weighted imaging, indicating a powerful tool for the cancer diagnosis and tracking of the therapeutic outcomes.
Ultrasound-responsive PMs
Due to the ease of administration, low cost, and deep penetration, ultrasound has been widely used in diagnostic and therapeutic applications for many years, and has been proven to be an efficient noninvasive approach for the diagnosis and treatment of a wide range of health problems such as osteoporosis and cardiovascular diseases.Citation83 Additionally, ultrasound has been extensively explored as a trigger for spatiotemporally controlled release of active agents encapsulated in nanopreparations at the desired site, thus reducing unwanted side effects. In the past years, a number of NDDSs with ultrasound-triggered release, including nanocapsules,Citation84–Citation86 nanoemulsions,Citation87,Citation88 liposomes,Citation89,Citation90 and PMs,Citation91,Citation92 have been reported. Ultrasound waves could produce thermal (by absorption of energy) and nonthermal/mechanical effects by the cavitation, resulting in the desta-bilization of nanopreparations and resultant drug release. In addition, ultrasound can also increase the permeability of biological barriers, such as cell membrane and blood–brain barrier, through the formation of cavitation bubbles and generated heat, leading to enhanced cellular uptake of the drugs.Citation93 Ultrasound-responsive PMs have shown increased drug release upon exposure to ultrasound by the physical/chemical disruption of the micelles, representing an attractive drug delivery and release strategy. To date, the most extensively studied micelles for ultrasound-triggered drug release are based on Pluronic copolymers that are composed of a ternary copolymer of poly(ethylene oxide) (PEO) and PPO (PEO-PPO-PEO).Citation94,Citation95
Recently, Wu et al developed Pluronic P123/F127 PMs encapsulating curcumin that could be permeabilized directly by focused ultrasound, leading to ultrasound-triggered drug release.Citation96 Compared to free curcumin, curcumin-loaded P123/F127 mixed micelles showed longer circulating time and increased cellular uptake. With the assistance of the focused ultrasound, such micellar system exhibited tumor-targeted deposition in a time-dependent manner following systemic administration, which was caused by the enhanced permeabilization of tumor regions and increased penetration of PMs in irradiated tumor cells under ultrasound sonoporation. Additionally, the mixed micelles could be regulated by ultrasound irradiation. The in vivo site-specific drug release was demonstrated in dual-tumor models, which showed spatiotemporal release of the entrapped drugs following intratumoral injection, resulting in significant inhibition of the tumor growth by approximately 6.5-fold more than that of the PMs without ultrasound irradiation. In another interesting work, Liang et al synthesized a metal–supramolecular diblock copolymer (poly(propylene glycol)-PEG) with a mechano-labile bis(terpyridine)–Cu(II) complex linkage in the junction point, which could self-assemble into the spherical PMs in aqueous solution.Citation97 They found that the weak Cu(II)–terpyridine dynamic bond in the copolymer chain was able to be cleaved under the high-intensity focused ultrasound, leading to the disruption of the copolymer micelles and the release of the loaded cargo.
Light-responsive PMs
Among various trigger signals used in biomedical applications, light, including UV, visible, and infrared/NIR light, represents an attractive external stimulus owing to its noninvasive nature, the possibility of remote control, and high spatiotemporal resolution.Citation98–Citation101 The use of NIR light is of particular interest due to its deep tissue penetration (up to about 10 cm in human body) without significant tissue damage, while UV light cannot penetrate deeply into the body as a result of absorption by the skin, blood, and tissues.Citation102 In addition, light-induced reactions can be regulated for various applications by adjusting the light parameters like wavelength, intensity, and exposure time. Because of these advantages, a large number of light-responsive NDDSs have been developed to achieve on-demand drug release for cancer treatment. Among them, PMs and liposomes are the most commonly studied ones. The light-responsive PMs are typically obtained by incorporation and/or conjugation of chromophores, such as azobenzene, pyrene, cinnamoyl, spirobenzopyran, or nitrobenzyl groups, in/to the polymeric structure.Citation103–Citation105 Upon illumination, the PMs’ nanostructure changes and the micelles dissociate, thus releasing the payloads. Due to the ability of reversible UV–visible pho-toisomerization, azobenzene and its derivatives are one of the most widely studied light-responsive chromophores, which can undergo a trans-to-cis photoisomerization of nitrogen double bond upon the UV irradiation, while this conversion can be reconverted to the trans isomer under the visible light. However, some other chromophores like 2-diazo-1,2-napthoquinone (DNQ) undergo light-responsive irreversible cleavage, converting hydrophobic molecules into hydrophilic ones.Citation106 Accordingly, light-responsive PMs are categorized into two types based on the above mechanisms of destabilization, including reversible structural change and irreversible photo-cleavage.
Poelma et al presented one-photon visible light-responsive PMs using the reversible structural change strategy for efficient, on-demand delivery of small molecules.Citation107 They developed the donor–acceptor Stenhouse adducts (DASAs) which could undergo a hydrophobic-to-hydrophilic polarity change triggered by visible light between 530 and 570 nm.Citation108 Compared to two-photon processes, molecules with one-photon absorption showed more desirable wavelengths and were more efficient, with the photoisomerization of DASA systems being triggered by low intensities of light (∼1 mW cm−2). In this study, the DASA building blocks were used as photochromic units in the construction of PMs, allowing for the light-triggered release of small molecules to cells by the structural disassembly of micelles using one-photon visible light. Spiropyran (SP), a well-known photochromic molecule, was exploited by Son et al to initiate the ring-opening multibranching polymerization of glycidol to afford a series of hyper-branched polyglycerol (SP-hb-PG) micelles.Citation109 The micelle assembly and disassembly could be induced by an external light source owing to the reversible photoisomerization of hydrophobic SP to hydrophilic merocyanine corresponding to the color change from colorless to pink. The assembly and disassembly of SP-hb-PG micelles were confirmed by a series of experiments, involving transmission electron microscopy (TEM), atomic force microscopy, UV–visible spectroscopy, and dynamic light scattering (DLS).
In addition to the reversible photoisomerization, the light-induced cleavage of chemical bonds has also been widely used in light-responsive PMs. Saravanakumar et al reported a biocompatible amphiphilic block copolymer (1O2-PEG-b-PCL) formed PMs bearing a singlet oxygen (1O2)-sensitive vinyldithioether cleavable linker at the core–shell junction, which could undergo 1O2-mediated photocleavage in the presence of visible light, leading to light-responsive release of 1O2 and anticancer drugs for enhanced photodynamic therapy ().Citation110 The double bond of the vinyldithioether linker can readily react with 1O2 to form a dioxetane intermediate, which is unstable and spontaneously cleaved. Unlike other conventional photo-responsive micelles that demand direct absorption of UV light, the prepared PMs utilized the 1O2 generated by visible light during photodynamic therapy as a trigger to disassemble and release a co-loaded drug in the tumor cell for effective therapy. In another study, Sun et al reported the preparation of an amphiphilic dendritic polymer of PEO-D3DNQ for both UV- and NIR-triggered drug release.Citation111 In this work, PEO-D3DNQ was prepared by click conjugation between DNQ-decorated poly(amido amine) dendron D3 (generation 3) and azide-terminated PEO (PEO-N3, molecular weights of 2 and 5 kDa). As character-ized by time-resolved UV–visible spectroscopy, DLS, and TEM, the obtained micelles exhibited both UV and NIR sensitivity in phosphate-buffered saline (PBS). It was found that the characteristic absorption intensity of DNQ progressively decreased and then leveled off within 8 minutes under UV365 nm irradiation, suggesting the completion of the Wolff rearrangement of DNQ, while under 808 nm NIR irradiation, it took a longer time of 40–60 minutes to complete the Wolff rearrangement of DNQ. After 30 minutes of 808 nm irradiation, most of the micelles could be disrupted and the apparent drug-release rate showed a nearly eightfold increase, presenting an NIR-triggered drug-release profile.
Figure 8 Schematic illustration of (A) the formation of Ce6 and DOX co-loaded micelles (1O2-PM-Ce6-DOX), (B) cellular uptake and visible light-triggered 1O2-mediated intracellular co-delivery of Ce6 and DOX for combination chemo- and photodynamic therapy, and (C) chemical structure of the 1O2-PEG-b-PCL copolymer.
Notes: Reprinted from Saravanakumar G, Lee J, Kim J, Kim WJ. Visible light-induced singlet oxygen-mediated intracellular disassembly of polymeric micelles co-loaded with a photosensitizer and an anticancer drug for enhanced photodynamic therapy. Chem Commun (Camb). 2015;51(49):9995–9998.Citation110 Creative Commons Attribution 3.0 Unported License. Published by the Royal Society of Chemistry.
Abbreviations: Ce6, chlorin e6; DOX, doxorubicin; PM, polymeric micelle; PEG, poly(ethylene glycol); PCL, poly(ε-caprolactone).
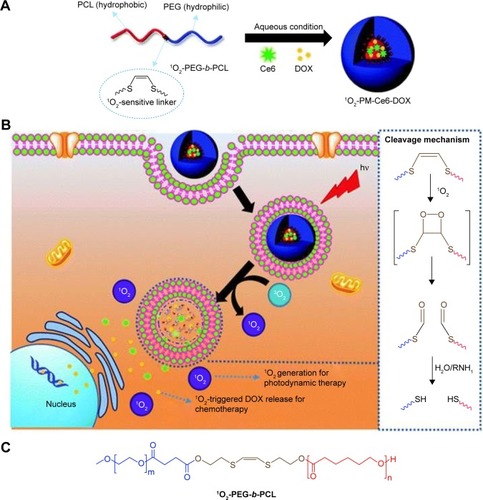
Multi-responsive PMs
Multi-stimuli-responsive PMs that are sensitive to two or more stimuli are emerging and have shown great potential for cancer-targeted drug delivery and therapy. By combining multiple stimuli sensitivities into one PM system, a precisely controlled drug delivery and release can be achieved, resulting in superior in vitro and/or in vivo anticancer activity. The combined sensitivities to different stimuli can take place either simultaneously or in a sequential way.
Dual pH/redox-responsive PMs
Dual pH/redox-responsive PMs have been developed, as both stimuli are naturally present in the cancer microenvironment. Recently, Alex et al prepared a dual redox/pH-responsive PM for co-delivery of DOX and polo-like kinase 1 siRNA.Citation112 Cationic structures including histidine and arginine were first grafted to the poly(styrene-altmaleic anhydride) through dis-ulfide linkages, and then short PEG chains were incorporated to impart stealth nature and self-assembly ability. Besides, in order to make them stabile in physiological conditions, drug-loaded PMs were noncovalently coated with BSA. The dual-stimuli-sensitive nature of the PMs significantly increased their drug release at low pH and 10 mM GSH which mimicked the endosomal pH and GSH-rich microen-vironment of cancer cells. Both in vitro and in vivo results showed synergistic effects of DOX and siRNA in inhibiting tumor cell proliferation.
Dual pH/thermo-responsive PMs
Among various dual-stimuli-responsive PMs developed for cancer therapy, pH and temperature are the most commonly studied combination because of the tumor’s acidity and relatively higher local temperature or easily applied external heat sources. Hong et al conjugated PHis to poly(lactic-co-glycolic acid) (PLGA)-PEG-PLGA to form PHis-PLGA-PEG-PLGA-PHis copolymers, by which a thermo- and pH-responsive PM was developed.Citation113 The temperature- and pH-induced structural changes of the PMs were characterized by alteration in particle size, pyrene fluorescence intensity, and 1H NMR spectra in D2O. Additionally, the in vitro drug release, cyto-toxicity, and intracellular localization further confirmed the temperature- and pH-responsive properties of the copolymer micelles. In another study, Chen et al prepared an optimized, biodegradable, dual temperature/pH-responsive PM system for enhanced tumor accumulation, in which the hydrophobic segment of dual-responsive copolymer was composed of poly(N-(2-hydroxypropyl) methacrylamide dilactate)-co-(N-(2-hydroxypropyl) methacrylamide-co-histidine).Citation114 The poly(N-(2-hydroxypropyl) methacrylamide dilactate) had a thermo-reversible LCST suitable for the targeted delivery of a variety of cargos, and histidine was pH sensitive and widely used in polymeric drug delivery systems.
Dual redox/magnetic-responsive PMs
The redox-responsive magnetic nanoparticles such as SPIONs have been developed to combine redox-responsive drug release with magnetic targeting and imaging. Recently, Tang et al developed redox-responsive star-shaped magnetic micelles with active-targeted and magnetic-guided functions for cancer therapy.Citation115 The magnetic star-shaped micelles were formed by self-assembly of four-arm PEG-PCL copolymers with disulfide bonds as intermediate linkers. DOX and magnetic iron oxide nanoparticles (Fe3O4) were simultaneously encapsulated into the hydrophobic cores of the micelles. The active targeting was rendered by the conjugation of phenyl-boronic acid ligands that showed high affinity and selectivity to the tumor-overexpressed antigen salic acid. The resultant magnetic PMs enhanced the antitumor efficacy under magnetic field but decreased the adverse effects.
Dual redox/enzyme-responsive PMs
In a recent study, Zhang et al developed dual enzyme/redox-responsive PMs with active targeting abilities to achieve rapid intracellular drug release.Citation116 In order to overcome the drugs’ poor solubility and low stability, camp-tothecin (CPT) was chemically conjugated to mPEG via a redox-responsive linker to form polymeric prodrugs. The enzyme-responsive behavior was achieved by introduction of the azo bonds as the linker between the hydrophobic poly-caprolactone and hydrophilic PEG segments. The azo bonds can be selectively cleaved by oxidation–reduction enzyme (azoreductase) in the presence of the coenzyme-reduced nicotinamide adenine dinucleotide phosphate. In addition, PBA was conjugated to the end of PEG to realize active tumor targeting and cellular uptake via receptor-mediated endocytosis. In the cytoplasm, CPT could be rapidly released through the dual triggers of enzyme and redox responsiveness and effectively inhibited the tumor proliferation and decreased the side effects.
Triple pH/thermo/redox-responsive PMs
In addition to dual-responsive PMs, triple-responsive systems are also attracting increasing interest. Huang et al reported novel triple thermo/pH/reduction-sensitive PMs based on a block copolymer p(poly(ethylene glycol) methyl meth-acrylate-co-(tert-butyloxycarbonylaminoethyldithioethyl methacrylamide)-co-vinylimidazole) (p(PEG-MEMA-co-Boc-Cyst-MMAm-co-VI))-b-PEG (PPBV) for intracellular drug delivery.Citation117 The pH/temperature-responsive behavior of such PMs was observed by both DLS and UV–vis absorbance. When the solution was heated above 21°C, the size of PPBV in PBS (pH 7.4) increased rapidly from 25 to 56 nm. Besides, the size–temperature curves for PPBV in aqueous solution moved toward higher temperatures with decreasing pH from 7.4 to 6.0. The PTX-loaded micelles showed slow drug release in PBS but rapid release after exposure to a weakly acidic pH or reductive environment, indicating the pH- and reduction-sensitive behavior of PPBV. The obtained PMs showed higher cytotoxicity against HepG2 cells due to the increased intracellular PTX concentration, which therefore may serve as promising carriers for cytostatic drugs.
Conclusion
A large number of preclinical studies on multifunctional PMs have been published, which showed that PMs-based drug delivery systems are promising to be an effective nanomedicine platform for drug delivery and cancer therapy, resulting in enhanced therapeutic index and reduced systemic side effects compared to traditional anticancer drugs. PMs are one of the nanocarriers that can selectively target drug to the tumor though the EPR effect. The small size along with the suitable surface properties such as the hydrophilic PEG corona could prevent the PMs from rapid clearance from the body, allowing for prolonged circulation time and efficient tumor accumulation. Additionally, as most of the anticancer drugs are poorly water-soluble, PMs may be a perfect way for drug solubilization as well as delivery. Moreover, PMs can be further modified with tumor targeting ligands to increase the drug’s tumor selectivity and enhance intracellular drug delivery. To date, about nine drug-loaded PMs have been investigated in clinical trials, of which Genexol-PM was approved in 2007 in Korea and marketed in Europe for treating breast cancer and small-cell lung cancer. However, the clinical translation and desired therapeutic effects of PMs are still far from satisfactory. The drug-loaded PMs currently investigated in the clinical trials mostly rely on the passive tumor targeting via the EPR effect; however, their targeting effect in human sometimes is not as pronounced as that obtained in the simple cell and animal models due to the complexity of the tumor and human body. Besides, the premature drug release in the bloodstream and inadequate drug release in the tumor may also account for the inferior therapeutic effects in human patients. Thus, in order to ensure the success of PMs, further modification of micelles is required regarding the drug loading and release, blood circulation, tumor targeting, cellular uptake, and intracellular drug release.
Numerous stimuli-responsive PMs have been developed to deliver anticancer drug in response to a variety of extra/intracellular stimuli or external triggers, including pH, redox potential, enzymes, temperature, light, ultrasound, and magnetic field, which showed the improved performance in addressing the above-mentioned concerns. Despite the impressive process has been achieved in the design and application of stimuli-responsive PMs for cancer therapy, many challenges and crucial issues still remain to be addressed. First, it is crucial to understand how PMs interact with the biological components. Upon administration, PMs encounter a variety of biological molecules, cells, and tissues/organs. The surface of PMs would be immediately covered with biological molecules such as serum proteins which play an important role in determining the subsequent biodistribution and cellular responses of PMs. Second, off-target effects or insufficient stimuli sensitivity may happen due to the nonspecific distribution of the stimuli, especially internal stimuli. For example, low pH, high concentration of GSH, or upregulation of certain enzymes can also be found in some normal cells or nontumoral tissues. Besides, the heterogeneity of the patients and tumors and the tumor type and stage greatly influence the status of the internal stimuli. Thus, the stimuli sensitivity of PMs should be well studied. Third, the complicated design and difficulties in the scale-up of stimuli-responsive PMs are likely to hamper their clinical translation, which is one major reason why a number of stimuli-responsive PMs have been reported, whereas only a few of them could enter the clinical stage.
Summing up, we reviewed the recent progress of stimuli-responsive PMs for cancer therapy and highlighted the representative examples regarding the stimuli-responsive temporal and spatial drug delivery and release. The potential and progress of stimuli-responsive PMs have been clearly shown in previous studies. In the near future, we believe that the stimuli-responsive PMs would become an important component of clinical cancer therapy.
Acknowledgments
The authors would like to acknowledge the support from the National Natural Science Foundation of China (grant numbers 81571786, 31771087, 81271687, 31671015) and Shaanxi Science & Technology Co-ordination & Innovation Project (2015KTCL03-12). Qing Zhou is grateful to the China Scholarship Council (CSC) for the financial support (CSC number 201603170199).
Disclosure
The authors report no conflicts of interest in this work.
References
- AlyHACancer therapy and vaccinationJ Immunol Methods20123821–212322658969
- LorussoDBriaECostantiniADi MaioMRostiGMancusoAPatients’ perception of chemotherapy side effects: expectations, doctor–patient communication and impact on quality of life – an Italian surveyEur J Cancer Care (Engl)2017262e12618
- DemariaMO’LearyMNChangJCellular senescence promotes adverse effects of chemotherapy and cancer relapseCancer Discov20177216517627979832
- ShiJKantoffPWWoosterRFarokhzadOCCancer nanomedicine: progress, challenges and opportunitiesNat Rev Cancer2017171203727834398
- von RoemelingCJiangWChanCKWeissmanILKimBYSBreaking down the barriers to precision cancer nanomedicineTrends Biotechnol201735215917127492049
- ZhouQZhangLWuHNanomaterials for cancer therapiesNanotechnol Rev201765473496
- GuptaADA review on recent advancement of cancer therapy using nanoparticlesBiochem Mol Biol Lett201731104
- Yousefpour MarzbaliMYari KhosroushahiAPolymeric micelles as mighty nanocarriers for cancer gene therapy: a reviewCancer Chemother Pharmacol201794637649
- ChoHLaiTCTomodaKKwonGSPolymeric micelles for multi-drug delivery in cancerAAPS PharmSciTech2015161102025501872
- Varela-MoreiraAShiYFensMHLammersTHenninkWESchiffelersRMClinical application of polymeric micelles for the treatment of cancerMater Chem Front2017114851501
- CabralHKataokaKProgress of drug-loaded polymeric micelles into clinical studiesJ Control Release201419046547624993430
- LiYXiaoKZhuWDengWLamKSStimuli-responsive cross-linked micelles for on-demand drug delivery against cancersAdv Drug Deliv Rev201466587324060922
- NakayamaMAkimotoJOkanoTPolymeric micelles with stimuli-triggering systems for advanced cancer drug targetingJ Drug Target201422758459925012066
- JonesMLerouxJPolymeric micelles – a new generation of colloidal drug carriersEur J Pharm Biopharm199948210111110469928
- SuttonDNasongklaNBlancoEGaoJFunctionalized micellar systems for cancer targeted drug deliveryPharm Res20072461029104617385025
- TorchilinVPStructure and design of polymeric surfactant-based drug delivery systemsJ Control Release2001732–313717211516494
- TorchilinVPMicellar nanocarriers: pharmaceutical perspectivesPharm Res200724111617109211
- GrosLRingsdorfHSchuppHPolymeric antitumor agents on a molecular and on a cellular level?Angew Chem Int Ed Engl1981204305325
- MatsumuraYPoly (amino acid) micelle nanocarriers in preclinical and clinical studiesAdv Drug Deliv Rev200860889991418406004
- PeerDKarpJMHongSFarokhzadOCMargalitRLangerRNano-carriers as an emerging platform for cancer therapyNat Nanotechnol200721275176018654426
- GreishKEnhanced permeability and retention (EPR) effect for anticancer nanomedicine drug targetingCancer Nanotechnology: Methods and Protocols20102537
- CabralHMatsumotoYMizunoKAccumulation of sub-100 nm polymeric micelles in poorly permeable tumours depends on sizeNat Nanotechnol201161281582322020122
- HuangKMaHLiuJSize-dependent localization and penetration of ultrasmall gold nanoparticles in cancer cells, multicellular spheroids, and tumors in vivoACS Nano2012654483449322540892
- MaXWuYJinSGold nanoparticles induce autophagosome accumulation through size-dependent nanoparticle uptake and lysosome impairmentACS Nano20115118629863921974862
- MahmudAXiongXBAliabadiHMLavasanifarAPolymeric micelles for drug targetingJ Drug Target200715955358417968711
- MillerTBreyerSvan ColenGPremature drug release of polymeric micelles and its effects on tumor targetingInt J Pharm20134451–211712423384729
- BaeYFukushimaSHaradaAKataokaKDesign of environment-sensitive supramolecular assemblies for intracellular drug delivery: polymeric micelles that are responsive to intracellular pH changeAngew Chem Int Ed Engl200342384640464314533151
- KoJParkKKimYSTumoral acidic extracellular pH targeting of pH-responsive MPEG-poly (beta-amino ester) block copolymer micelles for cancer therapyJ Control Release2007123210911517894942
- MinKHKimJHBaeSMTumoral acidic pH-responsive MPEG-poly (beta-amino ester) polymeric micelles for cancer targeting therapyJ Control Release2010144225926620188131
- KooHLeeHLeeSIn vivo tumor diagnosis and photodynamic therapy via tumoral pH-responsive polymeric micellesChem Commun (Camb)201046315668567020623050
- KanamalaMWilsonWRYangMPalmerBDWuZMechanisms and biomaterials in pH-responsive tumour targeted drug delivery: a reviewBiomaterials20168515216726871891
- WuHZhuLTorchilinVPpH-sensitive poly (histidine)-PEG/DSPE-PEG co-polymer micelles for cytosolic drug deliveryBiomaterials20133441213122223102622
- HuJMiuraSNaKBaeYHpH-responsive and charge shielded cationic micelle of poly (L-histidine)-block-short branched PEI for acidic cancer treatmentJ Control Release20131721697623954370
- YangTLiFZhangHMultifunctional pH-sensitive micelles for tumor-specific uptake and cellular deliveryPolym Chem20156813731382
- ZhouQHouYZhangLDual-pH sensitive charge-reversal nanocomplex for tumor-targeted drug delivery with enhanced anticancer activityTheranostics2017771806181928638469
- ZhouZLiLYangYXuXHuangYTumor targeting by pH-sensitive, biodegradable, cross-linked N-(2-hydroxypropyl) methacrylamide copolymer micellesBiomaterials201435246622663524814427
- MintzerMASimanekEENonviral vectors for gene deliveryChem Rev20081092259302
- AndersonDGLynnDMLangerRSemi-automated synthesis and screening of a large library of degradable cationic polymers for gene deliveryAngew Chem20031152732613266
- LeeHJPardridgeWMMonoclonal antibody radiopharmaceuticals: cationization, pegylation, radiometal chelation, pharmacokinetics, and tumor imagingBioconjug Chem200314354655312757378
- MaSFNishikawaMKatsumiHYamashitaFHashidaMCationic charge-dependent hepatic delivery of amidated serum albuminJ Control Release2005102358359415681081
- BalendiranGKDaburRFraserDThe role of glutathione in cancerCell Biochem Funct200422634335215386533
- SchaferFQBuettnerGRRedox environment of the cell as viewed through the redox state of the glutathione disulfide/glutathione coupleFree Radic Biol Med200130111191121211368918
- KuppusamyPLiHIlangovanGNoninvasive imaging of tumor redox status and its modification by tissue glutathione levelsCancer Res200262130731211782393
- SunYYanXYuanTDisassemblable micelles based on reduction-degradable amphiphilic graft copolymers for intracellular delivery of doxorubicinBiomaterials201031277124713120580429
- LiJHuoMWangJRedox-sensitive micelles self-assembled from amphiphilic hyaluronic acid-deoxycholic acid conjugates for targeted intracellular delivery of paclitaxelBiomaterials20123372310232022166223
- WangYCWangFSunTMWangJRedox-responsive nanoparticles from the single disulfide bond-bridged block copolymer as drug carriers for overcoming multidrug resistance in cancer cellsBioconjug Chem201122101939194521866903
- ShiCGuoXQuQTangZWangYZhouSActively targeted delivery of anticancer drug to tumor cells by redox-responsive star-shaped micellesBiomaterials201435308711872225002267
- WangJYangGGuoXTangZZhongZZhouSRedox-responsive polyanhydride micelles for cancer therapyBiomaterials20143593080309024388799
- HuJZhangGLiuSEnzyme-responsive polymeric assemblies, nanoparticles and hydrogelsChem Soc Rev201241185933594922695880
- EgebladMWerbZNew functions for the matrix metalloproteinases in cancer progressionNat Rev Cancer20022316117411990853
- KessenbrockKPlaksVWerbZMatrix metalloproteinases: regulators of the tumor microenvironmentCell20101411526720371345
- ParkJBLeeCSJangJHPhospholipase signalling networks in cancerNat Rev Cancer2012121178279223076158
- HuQKattiPSGuZEnzyme-responsive nanomaterials for controlled drug deliveryNanoscale2014621122731228625251024
- ChoiKYYoonHYKimJHSmart nanocarrier based on PEGylated hyaluronic acid for cancer therapyACS Nano20115118591859921967065
- JiangTMoRBellottiAZhouJGuZGel–liposome-mediated co-delivery of anticancer membrane-associated proteins and small-molecule drugs for enhanced therapeutic efficacyAdv Funct Mater2014241622952304
- XuJHGaoFPLiLLGelatin–mesoporous silica nanoparticles as matrix metalloproteinases-degradable drug delivery systems in vivoMicroporous Mesoporous Mater2013182165172
- ZhuLWangTPercheFTaigindATorchilinVPEnhanced anticancer activity of nanopreparation containing an MMP2-sensitive PEG-drug conjugate and cell-penetrating moietyProc Natl Acad Sci U S A201311042170471705224062440
- ZhuLPercheFWangTTorchilinVPMatrix metalloproteinase 2-sensitive multifunctional polymeric micelles for tumor-specific co-delivery of siRNA and hydrophobic drugsBiomaterials201435134213422224529391
- ColottaFAllavenaPSicaAGarlandaCMantovaniACancer-related inflammation, the seventh hallmark of cancer: links to genetic instabilityCarcinogenesis20093071073108119468060
- GromkowskiSHYagiJJanewayCAJrElevated temperature regulates tumor necrosis factor-mediated immune killingEur J Immunol1989199170917142792186
- ChenSLiYGuoCTemperature-responsive magnetite/PEO−PPO−PEO block copolymer nanoparticles for controlled drug targeting deliveryLangmuir20072325126691267617988160
- KoppoluBBhavsarZWadajkarASTemperature-sensitive polymer-coated magnetic nanoparticles as a potential drug delivery system for targeted therapy of thyroid cancerJ Biomed Nanotechnol20128698399023030006
- PurushothamSChangPRumpelHThermoresponsive core–shell magnetic nanoparticles for combined modalities of cancer therapyNanotechnology2009203030510119581698
- SunJTYuZQHongCYPanCYBiocompatible zwitterionic sulfobetaine copolymer-coated mesoporous silica nanoparticles for temperature-responsive drug releaseMacromol Rapid Commun201233981181822488562
- KanazawaHYamamotoKMatsushimaYTemperature-responsive chromatography using poly (N-isopropylacrylamide)-modified silicaAnal Chem199668110010521619225
- RonESBrombergLETemperature-responsive gels and thermogelling polymer matrices for protein and peptide deliveryAdv Drug Deliv Rev199831319722110837626
- NakayamaMOkanoTMiyazakiTKohoriFSakaiKYokoyamaMMolecular design of biodegradable polymeric micelles for temperature-responsive drug releaseJ Control Release20061151465616920217
- WeiHZhangXChengCChengSXZhuoRXSelf-assembled, thermosensitive micelles of a star block copolymer based on PMMA and PNIPAAm for controlled drug deliveryBiomaterials20072819910716959312
- ZhangXZWuDQChuCCSynthesis, characterization and controlled drug release of thermosensitive IPN–PNIPAAm hydrogelsBiomaterials200425173793380515020155
- ZhangXWuDChuCCSynthesis and characterization of partially biodegradable, temperature and pH sensitive Dex–MA/PNIPAAm hydrogelsBiomaterials200425194719473015120518
- SchmaljohannDOswaldJJørgensenBNitschkeMBeyerleinDWernerCThermo-responsive PNiPAAm-g-PEG films for controlled cell detachmentBiomacromolecules2003461733173914606903
- PanjaSDeyGBhartiRTailor-made temperature-sensitive micelle for targeted and on-demand release of anticancer drugsACS Appl Mater Interfaces2016819120631207427128684
- AhnDGLeeJParkSYKwarkYJLeeKYDoxorubicin-loaded alginate-g-poly (N-isopropylacrylamide) micelles for cancer imaging and therapyACS Appl Mater Interfaces2014624220692207725487046
- SawantRMSawantRRGultepeENanosized cancer cell-targeted polymeric immunomicelles loaded with superparamagnetic iron oxide nanoparticlesJ Nanopart Res20091171777
- LiaoCSunQLiangBShenJShuaiXTargeting EGFR-overexpressing tumor cells using Cetuximab-immunomicelles loaded with doxorubicin and superparamagnetic iron oxideEur J Radiol201180369970520810233
- GuptaAKNaregalkarRRVaidyaVDGuptaMRecent advances on surface engineering of magnetic iron oxide nanoparticles and their biomedical applicationsNanomedicine (Lond)200721233917716188
- GuptaAKGuptaMSynthesis and surface engineering of iron oxide nanoparticles for biomedical applicationsBiomaterials200526183995402115626447
- ShinkaiMFunctional magnetic particles for medical applicationJ Biosci Bioeng200294660661316233357
- BoyerCWhittakerMRBulmusVLiuJDavisTPThe design and utility of polymer-stabilized iron-oxide nanoparticles for nanomedicine applicationsNPG Asia Mater2010212330
- WeiJShuaiXWangRClickable and imageable multiblock polymer micelles with magnetically guided and PEG-switched targeting and release property for precise tumor theranosisBiomaterials201714513815328863308
- LiHYanKShangYFolate-bovine serum albumin functionalized polymeric micelles loaded with superparamagnetic iron oxide nanoparticles for tumor targeting and magnetic resonance imagingActa Biomater20151511712625595473
- LeeSYYangCYPengCLA theranostic micelleplex co-delivering SN-38 and VEGF siRNA for colorectal cancer therapyBiomaterials2016869210526896610
- MitragotriSHealing sound: the use of ultrasound in drug delivery and other therapeutic applicationsNat Rev Drug Discov20054325526015738980
- ChenYChenHSunYMultifunctional mesoporous composite nanocapsules for highly efficient MRI-guided high-intensity focused ultrasound cancer surgeryAngew Chem Int Ed Engl20115052125051250922076783
- WangXChenHChenYPerfluorohexane-encapsulated mesoporous silica nanocapsules as enhancement agents for highly efficient High Intensity Focused Ultrasound (HIFU)Adv Mater201224678579122223403
- YangPLiDJinSStimuli-responsive biodegradable poly (meth-acrylic acid) based nanocapsules for ultrasound traced and triggered drug delivery systemBiomaterials20143562079208824331704
- RapoportNYKennedyAMSheaJEScaifeCLNamKHControlled and targeted tumor chemotherapy by ultrasound-activated nanoemulsions/microbubblesJ Control Release2009138326827619477208
- RapoportNNamKHGuptaRUltrasound-mediated tumor imaging and nanotherapy using drug loaded, block copolymer stabilized per-fluorocarbon nanoemulsionsJ Control Release2011153141521277919
- HuangSLMacDonaldRCAcoustically active liposomes for drug encapsulation and ultrasound-triggered releaseBiochim Biophys Acta200416651–213414115471579
- SchroederAHonenRTurjemanKGabizonAKostJBarenholzYUltrasound triggered release of cisplatin from liposomes in murine tumorsJ Control Release20091371636819303426
- ZhangHXiaHWangJLiYHigh intensity focused ultrasound-responsive release behavior of PLA-b-PEG copolymer micellesJ Control Release20091391313919523500
- MohanPRapoportNDoxorubicin as a molecular nanotheranostic agent: effect of doxorubicin encapsulation in micelles or nanoemulsions on the ultrasound-mediated intracellular delivery and nuclear traffickingMol Pharm2010761959197320957997
- UngerECMcCreeryTPSweitzerRHUltrasound enhances gene expression of liposomal transfectionInvest Radiol199732127237279406011
- MarinASunHHusseiniGAPittWGChristensenDARapoportNYDrug delivery in pluronic micelles: effect of high-frequency ultrasound on drug release from micelles and intracellular uptakeJ Control Release2002841–2394712399166
- HusseiniGAChristensenDARapoportNYPittWGUltrasonic release of doxorubicin from Pluronic P105 micelles stabilized with an interpenetrating network of N, N-diethylacrylamideJ Control Release200283230330512363455
- WuPJiaYQuFUltrasound-responsive polymeric micelles for sonoporation-assisted site-specific therapeutic actionACS Appl Mater Interfaces2017931257062571628741924
- LiangBTongRWangZGuoSXiaHHigh intensity focused ultrasound responsive metallo-supramolecular block copolymer micellesLangmuir201430319524953225072274
- ShanmugamVSelvakumarSYehCSNear-infrared light-responsive nanomaterials in cancer therapeuticsChem Soc Rev201443176254628724811160
- AngelatosASRadtBCarusoFLight-responsive polyelectrolyte/gold nanoparticle microcapsulesJ Phys Chem B200510973071307616851322
- YangXLiuXLiuZPuFRenJQuXNear-infrared light-triggered, targeted drug delivery to cancer cells by aptamer gated nanovehiclesAdv Mater201224212890289522539076
- ZhangZWangJChenCNear-infrared light-mediated nanoplatforms for cancer thermo-chemotherapy and optical imagingAdv Mater201325283869388024048973
- JochumFDTheatoPTemperature- and light-responsive smart polymer materialsChem Soc Rev201342177468748322868906
- ZhaoYRational design of light-controllable polymer micellesChem Rec20077528629417924441
- SchumersJMFustinCAGohyJFLight-responsive block copolymersMacromol Rapid Commun201031181588160721567570
- ZhaoYLStoddartJFAzobenzene-based light-responsive hydrogel systemLangmuir200925158442844620050041
- CuiJDel CampoAPhoto-responsive polymers: properties, synthesis and applicationsde ArmasMRAAguilarMRRománJSSmart Polymers and Their ApplicationsCambridgeElsevier201493133
- PoelmaSOOhSSHelmySControlled drug release to cancer cells from modular one-photon visible light-responsive micellar systemChem Commun201652691052510528
- HelmySLeibfarthFAOhSPoelmaJEHawkerCJRead de AlanizJPhotoswitching using visible light: a new class of organic photochro-mic moleculesJ Am Chem Soc2014136238169817224848124
- SonSShinEKimBSLight-responsive micelles of spiropyran initiated hyperbranched polyglycerol for smart drug deliveryBiomacromolecules201415262863424432713
- SaravanakumarGLeeJKimJKimWJVisible light-induced singlet oxygen-mediated intracellular disassembly of polymeric micelles co-loaded with a photosensitizer and an anticancer drug for enhanced photodynamic therapyChem Commun (Camb)201551499995999825998105
- SunLZhuBSuYDongCMLight-responsive linear-dendritic amphiphiles and their nanomedicines for NIR-triggered drug releasePolym Chem20145516051613
- AlexMANehateCVeeranarayananSKumarDSKulshreshthaRKoulVSelf assembled dual responsive micelles stabilized with protein for co-delivery of drug and siRNA in cancer therapyBiomaterials20171339410628433941
- HongWChenDJiaLThermo- and pH-responsive copoly-mers based on PLGA-PEG-PLGA and poly (L-histidine): synthesis and in vitro characterization of copolymer micellesActa Biomater20141031259127124365708
- ChenYCLiaoLCLuPLThe accumulation of dual pH and temperature responsive micelles in tumorsBiomaterials201233184576458822445255
- TangZZhangLWangYLiDZhongZZhouSRedox-responsive star-shaped magnetic micelles with active-targeted and magnetic-guided functions for cancer therapyActa Biomater20164223224627373437
- ZhangLWangYZhangXWeiXXiongXZhouSEnzyme and redox dual-triggered intracellular release from actively targeted polymeric micellesACS Appl Mater Interfaces2017943388339928071889
- HuangXJiangXYangQTriple-stimuli (pH/thermo/reduction) sensitive copolymers for intracellular drug deliveryJ Mater Chem B201311318601868
- ValleJWArmstrongANewmanCA phase 2 study of SP1049C, doxorubicin in P-glycoprotein-targeting pluronics, in patients with advanced adenocarcinoma of the esophagus and gas-troesophageal junctionInvest New Drugs20112951029103720179989
- DansonSFerryDAlakhovVPhase I dose escalation and pharmacokinetic study of pluronic polymer-bound doxorubicin (SP1049C) in patients with advanced cancerBr J Cancer200490112085209115150584
- ArmstrongABrewerJNewmanCSP1049C as first-line therapy in advanced (inoperable or metastatic) adenocarcinoma of the oesophagus: a phase II window studyJ Clin Oncol20062418 Suppl4080
- MatsumuraYKataokaKPreclinical and clinical studies of anticancer agent-incorporating polymer micellesCancer Sci2009100457257919462526
- NakanishiTFukushimaSOkamotoKDevelopment of the polymer micelle carrier system for doxorubicinJ Control Release2001741–329530211489509
- KimKSParkWHuJBaeYHNaKA cancer-recognizable MRI contrast agents using pH-responsive polymeric micelleBiomaterials201435133734324139764
- YuPYuHGuoCReversal of doxorubicin resistance in breast cancer by mitochondria-targeted pH-responsive micellesActa Biomater20151411512425498306
- YuJDengHXieFChenWZhuBXuQThe potential of pH-responsive PEG-hyperbranched polyacylhydrazone micelles for cancer therapyBiomaterials20143593132314424439411
- TanYZhuYZhaoYMitochondrial alkaline pH-responsive drug release mediated by Celastrol loaded glycolipid-like micelles for cancer therapyBiomaterials201815416918129128845
- WenHLiYRedox sensitive nanoparticles with disulfide bond linked sheddable shell for intracellular drug deliveryMed Chem2014411748755
- YinTWangLYinLZhouJHuoMCo-delivery of hydrophobic paclitaxel and hydrophilic AURKA specific siRNA by redox-sensitive micelles for effective treatment of breast cancerBiomaterials201561102525996409
- MaYCWangJXTaoWRedox-responsive polyphosphoester-based micellar nanomedicines for overriding chemoresistance in breast cancer cellsACS Appl Mater Interfaces2015747263152632526552849
- ChenWHLuoGFLeiQMMP-2 responsive polymeric micelles for cancer-targeted intracellular drug deliveryChem Commun (Camb)201551346546825327260
- KalafatovicDNobisMJavidNMMP-9 triggered micelle-to-fibre transitions for slow release of doxorubicinBiomater Sci20153224624926218115
- SahuABoraUKasojuNGoswamiPSynthesis of novel biodegradable and self-assembling methoxy poly(ethylene glycol)–palmitate nanocarrier for curcumin delivery to cancer cellsActa Biomater2008461752176118524701
- ChengCWeiHShiBXBiotinylated thermoresponsive micelle self-assembled from double-hydrophilic block copolymer for drug delivery and tumor targetBiomaterials200829449750517959241
- EmamzadehMDesmaëleDCouvreurPPasparakisGDual controlled delivery of squalenoyl-gemcitabine and paclitaxel using thermo-responsive polymeric micelles for pancreatic cancerJ Control Release2017259e90e91
- HuYDarcosVMongeSLiSZhouYSuFThermo-responsive release of curcumin from micelles prepared by self-assembly of amphiphilic P(NIPAAm-co-DMAAm)-b-PLLA-b-P(NIPAAm-co-DMAAm) triblock copolymersInt J Pharm20144761–2314025260217
- HuYDarcosVMongeSLiSThermo-responsive drug release from self-assembled micelles of brush-like PLA/PEG analogues block copolymersInt J Pharm20154911–215216126095914
- HuangCTangZZhouYMagnetic micelles as a potential platform for dual targeted drug delivery in cancer therapyInt J Pharm20124291–211312222406331
- YangXChenYYuanRFolate-encoded and Fe3O4-loaded polymeric micelles for dual targeting of cancer cellsPolymer2008491634773485
- GloverALBennettJBPritchettJSMagnetic heating of iron oxide nanoparticles and magnetic micelles for cancer therapyIEEE Trans Magn201349123123523750047
- ChenCJLiuGYShiYTBiocompatible micelles based on comb-like PEG derivates: formation, characterization, and photo- responsivenessMacromol Rapid Commun201132141077108121674666
- JiWLiNChenDCoumarin-containing photo-responsive nanocomposites for NIR light-triggered controlled drug release via a two-photon processJ Mater Chem B201314359425949
- PruittJDPittWGSequestration and ultrasound-induced release of doxorubicin from stabilized Pluronic P105 micellesDrug Deliv20029425325812511204
- HusseiniGARapoportNYChristensenDAPruittJDPittWGKinetics of ultrasonic release of doxorubicin from pluronic P105 micellesColloids Surf B Biointerfaces2002243–4253264
- HusseiniGAAbdel-JabbarNMMjalliFSPittWGModeling and sensitivity analysis of acoustic release of doxorubicin from unstabilized pluronic P105 using an artificial neural network modelTechnol Cancer Res Treat2007614956
- ChenWZhongPMengFRedox and pH-responsive degradable micelles for dually activated intracellular anticancer drug releaseJ Control Release2013169317117923306022
- YuHCuiZYuPpH- and NIR light-responsive micelles with hyperthermia-triggered tumor penetration and cytoplasm drug release to reverse doxorubicin resistance in breast cancerAdv Funct Mater2015251724892500
- XuanJHanDXiaHZhaoYDual-stimuli-responsive micelle of an ABC triblock copolymer bearing a redox-cleavable unit and a photocleavable unit at two block junctionsLangmuir201430141041724328893
- ZouHYuanWTemperature- and redox-responsive magnetic complex micelles for controlled drug releaseJ Mater Chem B201532260269
- LeeSYLeeHInIParkSYpH/redox/photo responsive polymeric micelle via boronate ester and disulfide bonds with spiropyran-based photochromic polymer for cell imaging and anticancer drug deliveryEur Polym J201457110